DOI:
10.1039/D0RA01672A
(Paper)
RSC Adv., 2020,
10, 18390-18399
Synthesis and DSSC application of BODIPY decorated triazole bridged and benzene nucleus cored conjugated dendrimers†
Received
21st February 2020
, Accepted 28th April 2020
First published on 13th May 2020
Abstract
Conjugated dendrimers decorated with 5,5-difluoro-10-(4-(prop-2-ynyloxy)phenyl)-5H-dipyrrolo[1,2-c:1′,2′-f][1,3,2]diazaborinin-4-ium-5-uide, usually known as boron dipyrromethene (BODIPY), have been synthesized and their application as photosensitizer in dye sensitized solar cells (DSSCs) has been evaluated. Third generation triazole bridged BODIPY dendrimers show higher light energy harvesting efficiency of 2.5% better than the first and second generation dendrimers, when used as a dye material in solar cells. The current intensity increases with an increase in the generation of the dendrimer as revealed by cyclic voltammetry. Fluorescence decay analysis shows that the relaxation times τ1 and τ2 increase as the dendrimer generation increases, however τ2 for the third generation dendrimer decreases because of fluorescence quenching due to molecular crowding.
Introduction
During recent times the design and synthesis of donor–acceptor (D–A) molecular systems has attracted the attention of organic chemists. Incorporation of donor groups such as triazole at the meso position of the boron dipyrromethane (BODIPY) conjugated dendrimer endows many interesting properties. The donor–acceptor (D–A) molecular system has gained considerable attention from the scientific community due to its applications in optoelectronic devices. The BODIPY conjugated dendrimer dyes are known for their unique properties like strong absorption and emission with high quantum yield, high thermal and photochemical stability and ease of synthetic functionalization. The growing interest in BODIPY based fluorophores is due to their spectacular spectroscopic properties, such as strong absorption with high extinction coefficients, high fluorescence yields, and stabilities towards heat and light. The BODIPY unit exhibits a strong NLO response, good electrochemical properties and high thermal and photochemical stability.1 Our group and others have explored the application of the conjugated dendrimers for non-linear optical and multiphoton absorbing properties.
The BODIPY conjugated dendrimers function as strong electron acceptor, and their electron accepting strength and photonic property can be tuned by incorporating suitable functionality at appropriate position. BODIPY units when incorporated into the dendrimer system creates unique and novel class of supramolecules with interesting photophysical and electrochemical properties2 due to the long excited-state lifetime, photo chemical stability and possess good solubility in many solvent systems. BODIPY class of dyes are unique fluorophores with spectacular properties.3 BODIPY-based molecules show attractive applications in photovoltaics,4 optoelectronics,5 nonlinear optics,6 bioimaging or sensing,7 and photodynamic therapy,8 light-harvesting,9 and electron donor–acceptor10 systems and as of fluorescent probes for metal ions,11 components for molecular logic gates,12 and photosynthetic models13,14 and also in dye-sensitized solar cells.15 The electronic and photonic properties of BODIPY group are more interesting and useful in the field of supramolecular systems. 1,3-Dipolar cycloaddition16–18 between alkynes and azides by “click” chemistry is widely used in organic and bio-organic chemistry19 and chosen as an elective and convenient approach for the synthesize of triazole system. Triazole group is an ideal choice in supramolecular system to interact with many anions and cations.
Dye-sensitized solar cells (DSSC) are important alternative to the traditional semiconductor based solar cells because of the limits and problems that exist in the widely used constructs.20,21 The clean, renewable, free and readily available solar energy has triggered on the growing research interest on DSSCs. DSSC with the highest solar to electric energy efficiency of 13% was first reported by Gratzel in 1991.22 A practical DSSC has three vital components; (i) a ruthenium dye coated TiO2 working electrode (ii) a redox electrolyte comprising of a potassium iodide and iodine mixture in acetonitrile medium and (iii) a platinum counter electrode. It is clear that there are a number of factors that determine the efficiency of solar cells, but the structural and physical properties of the sensitizer are clearly important ones. Most of the recent research work focus on the modification of ruthenium based dyes. The ruthenium dyes while holding the record for conversion efficiencies has relatively low extinction coefficients, and further they are expensive and hard to purify. Motivated by the possibility of finding a replacement for the metal-complex dyes such as ruthenium dye, a number of chromophores, including coumarins, indolines,23,24 the porphyrin derivatives are particularly attractive as photo sensitizer's in DSSCs due to their structural similarity to chlorophylls in natural photosynthetic systems with tunable visible absorption properties.25 BODIPY dyes are likely to evolve as satisfactory alternatives to the traditional core of photo sensitizers. The absorption bands of the BODIPY dyes are highly tunable.26,27 Tris-BODIPY based supramolecular structure allows extension of the pi-conjugated system and thus reduces the HOMO–LUMO energy gap while the oxyethylene chains ensure chemical stability, good solubility and film forming properties of the molecules. Derivatives of BODIPY form an important class of fluorescent dyes that have attracted considerable interest in recent years owing to a unique combination of facile synthesis, stability and high photosensitizer properties.28,29 The present investigation deals with the synthesis of BODIPY conjugated dendrimer dyes 1, 2 and 3 and their optical, fluorescence and electrochemical properties along with their function as dye for harvesting solar energy in DSSC (Fig. 1).
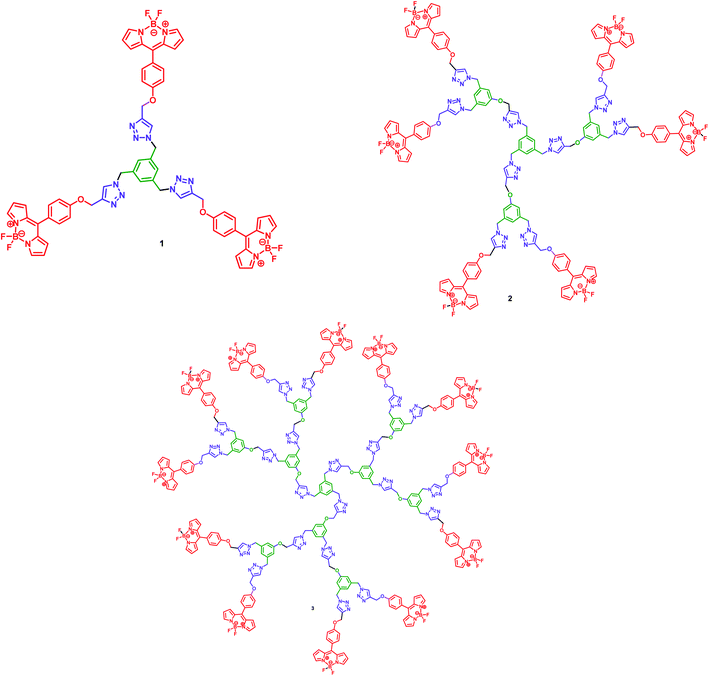 |
| Fig. 1 Molecular structure of BODIPY decorated triazole bridged dendrimers 1, 2 and 3. | |
Results and discussions
The BODIPY conjugated dendrimer dyes were synthesized by divergent strategy through click chemistry approach. The synthetic pathway leading to the chloro and azido dendrimers 6, 7, 8 and 9 is shown in Scheme 1. The divergent synthesis begins with the reaction of 1,3,5-tris(azido)benzene 5 with 3.1 equiv. of 1,3-bis(chloromethyl)-5-(propargyloxy)benzene 4 under Cu(I) catalyzed click reaction conditions gave the first generation chloro dendrimer 6 in 86% yield, which on further treatment with NaN3 in DMSO at 90 °C afforded the corresponding first generation azido dendrimer 7 in 92% yield. In 1H-NMR spectrum the compound 7 showed a singlet at δ 4.43 for the benzylic protons and two different singlets at δ 5.15, δ 5.60 for the O-methylene and N-methylene protons and a sharp singlet at δ 7.02 for the triazole proton in addition to the signals for the aromatic protons. The 13C NMR spectrum of the compound 7 displayed benzylic carbon at δ 53.3 and signals at δ 47.8 and 61.2 for –N–CH2 and –O–CH2 carbons respectively and the triazole carbon appeared at δ 142.7 in addition to the signals for the aromatic carbons. Reaction of 1.0 equiv. of the azido dendrimer 7 with 6.2 equiv. of 1,3-bis(chloromethyl)-5-(propargyloxy)benzene 4 under Cu(I) catalyzed click reaction conditions gave the second generation chloro dendrimer 8 in 86% yield. The 1H-NMR spectrum of the compound 8 showed a singlet at δ 4.68 for the benzylic protons and two different singlets at δ 5.13, δ 5.54 for the O-methylene and N-methylene protons and a sharp singlet at δ 7.01 for the triazole protons in addition to the signals for the aromatic protons. The 13C NMR spectrum of the compound 8 displayed benzylic carbon at δ 52.6 and signals at δ 46.7 and 61.1 for –N–CH2 and –O–CH2 carbons respectively, and the triazole carbon appeared at δ 139.4 in addition to the signals for the aromatic carbons. Reaction of 1.0 equiv. of the chloro dendrimer 8 with 18.0 equiv. of NaN3 in DMSO at 90 °C for 3 h gave the azido dendrimer 9 in 88% yield. The 1H-NMR spectrum of the compound 9 showed a singlet at δ 4.41 for the benzylic protons and two different singlets at δ 5.10, δ 5.55 for O-methylene and N-methylene protons and a sharp singlet at δ 7.02 for the triazole protons in addition to the signals for the aromatic protons. The 13C NMR spectrum of the compound 7 displayed the benzylic carbon at δ 52.6 and signals at δ 52.3 and 61.2 for –N–CH2 and –O–CH2 carbons respectively and the triazole carbon appeared at δ 142.5 in addition to the signals for the aromatic carbons. Further, the constitution of the dendritic wedges 7 and 9 was also confirmed from elemental analysis (Scheme 1).
 |
| Scheme 1 Reagents and conditions: (i) CuSO4 (5 mol%), sodium ascorbate (10 mol%), H2O–THF (1 : 1); rt, 12 h, 6 (86%); (ii) NaN3, (9 equiv./18 equiv.), DMSO, 90 °C, 3 h, 7 (92%). (iii) CuSO4 (5 mol%), sodium ascorbate (10 mol%), H2O–THF (1 : 1); rt, 12 h, 8 (86%). (iv) NaN3, (9 equiv./18 equiv.), DMSO, 90 °C, 3 h, 9 (88%). | |
The synthetic pathway leading to the propargylated BODIPY derivative 12 is shown in Scheme 2. Reaction of 1.0 equiv. of 4-(prop-2-ynyloxy)benzaldehyde 10 with 2.1 equiv. of pyrrole in the presence of TFA, dry DCM at room temperature for 8 h gave the 2,2′-((4-(prop-2-ynyloxy)phenyl)methylene)bis(1H-pyrrole) 11 as white solid in 87% yield, which on further treatment with TEA, DDQ in dry DCM at room temperature for 1.5 h and BF3·OEt2 in dry DCM at 0 °C for 3 h gave 5,5-difluoro-10-(4-(prop-2-ynyloxy)phenyl)-5H-dipyrrolo[1,2-c:1′,2′-f][1,3,2]diazaborinin-4-ium-5-uide (BODIPY) 12 in 74% yield (Scheme 2).
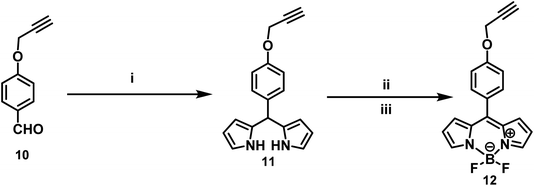 |
| Scheme 2 Reagents and conditions: (i) pyrrole (2.1 equiv.), TFA (0.1 mL), dry DCM, rt, 8 h, 11 (87%), (ii) TEA (10 mL), DDQ in dry DCM, rt, 1.5 h; (iii) BF3·OEt2 (10 mL), dry DCM, 0 °C, 3 h, 12 (74%). | |
The 1H NMR spectrum of the propargylated BODIPY 12 displayed a triplet at δ 3.71 for the acetylenic protons, a doublet at δ 4.71 for the propargyl methylene protons and a sharp singlet at δ 5.12 for O–CH2 proton in addition to the signals for the aromatic protons. The 13C NMR spectrum of 12 showed the BODIPY carbons at δ 114.9, 127.1, 143.6, 147.1, and 159.9 in addition to the signals for the other aliphatic and aromatic carbons.
The synthesis of the BODIPY dendrimer 1, 2 and 3 is shown in Scheme 3. Reaction of 3.1/6.1/12.1 equiv. of the propargylated BODIPY 12 with 1.0 equiv. of the azidodendrimer 5, 7 and 9 in the presence of Cu(I) catalyzed click chemistry reaction conditions gave BODIPY dendrimer 1, 2 and 3 in 86%, 88% and 85% yields, respectively (Scheme 3).
 |
| Scheme 3 Reagents and conditions: (i) CuSO4 (5 mol%), sodium ascorbate (10 mol%), H2O–THF (1 : 1); rt, 12 h. 1 (86%), 2 (88%), 3 (85%). | |
The 1H NMR spectrum of the BODIPY dendrimer 1 showed the BODIPY protons as singlets at δ 5.29 and 5.57, the triazole protons also as singlets at δ 7.66 and 7.72 in addition to the signals for the other aliphatic and aromatic protons. The 13C NMR spectrum of the dendrimer 1 showed three different BODIPY carbons at δ 68.7, 68.8, 69.0 and 69.2 and the triazole carbons at δ 139.3 and 143.6 in addition to the signals for the other aliphatic and aromatic carbons. The appearance of molecular ion peak at m/z 751.36 [M]+ in mass spectrum also confirmed the structure of the BODIPY dendrimer 1. Similarly, the structure of the BODIPY dendrimer 2 and 3 was also confirmed from the spectral and analytical data.
Photophysical studies
The UV-vis absorption spectra of all the BODIPY dendrimers 1, 2 and 3 were obtained in DCM (1 × 10−5 M) and the absorption maxima are listed in Table 1. The UV-visible spectra of the G0, G1 and G2 dendrimers 1, 2 and 3 shows absorption peak at 386 and 499 nm, 385 and 500 nm and 384 and 501 nm respectively (Fig. 2a) due to the BODIPY unit present in the dendrimeric system 1, 2 and 3. The absorption intensity increases from the lower to higher generation dendrimers, due to the increasing number of triazole and BODIPY units in the dendritic system though λmax remains almost constant at a given concentration of the of the dendrimers 1, 2 and 3 (Table 1) and hence, the dendrimer 3 has the highest light absorption efficiency due to more number of BODIPY units than the dendrimers 1, 2, which is usually called valence effect in dendrimer chemistry. Further, the optical density also increases linearly with respect to the number of BODIPY units which is shown in Fig. 2b. In conclusion, for the dendrimers 1, 2 and 3 the absorption intensity increases on increasing the dendritic generation.
Table 1 Absorption and emission maximum of dendrimers 1, 2 and 3 in CH2Cl2 at 1 × 10−5 M
Entry no. |
λabs max (nm) |
Molar extinction coefficient (ε) L mol−1 cm−1) |
λem [nm] |
Quantum yield (ΦF) |
1 (G0) |
384, 499 |
6000, 37 300 |
507 |
0.18 |
2 (G1) |
385, 500 |
9300, 51 700 |
508 |
0.34 |
3 (G2) |
386, 501 |
14 600, 75 000 |
509 |
0.46 |
 |
| Fig. 2 (a) UV and visible absorption spectra of the BODIPY dendrimers 1, 2 and 3 in DCM (1 × 10−5 M) at room temperature. (b) Variation of optical density in UV and visible absorption spectra with increase in the number of the BODIPY units in the dendrimers 1, 2 and 3. | |
The fluorescence spectra of the BODIPY dendrimers 1, 2 and 3 in DCM at room temperature is shown in Fig. 3. The BODIPY dendrimers 1, 2 and 3 when excited at 490 nm, exhibited the emission bands at 507, 508 and 509 nm, respectively (Fig. 3 and Table 1). The increase in fluorescence intensity of the BODIPY dendrimers with increase in dendritic generation is due to the presence of increased number of triazolyl and BODIPY units, otherwise known as multivalency effect in dendrimer chemistry.30,31
 |
| Fig. 3 Fluorescence spectra of the BODIPY dendrimers 1, 2 and 3 in DCM (1 × 10−5 M) at room temperature. | |
From the fluorescence spectrum the quantum yield of the synthesised BODIPY dendrimers 1, 2 and 3 were determined in dichloromethane and shown in Table 1, and compared with the fluorescence quantum yield of Rhodamine B as the standard. Fluorescence quantum yields (ΦF) were calculated by the comparative method [eqn (1)].32
|
ΦF = ΦF(std)FAstdη2FstdAη2std
| (1) |
where
ΦF is the fluorescence quantum yield of the standard
viz. Rhodamine B was employed as the standard (
ΦF = 0.76 in ethanol).
F and
Fstd are the areas under the fluorescence emission curves of the dendrimers
1,
2 and
3 and the standard, respectively.
A and
Astd are the respective absorbance of the samples and standard at the excitation wavelengths.
η2 and
η2std are the refractive indices of solvents used for the sample and standard, respectively. The concentration of the solutions at the excitation wavelength was fixed as 1 × 10
−5 M. The fluorescence quantum yields of the synthesised dendrimer increases with the increase in the generation of the dendrimers from zeroth to second generation dendrimer in dichloromethane and are found to be 0.18, 0.34 and 0.46 the dendrimer
1,
2 and
3 respectively.
Lifetime decay analysis
Lifetime decay analysis was carried out on all the fluorescent BODIPY dendrimers 1, 2 and 3 by using the IBH time-correlated single photon counting (TCSPC) technique on excitation at 490 nm in CH2Cl2 as solvent. The fluorescence decay (Fig. 4) of the dendrimer 2 show longer relaxation times τ2 than for the dendrimer 1 and 3. The relaxation time τ1 decreases with increases in the dendrimer generation. The relaxation time τ2 is greater than τ1 for all the dendrimer and it increases from the dendrimer 1 to 2. However the relaxation time τ2 decreases for the second generation dendrimer 3 which could be due to fluorescence quenching because of molecular crowding. In general the fluorescence lifetime increases as the generation of the dendrimer increases, which could be due to greater number of triazole and BODIPY units in the higher generation dendrimers.
 |
| Fig. 4 Lifetime decay of the BODIPY triazolyl dendrimers 1, 2, and 3 (prompt = instrument response function). | |
The lifetime decay is biexponential with lifetimes τ1 and τ2. The lifetime decay values of the BODIPY dendrimers 1, 2 and 3 are τ1 = 0.43 ns and τ2 = 2.53 ns, τ1 = 0.51 ns and τ2 = 3.57 ns, τ1 = 0.90 ns and τ2 = 1.62 ns, respectively (Table 2).
Table 2 Laser parameters for the dendrimers 1, 2 and 3 in CH2Cl2 at 1 × 10−5 M
S. no. |
τ1 (ns) |
τ2 (ns) |
A1 (%) |
A2 (%) |
τAvg. (ns) |
Red. χ2 |
1 (G0) |
0.43 |
2.53 |
90.61 |
9.39 |
4.69 |
1.28 |
2 (G1) |
0.51 |
3.57 |
91.20 |
8.80 |
5.54 |
1.25 |
3 (G2) |
0.90 |
1.62 |
65.50 |
34.50 |
1.07 |
1.27 |
Electrochemical studies
The redox property of the BODIPY conjugated dendrimers 1, 2 and 3 was carried out using cyclic voltammetry (CV) technique.33 The cyclic voltammogram for dendrimer 1 to 3 was obtained in the potential range of 0.0 V to 1.0 V at room temperature in CH2Cl2 containing 0.1 M TBAP as the supporting electrolyte at the scan rate 50 mV s−1 (Fig. 5) with glassy carbon (GC) electrode as the working electrode, platinum wire as the supporting electrode and Ag/AgCl as the reference electrode in CH2Cl2 using 0.1 M [nBuN][PF6] as supporting electrolyte. The reversible oxidation potential for the BODIPY conjugated dendrimers 1–3 is found to be 662, 638 and 600 mV and the reversible reduction peak is 594, 575 and 522 mV respectively. On increasing the dendrimer generation from 1 to 3 there is an increase in the redox peak potential from less positive to more positive peak potential due to the presence of more number of triazolyl and BODIPY units in 3 than in 1 and 2, though the current intensity remains constant (Table .3).
 |
| Fig. 5 Cyclic voltammogram of BODIPY conjugated dendrimers 1, 2 and 3 in DCM (1 × 10−5 M) at room temperature (scan rate at 50 mV s−1) and 0.1 M tetrabutyl ammonium perchlorate as a supporting electrolyte in dry DCM. | |
Table 3 Cyclic voltammetry parameters of the BODIPY dendrimers 1, 2 and 3 in DCM (1 × 10−5 M)
S. no. |
Cyclic voltammetry |
Epc |
Epa |
ΔE = (Epc − Epa) |
1 (G0) |
0.662 |
0.594 |
0.068 |
2 (G1) |
0.638 |
0.575 |
0.063 |
3 (G2) |
0.600 |
0.522 |
0.078 |
The BODIPYconjugated dendrimers 1, 2 and 3 exhibit oxidation and reduction waves. The oxidation and reduction waves correspond to the BODIPY moiety.34 The oxidation potential of the BODIPYconjugated dendrimers 1, 2 and 3 follows the order 3 > 2 > 1. The current response of the BODIPY conjugated dendrimers 3 is much higher than the BODIPY conjugated dendrimers 2 and 1, due to strong repulsive force operating with electron deficient BODIPY unit in dendrimers 1 and 2. The electron deficient BODIPY unit withdraws the electron density thereby making oxidation much difficult in the synthesised dendrimers.
DSSC studies
In an attempt to improve the solar energy harvesting performance in the solar cells, studies have been carried out to examine the role of the BODIPY based dye in DSSC. Fig. 6 shows the current density (Jsc) and voltage (Voc) characteristics of the cell with the BODIPY dendrimer 1, 2 and 3 under simulated solar illumination of 70 mW cm−2 and the parameters are listed in Table 4.
 |
| Fig. 6 Photovoltaic properties of the BODIPY dendrimers 1, 2, 3 in dye-sensitized solar cells under the illumination of 100 mW cm−2. | |
Table 4 Photovoltaic properties of the BODIPY dendrimer based dye-sensitized solar cells under the illumination of 70 mW cm−2
System |
Voltage (mV) |
Current (mA) |
Fill factor |
Efficiency (η) % |
TiO2/BODIPY dyes conjugated dendrimer 1/KI/I2/Pt |
670 |
3.4 |
0.51 |
1.7 |
TiO2/BODIPY dyes conjugated dendrimer 2/KI/I2/Pt |
698 |
4 |
0.52 |
2.1 |
TiO2/BODIPY dyes conjugated dendrimer 3/KI/I2/Pt |
725 |
4.7 |
0.52 |
2.5 |
In the current investigation cost effective BODIPY dyes were designed so as to have certain special features that could make them highly advantageous compared to other organic dyes, and thus, they are promising from the angle of stability and performance in harvesting solar energy. The presence of the BODIPY based dendrimer in DSSC shows an improved fill factor (FF) value of 52%, which is relatively high for BODIPY based DSSC and suggests that the more extended pi-conjugated structure of BODIPY may lead to better hole-transporting properties and/or better charge collection at the working (BODIPY) dye coated on TiO2 and counter Pt electrode.35 The efficient light-harvesting abilities of the dyes led to high Jsc values for these dye-loaded cells and report power conversion efficiency of 2.5%, a value which ranks the good reported for BODIPY based solar cells. The BODIPY dendrimer 1, 2, 3 has anchoring units such as triazolyl and BODIPY groups and hence the power conversion efficiency increases from lower to higher generation dendrimer due to the increasing BODIPY group in the surface, which is beneficial for the design of organic sensitizers and the results confirms that the synthesised BODIPY dye based dendrimer plays key role in dye-sensitized solar cells. The detailed electron transfer mechanism occurring in the present DSSC based on the TiO2/BODIPY dye/KI/I2/Pt system is shown in Fig. 7.
 |
| Fig. 7 The schematic cell diagram for TiO2/BODIPY dye/KI/I2/Pt. | |
The BODIPY dendrimers 1, 2 and 3 are strongly adsorbed on the TiO2 surface in the DSSC. All the dendrimers 1, 2 and 3 were independently mixed with TiO2 and after 3 h, the TiO was separated washed with water and UV-vis spectra was recorded by DRS technique. The TiO2 sample adsorbed with dendrimer 1, 2, and 3 showed the λmax in the UV-visible spectra at 384 and 501 nm, 385 and 502 nm and 386 and 503 nm respectively (ESI, Fig. S1†) and this is reflected in SEM analysis also (refer ESI, Fig. S2†). When BODIPY unit alone is used as dye in the DSSC, solar energy harvesting efficiency was reported to be very less (η = 1.7, 2.1, 2.5).36 However dendrimer with the BODIPY unit along with traizole bridging unit shows better power conversion efficiency. Hence, it is concluded that triazole unit is responsible for the adsorption of the dye at the TiO2 surface and the presence of BODIPY unit helps in increasing the solar energy harvesting efficiency in DSSC (ESI, Fig. S3†).
Experimental
All reagents were obtained commercially and used as such unless otherwise stated. Analytical TLC was performed on commercial Merk plates coated with Silica Gel GF254. Analytical samples were obtained from silica gel chromatography, using silica gel of 100–200 mesh and elution with the solvent system as mentioned under each Experiment section. The melting points were determined by using a Metler Toledo melting point apparatus by open capillary tube method and were uncorrected. 1H and 13C NMR spectra were recorded on a 300 MHz BRUKER AVANCE (75 MHz for 13C NMR) spectrometer. UV-vis absorption spectrum was measured with a PerkinElmer Lambda 35 UV-vis spectrometer.
BODIPY was adsorbed on the TiO2 surface by soaking the TiO2 photoelectrode in an ethanol solution of the dye at a concentration of (5 × 10−4 M) for 24 h at room temperature. The photoelectrode was washed dried and used for the measurement of photovoltaic performance. A sandwich type photoelectrochemical cell was composed of a dye-coated TiO2 photoanode. Platinum coated fluorinated tin oxide (FTO) conducting glass act as a counter electrode.37 The electrolyte solution was injected into the space between two electrodes. The electrolyte solution was composed of 4.1 × 10−2 M of KI, 8 × 10−3 M of I2, and dimethylpropylimidazolium iodide of 5.1 × 10−2 in 10 mL acetonitrile–ethanol (80–20%) solvent. The solar to electric energy conversion efficiency was measured under simulated solar light at 70 mW cm−2. The photocurrent–photovoltage (I–V) was measured using a BAS 100A electrochemical analyzer. The apparent cell area of TiO2 photoelectrode was 1 cm2 (1 cm × 1 cm).38,39 The cell configuration of the BODIPY based dye-sensitized solar cells is TiO2/BODIPY dye/KI/I2/Pt.
General procedure for the Cu-catalyzed Huisgen ‘click reaction’ (procedure A)
A solution of the azide (1.0 equiv.), alkyne (3.0 equiv./6.0 equiv./12.0 equiv.), CuSO4·5H2O (5 mol%) and NaAsc (10 mol%) in a mixture of THF–H2O (1
:
1, 20 mL) was stirred for 12 h at room temperature. The residue obtained after evaporation of the solvent was dissolved in CHCl3 (150 mL) and washed with NH4Cl solution (50 mL) and brine (50 mL) and dried over Na2SO4 and then concentrated to give a residue, which was purified by column chromatography (SiO2), using the eluent as mentioned under each compound.
General procedure for the first generation azido dendron (procedure B)
The reaction flask was charged with the chloro compound (1 equiv.), DMSO (10 mL) and added NaN3 (9.0 equiv./18 equiv. (1.5 equiv. per chloride)) and the mixture was heated up to 90 °C for 3 h and the completion of the reaction was monitored by TLC using MeOH
:
CHCl3 (1
:
19). The reaction mixture was then cooled to room temperature and diluted with H2O (100 mL) and extracted with CH2Cl2 (2 × 150 mL). The organic layer was washed with saturated NaCl, dried over Na2SO4 and evaporated to give the corresponding azido compound as a solid.
1,3-Bis(chloromethyl)-5-(prop-2-ynyloxy)benzene 4
1,3-Bis(chloromethyl)-5-(prop-2-ynyloxy)benzene 4 was obtained as white solid as per the reported procedure28 from 1,3-bis(hydroxymethyl)-5-propargyloxybenzene (1 g, 5.2 mmol) and thionyl chloride (1.52 mL, 20.83 mmol). Yield: 87%; mp: 88–90 °C; 1H NMR (300 MHz, CDCl3): δH 2.54 (t, 1H, J = 2.1 Hz), 4.55 (s, 4H), 4.71 (d, 2H, J = 2.4 Hz), 6.90 (s, 2H), 7.04 (s, 1H); 13C NMR (75 MHz, CDCl3): δC 45.7, 56.0, 76.0, 78.1, 115.1, 121.8, 139.4, 158.0; mass spectrum: MS (ES): m/z = 229 (M+).
First generation chlorodendron 6
Following the general procedure A, reaction of 1,3-bis(chloromethyl)-5-(propargyloxy)benzene 4 (1.17 g, 5.1 mmol), with 1,3,5-tris(azidomethyl)benzene 5 (0.4 g, 1.7 mmol) gave the chlorodendron 6 (1.36 g) as light yellow amorphous solid which was used as such without purification. Yield: 86%; 1H NMR: (300 MHz, DMSO-d6): δH 4.54 (s, 12H), 5.20 (s, 6H), 5.52 (s, 6H), 6.95 (s, 6H), 7.05 (s, 3H), 7.16 (s, 3H), 7.70 (s, 3H); 13C NMR (75 MHz, DMSO): δC 45.6, 53.2, 61.9, 114.9, 121.4, 123.3, 127.7, 136.7, 139.4, 142.9, 158.5; mass spectrum: MS (ES): m/z = 927 (M+).
First generation azidodendron 7
Following the general procedure B, reaction of the first generation chlorodendron 6 (1 g, 1.08 mmol) with NaN3 (0.7 g, 10.8 mmol) gave the azide 7 (0.97 g) as light brown solid. Yield: 92%; mp: 101–103 °C; 1H NMR: (300 MHz, CDCl3): δH 4.43 (s, 12H), 5.15 (s, 6H), 5.60 (s, 6H), 6.97 (s, 3H), 7.02 (s, 6H), 7.30 (s, 3H), 8.28 (s, 3H); 13C NMR (75 MHz CDCl3): δC 53.3, 61.2, 63.7, 114.3, 120.6, 124.8, 127.7, 137.1, 137.7, 142.7, 158.4; mass spectrum: MS (ES): m/z = 969 (M+).
Second generation chlorodendron 8
From the reaction of 1,3-bis(chloromethyl)-5-propargyloxybenzene 4 (0.21 g, 0.92 mmol, 6.2 equiv.) and the azidodendron 7 (0.15 g, 0.15 mmol, 1.0 equiv.) using the general procedure A for click chemistry, the chlorodendron 8 was obtained as a light brown amorphous solid after eluting from the column with CHCl3
:
MeOH (19
:
1). Yield: 0.3 g, 86%; mp: 201 °C; 1H NMR: (300 MHz, DMSO-d6): δH 4.68 (s, 24H); 5.04 (s, 6H); 5.13 (s, 12H); 5.54 (s, 12H); 5.57 (s, 6H); 6.93–7.01 (s, 27H); 7.25 (s, 3H); 8.23 (s, 3H); 8.28 (s, 6H); 13C NMR: (75 MHz, DMSO-d6): δC 46.7, 52.6, 61.1, 61.2, 114.2, 115.0, 121.6, 130.7, 137.8, 139.4, 158.1, 158.4; MS (MALDI-TOF): m/z = 2467 (M2+).
Second generation azidodendron 9
Following the general procedure B, reaction of the second generation chlorodendron 8 (0.2 g, 0.083 mmol, 1.0 equiv.) with NaN3 (0.08 g, 1.25 mmol, 15.0 equiv.) gave the azidodendron 9 as light brown solid from the column after eluting with CHCl3
:
MeOH (19
:
1). Yield: 0.17 g, 85%; mp: 180 °C; 1H NMR: (300 MHz, DMSO-d6): δH 4.41 (s, 24H); 5.10 (s, 6H); 5.15 (s, 12H); 5.55 (s, 12H); 5.58 (s, 6H); 6.9–7.02 (m, 27H); 7.29 (s, 3H); 8.25 (s, 3H); 8.29 (s, 6H); 13C NMR: (75 MHz, DMSO-d6): δC 52.3, 52.6, 53.3, 61.2, 114.2, 114.3, 120.0, 120.6, 124.8, 127.6, 137.1, 137.6, 137.9, 142.5, 142.8, 158.3; MS (MALDI-TOF): m/z = 2446 (M+).
5,5-Difluoro-10-(4-(prop-2-ynyloxy)phenyl)-5H-dipyrrolo[1,2-c:1′,2′-f][1,3,2]diazaborinin-4-ium-5-uide 12
Following the general procedure, 5,5-difluoro-10-(4-(prop-2-ynyloxy)phenyl)-5H-dipyrrolo[1,2-c:1′,2′-f][1,3,2]diazaborinin-4-ium-5-uide 12 was obtained as dark brown solid from 2,2′-((4-(4-(prop-2-ynyloxy)benzyloxy)phenyl)methylene)bis(1H-pyrrole) 10 (0.5 g, 1.8 mmol) and BF3·OEt2 (10 mL). Yield: 87%; mp: 80–82 °C; 1H NMR: (300 MHz, CDCl3): δH 2.61 (t, J = 2.1 Hz, 1H), 4.71 (d, J = 2.4 Hz, 2H), 6.17–6.20 (m, 2H), 6.73 (d, J = 1.5 Hz, 2H), 6.96 (d, J = 8.7 Hz, 2H), 7.18 (d, J = 8.7 Hz, 2H), 7.98 (s, 2H); 13C NMR: (75 MHz, CDCl3): δC 56.0, 76.3, 77.9, 114.9, 118.4, 127.1, 131.4, 132.3, 134.9, 143.6, 147.1, 159.9; mass spectrum: MS (FAB): m/z = 322 [M+]; elemental analysis calculated for: C18H13BF2N2O; C, 67.12 H, 4.07; found: C, 67.01 H, 4.02.
BODIPY dendrimer 1
The BODIPY dendrimer 1was synthesized from 1,3,5-tris(azidomethyl)benzene 4 (1.0 mmol, 1 equiv.), and the BODIPY alkyne 12 (3.0 mmol, 3.0 equiv.), in the presence of CuSO4·5H2O (5 mol%) and NaAsc (10 mol%) in a mixture of THF–H2O (1
:
1, 20 mL) following the procedure A. The residue obtained after evaporation of the solvent was dissolved in CHCl3 (150 mL) and washed with NH4Cl solution (50 mL) and brine (50 mL) and dried over anhydrous Na2SO4 and then concentrated to give a residue, which was purified by column chromatography with CHCl3
:
MeOH (25
:
1) as eleut. Yield: 85%; 1H NMR: (300 MHz, CDCl3): δH 5.29 (s, 6H), 5.56 (s, 6H), 6.53–6.54 (s, 6H), 6.93–6.96 (s, 6H), 7.12–7.15 (s, 6H), 7.26 (s, 3H), 7.52–7.55 (s, 6H) 7.67 (s, 3H), 7.91 (s, 6H); 13C NMR: (75 MHz, CDCl3): δC 53.6, 62.1, 114.8, 118.4, 123.0, 126.9, 127.3, 127.8, 131.3, 132.4, 134.8, 136.4, 143.6, 144.2, 160.6; MS (MALDI): m/z = 1209.58 [M + Na]+; elemental anal. calcd for C63H48B3F6N15O3: C, 62.56%; H, 4.00%; N, 17.37%; found: C, 62.46%; H, 4.09%; N, 17.47%.
BODIPY dendrimer 2
The BODIPY dendrimer 2 was synthesized from 1,3,5-tris(azidomethyl)benzene 4 (1.0 mmol, 1 equiv.), BODIPY alkyne 12 (6.0 mmol, 6.0 equiv.), in the presence of CuSO4·5H2O (5 mol%) and NaAsc (10 mol%) in a mixture of THF–H2O (1
:
1, 20 mL) following the procedure A. The residue obtained after evaporation of the solvent was dissolved in CHCl3 (150 mL) and washed with NH4Cl solution (50 mL) and brine (50 mL) and dried over anhydrous Na2SO4 and then concentrated to give a residue, which was purified from column chromatography with CHCl3
:
MeOH (25
:
1) as eleut. Yield: 88%; 1H NMR: (300 MHz, CDCl3): δH 4.80 (s, 24H), 4.95 (s, 3H), 5.96 (s, 6H), 5.01 (s, 6H), 6.56–6.59 (m, 19H), 6.61 (s, 8H), 6.98–7.12 (m, 12H), 7.51 (s, 5H), 7.54 (s, 16H), 7.57 (s, 4H), 7.92 (s, 14H); 13C NMR: (75 MHz, CDCl3): δC 56.0, 62.1, 114.0, 114.8, 114.9, 118.4, 127.1, 128.9, 131.4, 132.2, 132.3, 134.9, 139.3, 141.54, 143.6, 147.1, 159.9; MS (MALDI): m/z = 2902.64 [M + Na]+; elemental anal. calcd for C150H117B6F12N39O9: C, 62.07%; H, 4.06%; N, 18.82%; found: C, 62.17%; H, 4.09%; N, 18.85%.
BODIPY dendrimer 3
The BODIPY dendrimer 3 was synthesized from 1,3,5-tris(azidomethyl)benzene 4 (1.0 mmol, 1 equiv.), and the BODIPY alkyne 12 (12.0 mmol, 12.0 equiv.), in the presence of CuSO4·5H2O (5 mol%) and NaAsc (10 mol%) in a mixture of THF–H2O (1
:
1, 20 mL) following the procedure A. The residue obtained after evaporation of the solvent was dissolved in CHCl3 (150 mL) and washed with NH4Cl solution (50 mL) and brine (50 mL) and dried over anhydrous Na2SO4 and then concentrated to give a residue, which was purified from column chromatography with CHCl3
:
MeOH (25
:
1) as eleut. Yield: 85%; 1H NMR: (300 MHz, CDCl3): δH 5.27 (s, 12H), 5.37 (s, 24H), 5.46 (s, 40H), 6.51 (s, 19H), 6.80–6.83 (m, 19H), 6.90 (s, 20H), 7.10–7.11 (m, 24), 7.47–7.50 (m, 15H), 7.51–7.55 (m, 32H), 7.70 (s, 22H), 7.89 (s, 28H); 13C NMR: (75 MHz, CDCl3): δC 56.0, 62.1, 114.1, 114.8, 114.9, 118.4, 127.4, 127.1, 128.9, 131.4, 132.2, 132.3, 134.9, 139.3, 141.5, 143.6, 147.1, 159.9; MS (MALDI): m/z = 4096.19 [M + Na]+; elemental anal. calcd for C324H255B12F24N87O21: C, 61.88%; H, 4.09%; N, 19.38%; found: C, 61.97%; H, 4.09%; N, 19.45%.
Conclusion
The triazole bridged BODIPY conjugated dendrimers 1, 2 and 3 were obtained up to third generation. In the UV-vis spectra the absorption intensity linearly increases with increase in the number of BODIPY group. In CV the current intensity increases with increase in the dendrimer generation. Dendrimer 3 shows increase in the solar energy harvesting efficiency of 2.5% when used as the dye material which ranks good among the reported BODIPY based dye compounds.
Conflicts of interest
The authors have no conflicts of interest.
Acknowledgements
The authors thank DST-FIST for providing an NMR facility to the Department of Organic Chemistry and University of Madras, Chennai 600025, India. SG thanks the financial assistance from DST Fast Track (SB/FT/CS-063/2013), New Delhi for the solar cell studies.
References
-
(a) R. Misra, T. Jadhav, B. Dhokale, P. Gautam, R. Sharma, R. Maragani and S. M. Mobin, Dalton Trans., 2014, 43, 13076 RSC;
(b) R. Misra, B. Dhokale, T. Jadhav and M. Shaikh Mobin, New J. Chem., 2014, 38, 3579 RSC.
- R. Misra, B. Dhokale, T. Jadhav and S. M. Mobin, Dalton Trans., 2013, 42, 13658 RSC.
-
(a) A. B. Nepomnyashchii and A. J. Bard, Acc. Chem. Res., 2012, 45, 1844 CrossRef CAS PubMed;
(b) T. Cheng, T. Wang, W. Zhu, X. Chen, Y. Yang, Y. Xu and X. Qian, Org. Lett., 2011, 13, 3656 CrossRef CAS PubMed;
(c) O. A. Bozdemir, R. Guliyev, O. Buyukcakir, S. Selcuk, S. Kolemen, G. Gulseren, T. Nalbantoglu, H. Boyaci and E. U. Akkaya, J. Am. Chem. Soc., 2010, 132, 8029 CrossRef CAS PubMed;
(d) M. Benstead, G. H. Mehl and R. W. Boyle, Tetrahedron, 2011, 67, 3573 CrossRef CAS.
-
(a) S. E. Ela, M. D. Ylimaz, B. Icil, Y. Dede, S. Icli and E. U. Akkaya, Org. Lett., 2008, 10, 3299 CrossRef PubMed;
(b) D. Kumaresan, R. P. Thummel, T. Bura, G. Ulrich and R. Ziessel, Chem.–Eur. J., 2009, 15, 6335 CrossRef CAS PubMed;
(c) S. Kolemen, Y. Cakmak, S. E. Ela, Y. Altay, J. Brendel, M. Thelakkat and E. U. Akkaya, Org. Lett., 2010, 12, 3812 CrossRef CAS PubMed.
-
(a) M. T. Whited, P. I. Djurovich, S. T. Roberts, A. C. Durrell, C. W. Schlenker, S. E. Bradforth and M. E. Thompson, J. Am. Chem. Soc., 2011, 133, 88 CrossRef CAS PubMed;
(b) A. Florian, M. J. Mayoral, V. Stepanenko and G. Fernandez, Chem.–Eur. J., 2012, 18, 14957 CrossRef CAS PubMed.
-
(a) Q. Zheng, G. Xu and P. Prasad, Chem.–Eur. J., 2008, 14, 5812 CrossRef CAS PubMed;
(b) P. Didier, G. Ulrich, Y. Mely and R. Ziessel, Org. Biomol. Chem., 2009, 7, 3639 RSC;
(c) P. A. Bouit, K. Kamada, P. Feneyrou, G. Berginc, L. Toupet, O. Maury and C. Andraud, Adv. Mater., 2009, 21, 1151 CrossRef CAS.
-
(a) J. Murtagh, D. O. Frimannsson and D. F. O'Shea, Org. Lett., 2009, 11, 5386 CrossRef CAS PubMed;
(b) W. J. Shi, J. Y. Liu and D. K. P. Ng, Chem.–Asian J., 2012, 7, 196 CrossRef CAS PubMed;
(c) S. Madhu, R. Gonnade and M. Ravikanth, J. Org. Chem., 2013, 78, 5056 CrossRef CAS PubMed.
-
(a) T. Yogo, Y. Urano, Y. Ishitsuka, F. Maniwa and T. Nagano, J. Am. Chem. Soc., 2005, 127, 12162 CrossRef CAS PubMed;
(b) S. Ozlem and E. U. Akkaya, J. Am. Chem. Soc., 2009, 131, 48 CrossRef CAS PubMed.
-
(a) J. Warnan, F. Buchet, Y. Pellegrin, E. Blart and F. Odobel, Org. Lett., 2011, 13, 3944 CrossRef CAS PubMed;
(b) J. Y. Liu, M. E. E. Khouly, S. Fukuzumi and D. K. P. Ng, Chem.–Eur. J., 2011, 17, 1605 CrossRef CAS PubMed;
(c) J. Iehl, J. F. Nierengarten, A. Harriman, T. Bura and R. Ziessel, J. Am. Chem. Soc., 2012, 134, 988 CrossRef CAS PubMed.
-
(a) C. A. Wijesinghe, M. E. E. Khouly, J. D. Blakemore, M. E. Zandler, S. Fukuzumi and F. D'Souza, Chem. Commun., 2010, 46, 3301 RSC;
(b) C. A. Wijesinghe, M. E. E. Khouly, N. K. Subbaiyan, M. Supur, M. E. Zandler, K. Ohkubo, S. Fukuzumi and F. D'Souza, Chem.–Eur. J., 2011, 17, 3147 CrossRef CAS PubMed.
-
(a) S. Atilgan, T. Ozdemir and E. U. Akkaya, Org. Lett., 2008, 10, 4065 CrossRef CAS PubMed;
(b) R. Guliyev, A. Coskun and E. U. Akkaya, J. Am. Chem. Soc., 2009, 131, 9007 CrossRef CAS PubMed.
-
(a) O. A. Bozdemir, R. Guliyev, O. Buyukcakir, S. Selcuk, S. K. lemen, G. Gulseren, T. Nalbantoglu, H. Boyaci and E. U. Akkaya, J. Am. Chem. Soc., 2010, 132, 8029 CrossRef CAS PubMed;
(b) R. Guliyev, S. Ozturk, Z. Kostereli and E. U. Akkaya, Angew. Chem., 2011, 123, 10000 (Angew. Chem., Int. Ed., 2011, 50, 9826) CrossRef.
-
(a) S. Ozlem and E. U. Akkaya, J. Am. Chem. Soc., 2009, 131, 48 CrossRef CAS PubMed;
(b) H. He, P. C. Lo, S. L. Yeung, W. P. Fong and D. K. P. Ng, J. Med. Chem., 2011, 54, 3097 CrossRef CAS PubMed.
-
(a) R. Ziessel and A. Harriman, Chem. Commun., 2011, 47, 611 RSC;
(b) C. Y. Lee, O. K. Farha, B. J. Hong, A. A. Sarjeant, S. T. Nguyen and J. T. Hupp, J. Am. Chem. Soc., 2011, 133, 15858 CrossRef CAS PubMed.
-
(a) S. E. Ela, M. D. Yilmaz, B. Icli, Y. Dede, S. Icli and E. U. Akkaya, Org. Lett., 2008, 10, 3299 CrossRef PubMed;
(b) S. Kolemen, Y. Cakmak, S. E. Ela, Y. Altay, J. Brendel, M. Thelakkat and E. U. Akkaya, Org. Lett., 2010, 12, 3812 CrossRef CAS PubMed.
- R. Huisgen, Angew. Chem., Int. Ed. Engl., 1968, 7, 321 CrossRef CAS.
- A. Kannan, V. Saravanan and P. Rajakumar, Asian J. Org. Chem., 2016, 5, 1155 CrossRef CAS.
-
(a) V. Saravanan, A. Kannan and P. Rajakumar, New J. Chem., 2017, 41, 1714 RSC;
(b) V. Saravanan, A. Kannan and P. Rajakumar, Sens. Actuators, B, 2017, 242, 904 CrossRef CAS;
(c) V. Saravanan, A. Kannan and P. Rajakumar, New J. Chem., 2018, 42, 2504 RSC.
-
(a) H. C. Kolb, M. G. Finn and K. B. Sharpless, Angew. Chem., Int. Ed., 2001, 40, 2004 CrossRef CAS;
(b) C. Tornoe, C. Christensen and M. Meldal, J. Org. Chem., 2002, 67, 3057 CrossRef CAS PubMed.
- A. Hagfeldt and M. Gratzel, Acc. Chem. Res., 2000, 33, 269 CrossRef CAS PubMed.
- M. K. Nazeeruddin, A. Kay, I. Rodicio, R. Humphry-Baker, E. Muller, P. Liska, N. Vlachopoulos and M. Gratzel, J. Am. Chem. Soc., 1993, 115, 6382 CrossRef CAS.
- B. O'Regan and M. Gratzel, Nature, 1991, 353, 737 CrossRef.
- K. Hara, K. Sayama, Y. Ogha, A. Shinpo, S. Suga and H. Arakawa, Chem. Commun., 2001, 569 RSC.
- T. Horiuchi, H. Miura, K. Sumioka and S. Uchida, J. Am. Chem. Soc., 2004, 126, 12218 CrossRef CAS PubMed.
- C. Y. Lee and J. T. Hupp, Langmuir, 2010, 26, 3760 CrossRef CAS PubMed.
- Y. Cakmak, T. Nalbantoglu, T. Durgut and E. U. Akkaya, Tetrahedron Lett., 2014, 55, 538 CrossRef CAS.
- A. Treibs, F. Kreuzer and H. Liebigs, Ann. Chem., 1968, 718, 208 CAS.
- T. Rousseau, A. Cravino, T. Bura, G. Ulrich, R. Ziessel and J. Roncali, Chem. Commun., 2009, 1673 RSC.
- C. Yeon Lee and T. H. Joseph, Langmuir, 2010, 26, 3760 CrossRef PubMed.
- J. C. T. Carlson, L. G. Meimetis, S. A. Hilderbrand and R. Weissleder, Angew. Chem., Int. Ed., 2013, 52, 6917 CrossRef CAS.
- H. D. Agnew, R. D. Rohde, S. W. Millward, A. Nag, W. S. Yeo, J. E. Hein, S. M. Pitram, A. A. Tariq, V. M. Burns, R. J. Krom, V. V. Fokin, K. B. Sharpless and J. R. Heath, Angew. Chem., Int. Ed., 2009, 48, 4944 CrossRef CAS.
- F. S. Fery and D. Lavabre, J. Chem. Educ., 1999, 76, 1260 CrossRef.
- M. Poddar, V. Sharma, S. M. Mobin and R. Misra, Chem.–Asian J., 2018, 13, 4881 CrossRef PubMed.
- B. Dhokale, T. Jadhav, M. Shaikh Mobin and R. Misra, Dalton Trans., 2016, 45, 1476 RSC.
-
(a) S. Erten-Ela, M. DenizYilmaz, B. Icli, Y. Dede, S. Icli and E. U. Akkaya, Org. Lett., 2008, 15, 3299 CrossRef PubMed;
(b) J. Liao, H. Zhao, Y. Xu, W. Zhou, F. Peng, Y. Wanga and Y. Fang, RSC Adv., 2017, 7, 33975 RSC.
- S. Ganesan, B. Muthuraaman, J. Madhavan, P. Maruthamuthu and A. Suthanthiraraj, Sol. Energy Mater. Sol. Cells, 2008, 92, 1718 CrossRef CAS.
- E. Zervaki Galateia, N. Agapi, N. Vasilis, D. S. Ganesh and C. G. Athanassios, J. Mater. Chem. C, 2015, 3, 5652 RSC.
- J. Babu, S. Ganesan, M. Karuppusamy and P. Rajakumar, ChemistrySelect, 2018, 3, 9222 CrossRef CAS.
- K. Rajavelua, M. Sudip, R. Kothandaraman and P. Rajakumar, Sol. Energy, 2018, 166, 379 CrossRef.
Footnote |
† Electronic supplementary information (ESI) available. See DOI: 10.1039/d0ra01672a |
|
This journal is © The Royal Society of Chemistry 2020 |