DOI:
10.1039/D0RA01638A
(Paper)
RSC Adv., 2020,
10, 15221-15227
PVP-coated Sb2Se3 nanorods as nanotheranostic agents for photoacoustic imaging and photothermal therapy in NIR-I bio-windows†
Received
20th February 2020
, Accepted 7th April 2020
First published on 17th April 2020
Abstract
Antimony selenide (Sb2Se3) as a simple, low toxicity, low-cost p-type semiconductor material with broad absorbance ranging from the UV to the NIR region has many potential applications in photovoltaic, thermoelectric, and phase-change memory devices. Owing to these excellent properties, Sb2Se3 nanorods were firstly synthesized with triphenylantimony and dibenzyldiselenide under solvothermal conditions. In order to enhance the biocompatibility of the Sb2Se3 nanorods, polyvinylpyrrolidone (PVP) was coated onto the surface of the Sb2Se3 nanorods to form PVP-coated Sb2Se3 nanorods. The cell viability of PVP-coated Sb2Se3 nanorods toward Hep-2 cells was assessed for 24 h using a Cell Counting Kit-8 (CCK-8) assay. The results showed that Hep-2 cells treated with PVP-coated Sb2Se3 nanorods were alive at a concentration as high as 100 μg mL−1 in the absence of NIR irradiation. In vivo assessment confirmed that PVP-coated Sb2Se3 nanorods exhibited excellent photoacoustic imaging and PTT performance, which yielded complete ablation of tumors after laser irradiation (808 nm or 980 nm) in the NIR-I bio-window.
1 Introduction
Semiconductor nanotherapeutics have attracted widespread attention in the treatment of cancer due to their remarkable physicochemical and biochemical properties, such as low band gap, prolonged circulation time and enhanced permeability and retention (EPR) effect.1–5 Currently, diverse types of nanotherapeutics, such as organic dye ICG; inorganic nanoparticles Pd, Ge, Au, black phosphorus QDs, CuS, MoS2, Bi2S3 and WO3−X nanocrystals; organic semiconducting polymer nanoparticles (SPNs) and ppy nanoparticles, have been investigated for NIR PTT.6–20 Antimony selenide (Sb2Se3) as a simple, low toxicity, low-cost p-type semiconductor material with broad absorbance ranging from the UV to the NIR region has many potential applications in photovoltaic, thermoelectric, and phase-change memory devices.21–23 Owing to these excellent properties, much effort has been devoted to the fabrication of Sb2Se3 and its photovoltaic applications, rarely have this material been explored for its biomedical applications. In view of the above consideration, it is highly recommended to develop a novel Sb2Se3 nanotherapeutic agent that can be applied in PTT for cancer cells.
Photothermal therapy (PTT), as a flourishing therapeutic modality, employs light-absorbing agents to generate localized heat upon continuous irradiation of near-infrared (NIR) laser for hyperthermia ablation of cancer cells. In the light of the superiority of PTT in therapeutic modality, such as minimal invasiveness, noninvasive penetration and low toxicity, PTT has received increasing attention in recent years.24–35 Photoacoustic imaging (PAI), which convert incident light into acoustic waves by exciting nanoparticle or chromophore with a strong optical absorbance spectrum in the NIR region, show powerful advantage such as deep tissue penetration, high spatial resolution, high signal-noise ratio in contrast to conventional X-ray computed tomography (CT), positron emission tomography (PET), magnetic resonance imaging (MRI) and fluorescence images (FLI).36–49
In this work, we report a biocompatible PVP-coated Sb2Se3 nanorods as PTT theranostic agents with relatively narrow band gap energy, which are responsive to the light (808 and 980 nm) in the NIR-I bio-windows (Scheme 1). The PTT studies in vitro and in vivo showed that PVP-coated Sb2Se3 nanorods were effective for photoacoustic imaging and photothermal destruction of cancer cell. Therefore, Sb2Se3 is a promising nanotherapeutics agent for PAI and PTT.
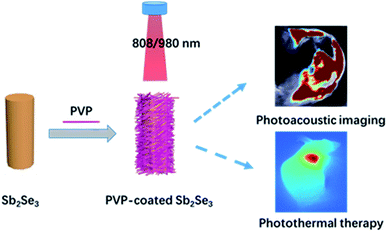 |
| Scheme 1 Schematic illustration of PVP-coated Sb2Se3 nanorods nanotheranostic agent for photoacoustic imaging diagnosis and photothermal cancer treatment in NIR-I bio-windows. | |
2 Experimental section
2.1 Chemicals
Triphenylantimony (97%), dibenzyldiselenide (98%), PVP-K30 were purchased from Sinopharm Chemicals. Cell Counting Kit (CCK-8) and 4′,6-diamidino-2-phenylindole (DAPI) were purchased from Sigma-Aldrich. All solvents were of analytical grade and used without further purification. Deionized water (18 MΩ cm) used in the experiments was produced from a Milli-Q Gradient System.
2.2 Synthesis of PVP-coated Sb2Se3 nanorods
In a typical procedure,50–52 0.10 mmol (105.4 mg) triphenylantimony and 0.15 mmol (68 mg) dibenzyldiselenide were dissolved in 5.0 mL oleylamine. Besides, 0.2 g PVP was dissolved in 10 mL EtOH. Then, two solutions were mixed and stirred, transferred into 25 mL Teflon-lined stainless steel autoclave. The reaction was kept at 200 °C for 16 h. After that, the autoclaves were cooled to room temperature naturally. The resulting product was washed with ethanol several times. To improve the biocompatibility of the Sb2Se3 nanorods, 10 mg of PVP was dissolved in 2 mL of Sb2Se3 nanorods aqueous solution (2 mg mL−1) with stirring for 12 h. After several washes with deionized water, the final product was stored at room temperature for later use.
2.3 CCK-8 assays in laryngeal cancer
Hep-2 cells (≈10
000 cells per well) pressed in a 96-well plate were cultured in fresh media that contained 10% fetal calf serum. The PVP-coated Sb2Se3 nanorods at various concentrations were added and cultivated at 37 °C for 24 h. Then, 10 μL of CCK-8 was added to each well after 24 h. The Hep-2 cells were further incubated for 2 h. The determination was carried at 460 nm with enzymatic marker.
2.4 Cell photothermal performance
The photothermal behavior of PVP-coated Sb2Se3 nanorods against Hep-2 cells was investigated in the presence or absence of NIR irradiation. Hep-2 cells were seeded at a concentration of 1 × 104 cells per well onto a 96-well plate and precultured for 24 h at 37 °C. After incubated with PVP-coated Sb2Se3 nanorods, the cells were exposed to NIR laser (808/980 nm, 1 W cm−2) for 5 min and then stained with calcein AM and PI for 20 min to verify the photothermal behavior for killing cancer cells, and the cells image were obtained using a fluorescence microscope (Olympus, IX73).
2.5 Animal tumor model
The animal procedures were performed in accordance with the Guidelines for Care and Use of Laboratory Animals of Shanxi Medical University, and the experiments were approved by the Animal Ethics Committee of Shanxi Medical University. The six-week-old female nude mice (16–18 g) were purchased from Beijing Vital River Experimental Animal Company. After 2 weeks of acclimatization, tumor models were established by subcutaneous injection of 1 × 106 Hep-2 cells into the RL region of the nude mice. When the tumor volumes reached 100 mm3, the mice were used for imaging and treatment in vivo.
2.6 In vivo PAI study
For in vivo PAI, the tumor-bearing mice were injected with PVP-coated Sb2Se3 nanorods via intratumoral administration and then monitored by a real-time MSOT imaging system with a laser wavelength of 730 nm at 1, 2, 4, 6 and 12 h post injection.
2.7 In vivo PTT study
To investigate the PTT effect of PVP-coated Sb2Se3 nanorods in vivo, Hep-2 tumor-bearing mice were divided into four groups and given following treatments: (I), laser (808 nm); (II), PVP-coated Sb2Se3 nanorods + laser (808 nm); (III), laser (980 nm); (IV), PVP-coated Sb2Se3 nanorods + laser (980 nm). After 2 h intratumoral injection, the mice were irradiated with NIR laser for 5 min. Then an infrared thermal camera (Fluke Ti400) was applied to record the temperature of tumor site. After treatment, the tumor volume and body weight of each mice were inspected in the following 14 days.
2.8 Characterization
The morphology and size of PVP-coated Sb2Se3 nanorods were characterized by a TEM (JEOL-2100F) operated at 200 kV. UV-Vis-NIR absorption spectra were acquired by a UV-2550 spectrophotometer. The Fourier transform infrared (FTIR) spectrum was measured by a Vector 22 spectrometer with KBr pellets (Bruker AXS, Inc., Madison, WI, USA). Thermogravimetric analyses (TGA) were performed on a simultaneous SDT 2960 thermal analyzer from 30 to 600 °C with a heating rate of 20 °C min−1 under N2 atmosphere. Zeta potential were measured by a Nano-Zetasizer (Malvern Instruments Ltd.).
3 Results and discussion
Sb2Se3 nanorods were prepared using a previously reported method with slight modification. PVP was coated on the surface of Sb2Se3 nanorods in order to enhance the biocompatibility of Sb2Se3 nanorods. The successful coating of the Sb2Se3 nanorods can be verified by the TGA (Fig. 1C) and FT-IR spectra (Fig. S1†). Thermogravimetric analysis revealed that the mass fraction of PVP in the as-prepared PVP-coated Sb2Se3 nanorods is 7%.53 The characteristic absorption band at 1660 cm−1 belongs to the C
O stretching vibration mode, suggesting that the coating of PVP on the surface Sb2Se3.54 Due to the low loading capacity, the FT-IR spectra of PVP-coated Sb2Se3 nanorods show weak absorbance intensity. Transmission electron microscopy (TEM) images of Sb2Se3 and PVP-coated Sb2Se3 nanorods were shown in Fig. 1A. All the PVP-coated Sb2Se3 show a nanorod morphology with average size of 500 nm. The composition of the core–shell nanostructures was more evident from the EDX elemental mapping data. Evidently, the elements Sb and Se is mainly distributed in the core and the elements C and N are homogenously distributed throughout the whole nanostructures, indicating that the Sb2Se3 core is surrounded with a uniform and continuous PVP shell. The ζ potentials of Sb2Se3 and PVP-coated Sb2Se3 nanorods are −20 mV and +2.5 mV, respectively (Fig. S2†). All data above indicate that the layer coated on Sb2Se3 nanorods is PVP. UV-Vis spectra were recorded to investigate the effect of Sb2Se3 and PVP-coated Sb2Se3 nanorods. As displayed in Fig. 1B, the Sb2Se3 and PVP-coated Sb2Se3 nanorods show similar absorbance spectra from 400 to 1000 nm. However, the absorbance of PVP-coated Sb2Se3 nanorods is much higher than Sb2Se3 in the range of 400 to 1000 nm, which encourage us to further explore the PA performance of PVP-coated Sb2Se3 nanorods. To evaluate photoacoustic behavior of PVP-coated Sb2Se3 nanorods, the photoacoustic signals of PVP-coated Sb2Se3 nanorods aqueous dispersions with concentrations from 0.2 to 1.0 mg mL−1 were determined with water. As shown in Fig. 4C, PVP-coated Sb2Se3 nanorods aqueous solution showed concentration-dependent PA signals under 808 nm light excitation due to their thermoelastic expansion in aqueous solution, indicating that PVP-coated Sb2Se3 nanorods might act as a PA contrast agent.
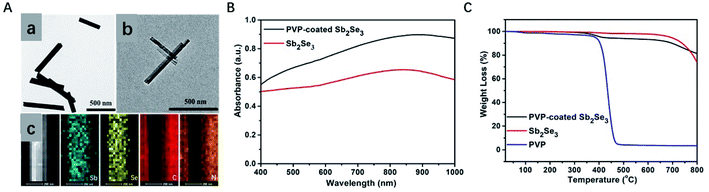 |
| Fig. 1 TEM images of Sb2Se3 (A-a) and PVP-coated Sb2Se3 nanorods (A-b) and (A-c) EDX elemental mapping images of PVP-coated Sb2Se3 nanorods. (B) UV-vis-NIR absorption spectra of Sb2Se3 and PVP-coated Sb2Se3 nanorods aqueous solution. (C) TGA curves of PVP, Sb2Se3 and PVP-coated Sb2Se3 nanorods. | |
In order to investigate the photothermal properties of the obtained PVP-coated Sb2Se3 nanorods, PVP-coated Sb2Se3 nanorods with different concentrations (0, 0.125, 0.25, 0.5 and 1.0 mg mL−1) were irradiated with an 808 nm laser for 300 s. The temperature elevation of PVP-coated Sb2Se3 nanorods aqueous dispersions shows an obvious concentration-dependent temperature increase in response to NIR irradiation (808 nm, 1.5 W cm−2) (Fig. 2A), especially for that with a higher PVP-coated Sb2Se3 nanorods concentration and extended irradiation duration. For example, the temperature of PVP-coated Sb2Se3 nanorods (0.25 mg mL−1) raised up to 55 °C within 5 min which can kill cancer cells effectively, while the control group of phosphate buffered saline (PBS) only showed a mild temperature elevation (from 12.2 to 20.0 °C). The color change in the photothermal images was clearly observed (Fig. 2B), which was measured using an infrared thermal camera with the PVP-coated Sb2Se3 nanorods solution (0.125, 0.25, 0.5, 1.0 and 2.0 mg mL−1) under 808 nm light exposure at 1.0 W cm−2 for 5 min and PBS was selected as the control. It is clearly seen that the photothermal effect of PVP-coated Sb2Se3 nanorods was proportionally improved as the concentrations increased. At the same concentration of 0.25 mg mL−1, the temperature increase of the PVP-coated Sb2Se3 nanorods solution may be controlled under continuous radiation of an 808 nm laser, as shown in Fig. 2C. It also confirmed that the photothermal effect of the PVP-coated Sb2Se3 nanorods solution was complying with a laser power density-dependent mode.
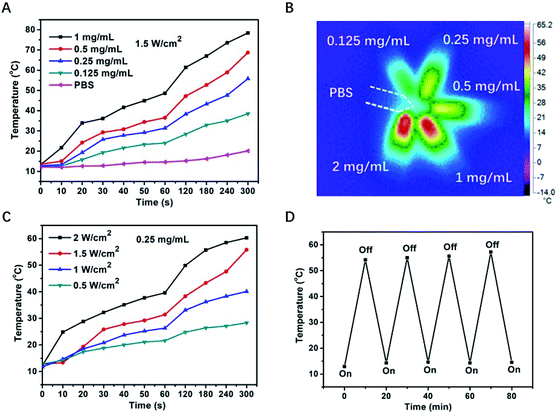 |
| Fig. 2 (A) Temperature changes of PVP-coated Sb2Se3 nanorods at different concentrations exposed to 808 nm laser at a power density of 1.5 W cm−2. (B) Infrared thermal images of the solution containing PVP-coated Sb2Se3 nanorods under the NIR laser (808 nm, 1.0 W cm−2) irradiation for 5 min. (C) Temperature change of PVP-coated Sb2Se3 nanorods at a constant concentration with different power. (D) Temperature variation of PVP-coated Sb2Se3 nanorods aqueous solution under 808 nm laser irradiation (1.0 W cm−2) for four laser on/off cycles. | |
To evaluate their photostability, four cycles of light irradiation was carried out (Fig. 2D). PVP-coated Sb2Se3 nanorods dispersions (0.5 mg mL−1) was irradiated with 808 nm laser at 1 W cm−2 for 10 min, and the irradiation was tuned off for leaving the photothermal temperature to room temperature. The same switch on/off was repeated for another three times. The results exhibited that PVP-coated Sb2Se3 nanorods showed the temperature increase of 54.2 °C after the first irradiation, and there was no obvious change in temperature variation after three switch on/off cycles, suggesting that PVP-coated Sb2Se3 nanorods dispersions possess excellent photostability under repeated irradiation. The photothermal and photostability behavior of PVP-coated Sb2Se3 nanorods upon 980 nm laser irradiation was also investigated, similar outcome was shown in Fig. S3,† which attributed to the NIR absorption.
In view of above results, NIR-mediated PVP-coated Sb2Se3 nanorods displaying photothermal effect urge us to examine phototherapeutic effect toward Hep-2 cells in vitro. Prior to in vitro photothermal therapy, the biosafety evaluation illustrated in Fig. 3A is vitally important for the next task. Therefore, the cell viability of PVP-coated Sb2Se3 nanorods toward Hep-2 cells was assessed for 24 h by Cell Counting Kit-8 (CCK-8) assay. The result showed that Hep-2 cells treated with PVP-coated Sb2Se3 nanorods were alive at a concentration as high as 100 μg mL−1 in the absence of NIR irradiation. Furthermore, a live/dead cell assay was performed using calcein acetoxymethyl (calcein AM, green) and propidium iodide (PI, red) staining after NIR light irradiation at 808 nm for 5 min. Under NIR light irradiation, almost all the cells induced by PVP-coated Sb2Se3 nanorods to death emitted red fluorescence. In contrast, there was green fluorescence observed in the absence of laser irradiation, which implied that cells were living (Fig. 3B and S4†). In a word, these results indicate that PVP-coated Sb2Se3 nanorods have promising potential as a photothermal agent to kill cancer cells. We further investigate photothermal effect and live/dead cell assay of PVP-coated Sb2Se3 nanorods under 980 nm laser irradiation, as shown in Fig. S5.† All results confirmed that PVP-coated Sb2Se3 nanorods show promising photothermal ability for killing cancer cells in the NIR-I bio-windows.
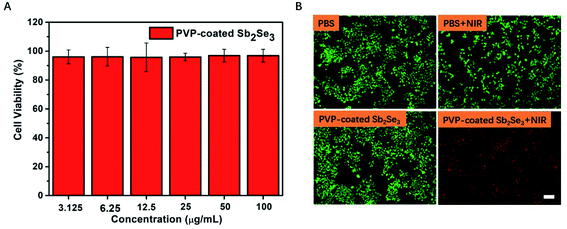 |
| Fig. 3 (A) Cell viability of Hep-2 cells after cultured with various concentrations of PVP-coated Sb2Se3 nanorods for 24 h. (B) Fluorescence images of live (green) and dead (red) cells stained by calcein AM and PI after being treated with PBS, PBS + NIR (808 nm), PVP-coated Sb2Se3 nanorods, and PVP-coated Sb2Se3 nanorods + NIR (808 nm). Scale bar: 100 μm. | |
Encouraged by the photoacoustic and photothermal properties in vitro, we continued to evaluate the potential of PVP-coated Sb2Se3 nanorods as photoacoustic imaging and thermal imaging agent. The PA imaging was investigated in vivo. As shown in Fig. 4A, mice bearing Hep-2 tumors were injected with PVP-coated Sb2Se3 nanorods. PA signals in tumor was gradually enhanced over time and reached their maximum 2 h after injection, indicating that the optimal treatment time was 2 h after i.t. injection (Fig. 4B). Furthermore, the IR thermal imaging was applied to detect the real-time temperature of tumor region at 2 h post injection. For mice treated with PVP-coated Sb2Se3 nanorods, tumor temperature gradually increased to 60 °C within 5 min when exposed to 808 nm laser, while the temperature of control group displayed little change (Fig. 5A and B). In comparison, the tumor temperature reaches 57.5 °C exposed to 980 nm laser under the same condition, both of which could absolutely meet to the requirement and efficacy of PTT (Fig. S6A and B†). Thus, the PVP-coated Sb2Se3 nanorods could be utilized as photoacoustic and photothermal contrast agent.
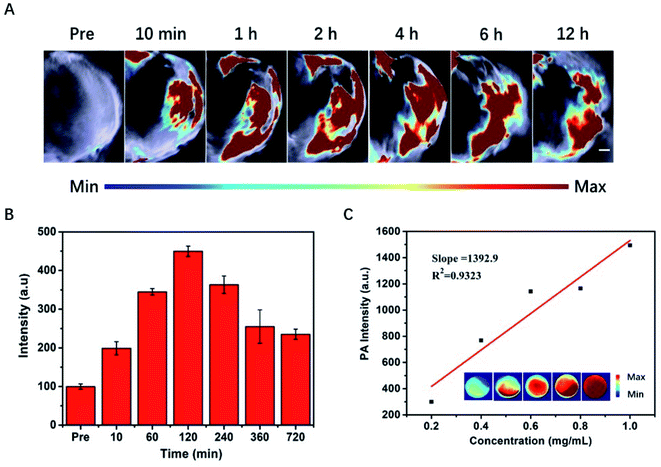 |
| Fig. 4 (A) PA images of tumors at different time points after injection of PVP-coated Sb2Se3 nanorods. (B) Quantitative analysis of PA values in (A). (C) Linear relationship between concentrations and PA signals, with corresponding PA images in the inset. | |
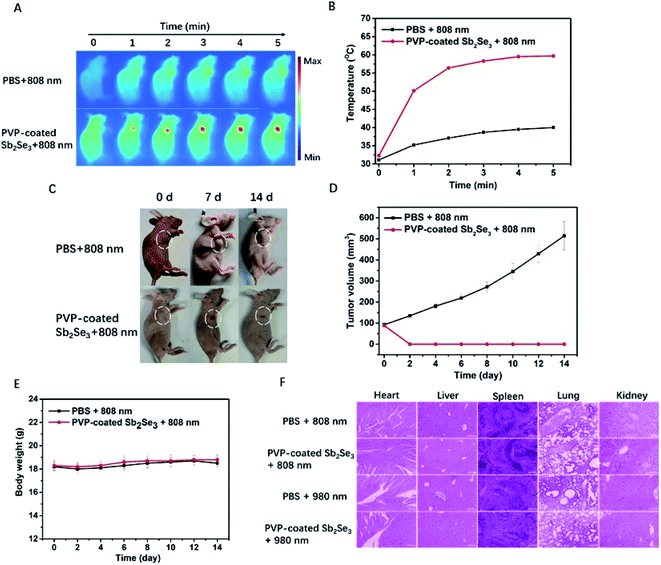 |
| Fig. 5 (A) IR thermal images of Hep-2 tumor bearing nude mice. (B) Time-dependent temperature change in mice tumor after different treatments. (C) Representative photographs of mice tumor after various treatment 0–14 day. (D) Tumor growth curves of Hep-2 tumor bearing nude mice after treatment for 14 days. (E) Body weights of mice during different treatments. (F) H&E staining images of major organs (heart, liver, spleen, lung, kidney). Scale bar: 100 μm. | |
In view of above good PAI and PTT, upon NIR light irradiation, we further evaluated the potential application of PVP-coated Sb2Se3 nanorods for cancer therapy using the Hep-2 tumor bearing nude mice. At 2 h postinjection, the 808 nm or 980 nm NIR laser was applied to irradiate the tumor site. After 14 d of treatment, the tumor was completely eliminated in the PVP-coated Sb2Se3 nanorods plus laser and increased in PBS plus laser (Fig. 5C and S6C†), which is in accordance with the tumor volume change (Fig. 5D and S6D†). More importantly, the body weight of the mice showed no apparent change during treatment (Fig. 5E and S6E†). Due to complete ablation of tumor after treatment, the hematoxylin and eosin (H&E) staining of tumor slides have not been shown. Meanwhile, there were no evident organs damage observed in the slices collected from major organs of control group and PVP-coated Sb2Se3 nanorods plus laser, indicating the negligible toxicity of PVP-coated Sb2Se3 nanorods in vivo (Fig. 5F). The in vivo photothermal therapy by i.t. injection further verified the efficient cancer therapy ability of PVP-coated Sb2Se3 nanorods.
4 Conclusions
In summary, this work firstly presented the biomedicine application of PVP-coated Sb2Se3 nanorods as photothermal nanotherapeutics under either 808 nm or 980 nm in fighting against cancer cell. Due to the strong NIR absorption capability in the NIR-I bio-windows (750–1000 nm),55 the PVP-coated Sb2Se3 nanorods display preferable photoacoustic and photothermal behavior. In vivo assessment confirmed that PVP-coated Sb2Se3 nanorods effectively induced PTT properties, which yielded complete ablation of tumor after laser irradiation (808 nm or 980 nm) in the NIR-I bio-windows. This work inspires us to develop much more photovoltaic semiconductor materials with low toxicity and expands their potential application in biomedical field for cancer treatment.
Conflicts of interest
There are no conflicts to declare.
Acknowledgements
This work has been financially supported by the National Natural Science Foundation of China (No. 81571747, 81771907), Science and technology innovation team project of Shanxi Province (No. 201705D131026), Engineering Technology Research Center of Shanxi Province (No. 201805D121008) Scientific and technological achievements transformation project of Shanxi Province (No. 201704D131006), Laboratory Construction Project of Shanxi Province, The Projects for Local Science and Technology Development Guided by the Central Committee (YDZX20191400002537), Natural Science Foundation of Shanxi Province (No. 201901D111213) and Startup Foundation for Doctors of Shanxi Medical University (No. 03201505).
Notes and references
- S. Wang, A. Riedinger, H. Li, C. Fu, H. Liu, L. Li, T. Liu, L. Tan, M. J. Barthel, G. Pugliese, F. De Donato, M. Scotto D'Abbusco, X. Meng, L. Manna, H. Meng and T. Pellegrino, ACS Nano, 2015, 9, 1788–1800 CrossRef CAS PubMed.
- B. Godin, E. Tasciotti, X. Liu, R. E. Serda and M. Ferrari, Acc. Chem. Res., 2011, 44, 979–989 CrossRef CAS PubMed.
- X. Zhen, C. Xie and K. Pu, Angew. Chem., 2018, 57, 3938–3942 CrossRef CAS PubMed.
- W. Li, P. Rong, K. Yang, P. Huang, K. Sun and X. Chen, Biomaterials, 2015, 45, 18–26 CrossRef CAS PubMed.
- W. Huang, Y. Huang, Y. You, T. Nie and T. Chen, Adv. Funct. Mater., 2017, 27, 1701388 CrossRef.
- M. Zhu, Z. Sheng, Y. Jia, D. Hu, X. Liu, X. Xia, C. Liu, P. Wang, X. Wang and H. Zheng, ACS Appl. Mater. Interfaces, 2017, 9, 39249–39258 CrossRef CAS PubMed.
- C. Zhang, L. Zhou, J. Zhang, Y. Y. Fu, X. Zhang, C. Yu, S. K. Sun and X. P. Yan, Nanoscale, 2016, 8, 16204–16211 RSC.
- Q. Chen, L. Xu, C. Liang, C. Wang, R. Peng and Z. Liu, Nat. Commun., 2016, 7, 13193 CrossRef CAS PubMed.
- P. Zhao, Z. Jin, Q. Chen, T. Yang, D. Chen, J. Meng, X. Lu, Z. Gu and Q. He, Nat. Commun., 2018, 9, 4241 CrossRef PubMed.
- W. Sun, G. Zhong, C. Kübel, A. A. Jelle, C. Qian, L. Wang, M. Ebrahimi, L. M. Reyes, A. S. Helmy and G. A. Ozin, Angew. Chem., Int. Ed., 2017, 56, 6329–6334 CrossRef CAS PubMed.
- W. Shang, C. Zeng, Y. Du, H. Hui, X. Liang, C. Chi, K. Wang, Z. Wang and J. Tian, Adv. Mater., 2017, 29, 1604381 CrossRef PubMed.
- X. Zhao, C.-X. Yang, L.-G. Chen and X.-P. Yan, Nat. Commun., 2017, 8, 14998 CrossRef CAS PubMed.
- Z. Sun, H. Xie, S. Tang, X. F. Yu, Z. Guo, J. Shao, H. Zhang, H. Huang, H. Wang and P. K. Chu, Angew. Chem., 2015, 54, 11526–11530 CrossRef CAS PubMed.
- Q. Tian, M. Tang, Y. Sun, R. Zou, Z. Chen, M. Zhu, S. Yang, J. Wang, J. Wang and J. Hu, Adv. Mater., 2011, 23, 3542–3547 CrossRef CAS PubMed.
- R. Marin, A. Skripka, L. V. Besteiro, A. Benayas, Z. Wang, A. O. Govorov, P. Canton and F. Vetrone, Small, 2018, 1803282 CrossRef PubMed.
- Q. Gao, X. Zhang, W. Yin, D. Ma, C. Xie, L. Zheng, X. Dong, L. Mei, J. Yu, C. Wang, Z. Gu and Y. Zhao, Small, 2018, 14, 1802290 CrossRef PubMed.
- Z. Xiao, C. Xu, X. Jiang, W. Zhang, Y. Peng, R. Zou, X. Huang, Q. Liu, Z. Qin and J. Hu, Nano Res., 2016, 9, 1934–1947 CrossRef CAS.
- K. Deng, Z. Hou, X. Deng, P. Yang, C. Li and J. Lin, Adv. Funct. Mater., 2015, 25, 7280–7290 CrossRef CAS.
- Y. Jiang, P. K. Upputuri, C. Xie, Y. Lyu, L. Zhang, Q. Xiong, M. Pramanik and K. Pu, Nano Lett., 2017, 17, 4964–4969 CrossRef CAS PubMed.
- X. Wang, Y. Ma, X. Sheng, Y. Wang and H. Xu, Nano Lett., 2018, 18, 2217–2225 CrossRef CAS PubMed.
- Y. Zhou, L. Wang, S. Chen, S. Qin, X. Liu, J. Chen, D.-J. Xue, M. Luo, Y. Cao, Y. Cheng, E. H. Sargent and J. Tang, Nat. Photonics, 2015, 9, 409 CrossRef CAS.
- M. S. Dresselhaus, G. Chen, M. Y. Tang, R. G. Yang, H. Lee, D. Z. Wang, Z. F. Ren, J. P. Fleurial and P. Gogna, Adv. Mater., 2007, 19, 1043–1053 CrossRef CAS.
- C. Karthik, R. J. Mehta, W. Jiang, E. Castillo, T. Borca-Tasciuc and G. Ramanath, Appl. Phys. Lett., 2011, 99, 103101 CrossRef.
- S. Liang, Z. Xie, Y. Wei, Z. Cheng, Y. Han and J. Lin, Dalton Trans., 2018, 47, 7916–7924 RSC.
- R. Zhang, K. Cheng, A. L. Antaris, X. Ma, M. Yang, S. Ramakrishnan, G. Liu, A. Lu, H. Dai, M. Tian and Z. Cheng, Biomaterials, 2016, 103, 265–277 CrossRef CAS PubMed.
- W. Wang, L. Wang, Y. Li, S. Liu, Z. Xie and X. Jing, Adv. Mater., 2016, 28, 9320–9325 CrossRef CAS PubMed.
- Z. Wang, Z. Chang, M. Lu, D. Shao, J. Yue, D. Yang, M. Li and W. F. Dong, ACS Appl. Mater. Interfaces, 2017, 9, 30306–30317 CrossRef CAS PubMed.
- T. T. Zhang, C. H. Xu, W. Zhao, Y. Gu, X. L. Li, J. J. Xu and H. Y. Chen, Chem. Sci., 2018, 9, 6749–6757 RSC.
- Z. Li, Y. Hu, Z. Miao, H. Xu, C. Li, Y. Zhao, Z. Li, M. Chang, Z. Ma, Y. Sun, F. Besenbacher, P. Huang and M. Yu, Nano Lett., 2018, 18, 6778–6788 CrossRef CAS PubMed.
- K. Zhang, X. Meng, Y. Cao, Z. Yang, H. Dong, Y. Zhang, H. Lu, Z. Shi and X. Zhang, Adv. Funct. Mater., 2018, 28, 1804634 CrossRef.
- L. Song, X. Dong, S. Zhu, C. Zhang, W. Yin, X. Zhang, X. Liu and Z. Gu, Adv. Healthcare Mater., 2018, 7, 1800830 CrossRef PubMed.
- X. Ji, N. Kong, J. Wang, W. Li, Y. Xiao, S. T. Gan, Y. Zhang, Y. Li, X. Song, Q. Xiong, S. Shi, Z. Li, W. Tao, H. Zhang, L. Mei and J. Shi, Adv. Mater., 2018, 30, 1803031 CrossRef PubMed.
- J.-Y. Zeng, M.-K. Zhang, M.-Y. Peng, D. Gong and X.-Z. Zhang, Adv. Funct. Mater., 2017, 28, 1705451 CrossRef.
- S. Wang, X. Ma, X. Hong, Y. Cheng, Y. Tian, S. Zhao, W. Liu, Y. Tang, R. Zhao, L. Song, Z. Teng and G. Lu, ACS Nano, 2018, 12, 662–670 CrossRef CAS PubMed.
- S. Goel, C. A. Ferreira, F. Chen, P. A. Ellison, C. M. Siamof, T. E. Barnhart and W. Cai, Adv. Mater., 2018, 30, 1704367 CrossRef PubMed.
- R. Zhang, Q. Fan, M. Yang, K. Cheng, X. Lu, L. Zhang, W. Huang and Z. Cheng, Adv. Mater., 2015, 27, 5063–5069 CrossRef CAS PubMed.
- Q. Fan, K. Cheng, X. Hu, X. Ma, R. Zhang, M. Yang, X. Lu, L. Xing, W. Huang, S. S. Gambhir and Z. Cheng, J. Am. Chem. Soc., 2014, 136, 15185–15194 CrossRef CAS PubMed.
- M. Yang, Q. Fan, R. Zhang, K. Cheng, J. Yan, D. Pan, X. Ma, A. Lu and Z. Cheng, Biomaterials, 2015, 69, 30–37 CrossRef CAS PubMed.
- J. Sun, W. Xu, L. Li, B. Fan, X. Peng, B. Qu, L. Wang, T. Li, S. Li and R. Zhang, Nanoscale, 2018, 10, 10584–10595 RSC.
- X. Jiang, S. Zhang, F. Ren, L. Chen, J. Zeng, M. Zhu, Z. Cheng, M. Gao and Z. Li, ACS Nano, 2017, 11, 5633–5645 CrossRef CAS PubMed.
- P. Si, E. Yuan, O. Liba, Y. Winetraub, S. Yousefi, E. D. SoRelle, D. W. Yecies, R. Dutta and A. de la Zerda, ACS Nano, 2018, 12, 11986–11994 CrossRef CAS PubMed.
- Q. Zou, J. Huang and X. Zhang, Small, 2018, 14, 1803101 CrossRef PubMed.
- P. Garrigue, J. Tang, L. Ding, A. Bouhlel, A. Tintaru, E. Laurini, Y. Huang, Z. Lyu, M. Zhang, S. Fernandez, L. Balasse, W. Lan, E. Mas, D. Marson, Y. Weng, X. Liu, S. Giorgio, J. Iovanna, S. Pricl, B. Guillet and L. Peng, Proc. Natl. Acad. Sci. U. S. A., 2018, 115, 11454–11459 CrossRef CAS PubMed.
- Q. Jin, W. Zhu, D. Jiang, R. Zhang, C. J. Kutyreff, J. W. Engle, P. Huang, W. Cai, Z. Liu and L. Cheng, Nanoscale, 2017, 9, 12609–12617 RSC.
- J. C. Yang, Y. Chen, Y. H. Li and X. B. Yin, ACS Appl. Mater. Interfaces, 2017, 9, 22278–22288 CrossRef CAS PubMed.
- Z. Shen, T. Liu, Y. Li, J. Lau, Z. Yang, W. Fan, Z. Zhou, C. Shi, C. Ke, V. I. Bregadze, S. K. Mandal, Y. Liu, Z. Li, T. Xue, G. Zhu, J. Munasinghe, G. Niu, A. Wu and X. Chen, ACS Nano, 2018, 12, 11355–11365 CrossRef CAS PubMed.
- H. Wan, H. Ma, S. Zhu, F. Wang, Y. Tian, R. Ma, Q. Yang, Z. Hu, T. Zhu, W. Wang, Z. Ma, M. Zhang, Y. Zhong, H. Sun, Y. Liang and H. Dai, Adv. Funct. Mater., 2018, 1804956 CrossRef PubMed.
- J. S. Ni, P. Zhang, T. Jiang, Y. Chen, H. Su, D. Wang, Z. Q. Yu, R. T. K. Kwok, Z. Zhao, J. W. Y. Lam and B. Z. Tang, Adv. Mater., 2018, 1805220 CrossRef PubMed.
- X. Hao, C. Li, Y. Zhang, H. Wang, G. Chen, M. Wang and Q. Wang, Adv. Mater., 2018, 1804437 CrossRef.
- G. Chen, J. Zhou, J. Zuo and Q. Yang, ACS Appl. Mater. Interfaces, 2016, 8, 2819–2825 CrossRef CAS PubMed.
- R. J. Mehta, C. Karthik, W. Jiang, B. Singh, Y. Shi, R. W. Siegel, T. Borca-Tasciuc and G. Ramanath, Nano Lett., 2010, 10, 4417–4422 CrossRef CAS PubMed.
- J. Ma, Y. Wang, Y. Wang, P. Peng, J. Lian, X. Duan, Z. Liu, X. Liu, Q. Chen, T. Kim, G. Yao and W. Zheng, CrystEngComm, 2011, 13, 2369–2374 RSC.
- P. Lei, R. An, P. Zhang, S. Yao, S. Song, L. Dong, X. Xu, K. Du, J. Feng and H. Zhang, Adv. Funct. Mater., 2017, 27, 1702018 CrossRef.
- J. Wei, J. Li, D. Sun, Q. Li, J. Ma, X. Chen, X. Zhu and N. Zheng, Adv. Funct. Mater., 2018, 28, 1706310 CrossRef.
- H. Lin, S. Gao, C. Dai, Y. Chen and J. Shi, J. Am. Chem. Soc., 2017, 139, 16235–16247 CrossRef CAS PubMed.
Footnotes |
† Electronic supplementary information (ESI) available. See DOI: 10.1039/d0ra01638a |
‡ The first two authors contributed equally to this work. |
|
This journal is © The Royal Society of Chemistry 2020 |
Click here to see how this site uses Cookies. View our privacy policy here.