DOI:
10.1039/D0RA01522A
(Paper)
RSC Adv., 2020,
10, 16266-16276
Ruthenium carboranyl complexes with 2,2′-bipyridine derivatives for potential bimodal therapy application†
Received
17th February 2020
, Accepted 6th April 2020
First published on 23rd April 2020
Abstract
Ruthenium complexes of carboranyl ligands offer the possibility of dual action (chemo + radiotherapy) that might result in significant clinical benefits. In that frame, we describe herein the development of ruthenium–carboranyl complexes bearing bipyridyl derivatives with the general formula [3-CO-3,3-{κ2-4,4′-R2-2,2′-bipy}-closo-3,1,2-RuC2B9H11] (R = CH3, RuCB1 or R = CH2OH, RuCB2). Both compounds crystallized in the monoclinic system, showing the expected three-legged piano stool structure. The ruthenacarboranes are stable in cell culture media and were tested against two cell lines that have shown favorable clinical responses with BNCT, namely melanoma (A375) and glioblastoma (U87). RuCB1 shows no cytotoxic activity up to 100 μM while RuCB2 showed moderate activity for both cell lines. Cell distribution assays showed that RuCB2 presents high boron internalization that is proportional to the concentration used indicating that RuCB2 presents features to be further studied as a potential anticancer bimodal agent (chemo + radiotherapy).
Introduction
Cancer is the second leading cause of death worldwide. Most of the FDA approved anticancer drugs are purely organic molecules, which may include nitrogen, oxygen, and halogens besides carbon and hydrogen, all of them right-hand neighbors of carbon. The successful introduction of cisplatin as an anticancer drug opened the possibility to investigate the potential applications of metal-based compounds in medicine. Many organometallic complexes of Fe(II), Rh(III), Ir(III), Ru(II) and Os(II) have been shown to present modes of action different to cisplatin and related platinum drugs, for which DNA is the main target.1 Although ruthenium-based organometallic compounds have emerged as promising anticancer drugs due to their unique and versatile biochemical properties only a few of them have been reported comprehensively.2
Boron is located on the left side of the carbon in the Periodic Table and both are elements that have the property to build molecules of unlimited size by covalent self-bonding. Essentially, the twelve-vertex C2B10H12 carborane, ortho-, meta- or para-isomers, rank among the most chemical and biological stable molecular compounds known, which display many particular characteristics that do not find a parallel in their organic counterparts.3 These species have two moderately acidic C–H vertices that are easily deprotonated with strong bases and functionalized using electrophiles.4 On the other hand, the anionic nido-carborane ([C2B9H12]− and [C2B9H11]2−) derivatives result from the known as “partial deboronation process”, which takes place by the attack of a nucleophile5–13 to the corresponding closo-C2B10H12 cluster resulting in the loss of a B vertex. Metallacarboranes, neutral half-sandwich [M(C2B9H11)LL′L′′] (L, L′, L′′ being anionic and/or neutral ligands) and the anionic sandwich metallabisdicarbollides [M(C2B9H11)2]−, are derivatives of boron hydrides that contain carbon and metal atoms into the fragment.3 A large number of metals have been incorporated as cluster vertices in metallacarboranes, keeping the chemical and self-assembly properties of the closo carboranes clusters and incorporating the redox properties of the metal.3
Boron neutron capture therapy (BNCT) is a non-invasive binary cancer treatment modality that is based on the selective accumulation of a 10B-containing agent at the tumor site followed by irradiation with low-energy neutrons producing high-linear energy transfer (LET) alpha particles (4He2+) and recoiling lithium ions (7Li3+).14 These high-energy particles have a short path length (5–9 μm) that is approximately the diameter of a cell. Thus, their action is limited to the cells containing the boron agent, which decreases the damage to normal tissues. However, the success of this therapy is highly dependent on the 10B delivered to the cells and thus several classes of BNCT agents have been developed in the past such as sodium tetraborate (borax), sodium pentaborate, p-boronophenylalanine (BPA), sodium borocaptate (BSH), p-carboxyphenylboronic acid, or sodium decahydrodecaborate.15–18 However, the need for the delivery of high amounts of 10B led to the development of boron clusters (boranes, carboranes and metallacarboranes).19 In dicarba-closo-dodecarboranes, hexacoordinated carbon and boron atoms adopt the regular icosahedral geometry. As discussed above, the two carbon vertices in dicarba-closo-dodecarboranes bear relatively acidic hydrogen atoms, which are readily replaced by metals or organic groups.20,21 Besides, substituents can also be introduced with good control to at least a certain number of boron vertices, making the research in this area very versatile and attractive for BNCT. The icosahedral closo-C2B10H12 carborane22 and metallacarborane23 [M(C2B9H11)2]− clusters are 3D aromatic moieties, possessing high symmetry and stability,24 and generally low cytotoxicity,25,26 being good candidates for BNCT.25,27–29 In this frame, several compositions for potential BNCT applications have been developed,30–35 including high boron-loaded DNA-oligomers,36 periphery-decorated and core-initiated borane polyanionic macromolecules,37 peptide–cobalt bis(dicarbollide) conjugates,38 nucleoside–boron cluster conjugates39,40 and cholesterol–metallacarborane conjugates,41 among others.
Examples of ruthenacarborane complexes for potential BNCT are scarcer and comprise the ‘Ru(η6-arene)’ moiety42–46 bonded to the carborane through a dithiolate motif or the upper pentagonal face of the cluster. In this frame, taking into consideration the high stability and the cytotoxic properties of [Ru(η5-C5H5)(2,2′-bipyridine)(Z)]+ complexes we decided to replace the (η5-C5H5)− ligand by the bioisostere dicarbollide anion. The cytotoxicity of the [Ru(η5-C5H5)(2,2′-bipyridine)(Z)]+ family of compounds can be tuned by the ligand Z; when Z is a phosphane, the compounds are highly cytotoxic, whereas when Z is a CO, the compounds show only moderate to low cytotoxicity.47 Previous studies also showed that [Ru(η5-C5H5)(2,2′-bipyridine)(Z)]+ compounds are well internalized into cancer cells.48–50 Thus, based on previous results, we decided to synthesize two multifunctional compounds with the general formula [3-CO-3,3-{κ2-4,4′-R2-2,2′-bipy}-closo-3,1,2-RuC2B9H11], that would provide multi-modal treatment acting at once as chemotherapeutic and BNCT agents. The potential treatment using bifunctional compound types would allow to reduce the doses to get the same therapeutic effect while diminishing the secondary effects to the patient.
Results and discussion
Synthesis
New mononuclear ruthenium–carboranyl complexes bearing bipyridyl derivatives with the general formula [3-CO-3,3-{κ2-4,4′-R2-2,2′-bipy}-closo-3,1,2-RuC2B9H11] were obtained by treatment of the parental tricarbonyl complex [3,3,3-(CO)3-closo-3,1,2-RuC2B9H11] with trimethylamine N-oxide (Me3NO, TMAO) following a reported procedure,51 and the corresponding bipyridyl ligand (Scheme 1, RuCB1, R = –CH3; RuCB2, R = –CH2OH). Sigma coordination of each bidentate chelator to the ruthenium core was achieved in comparable yield to previously reported closo-ruthenacarboranes bearing bipyridine based ligands.51
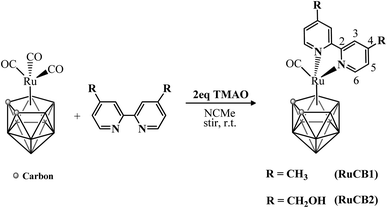 |
| Scheme 1 Synthetic route of the new ruthenacarborane complexes RuCB1 and RuCB2; the 2,2′-bipyridine ligand is numbered for NMR assignments. TMAO = trimethylamine N-oxide. | |
Purification of the new closo-ruthenacarborane complexes was achieved by column chromatography on silica gel and single crystals of RuCB1 and RuCB2 were successfully obtained by slow diffusion recrystallization, at room temperature.
The formulation and purity of the new complexes was fully elucidated, both in the solid-state and in solution, by FT-IR, UV-Vis and NMR (1H, 11B, 13C nuclei) spectroscopies, cyclic voltammetry and single-crystal X-ray diffraction.
NMR spectroscopy
Tables S1 and S2 at the ESI† summarize the 1H NMR and 11B{1H} NMR data for RuCB1 and RuCB2. All resonances were attributed using 1D and 2D NMR experiments (1H, 11B, 13C{1H}, 1H–1H COSY, HMQC, and HMBC) following the atom numbering presented in Scheme 1. The newly synthesized closo-ruthenacarborane complexes displayed resonances in their 1H NMR spectra that were easily ascribed to the two CH cage protons due to their broad character. These signals appeared at δ 3.26 ppm and δ 3.30 ppm for RuCB1 and RuCB2, respectively, and revealed an integration ratio of 1
:
1 between the carboranyl ligand and the respective 2,2′-bipyridyl ligand. The bipyridyl aromatic protons resonate at higher chemical shift (downfield) values when compared to the corresponding resonances of the free ligand (RuCB1: ΔH6 +0.49 ppm, ΔH5 +0.34 ppm and ΔH3 +0.26 ppm; RuCB2, ΔH6 +0.49 ppm, ΔH5 +0.31 ppm and ΔH3 +0.14 ppm; see Table S1†) which agrees with a σ dative coordination to the metal. Additionally, a resonance at δ 2.62 ppm appears in the 1H NMR of complex RuCB1 and it is readily attributed to the equivalent protons of the methyl groups of the coordinated 4,4′-dimethyl-2,2′-bipyridyl ligand, while the two geminal protons of the groups of the 4,4′-dihydroxymethyl-2,2′-bipyridyl ligand of complex RuCB2 resonate at δ 4.96 ppm. Characterization of these complexes was also performed by 13C-APT NMR experiments and the results are in accordance with the previously discussed effects in the 1H NMR analysis. In the 13C-APT NMR spectra of all complexes, a broad singlet resonance appears at δ ≈ 45 ppm and by their position and shape, were easily attributed to the two equivalent carbons present at the carboranyl structure. The resonances for the carbonyl co-ligand appear at δ 199 ppm along with the expected remaining carbons of the 2,2′-bipyridyl ligand (121 < δ < 156 ppm).
RuCB1 and RuCB2 displayed at its 11B{1H} NMR spectra, a general pattern of four peaks with an integration intensity ratio of 1
:
3
:
2
:
3 (Fig. S4;† spectra from RuCB1), being the second and fourth signals result of overlap of broad unresolved 1 + 2 resonances, similarly to results reported for [3-CO-3,3-{κ2-Me2N(CH2)2NMe2}-closo-3,1,2-RuC2B9H11].51 The appearance of the aforementioned resonances agrees with the typical chemical shift range for the closo-3,1,2-MC2B9H11 system (M = Rh, Ru) (−0.9 ppm < δ < −22.3 ppm).52–55 Furthermore, the weighted average 11B NMR chemical shift, 〈δ(11B)〉, is −11.1 and −12.4 ppm for RuCB1 and RuCB2, respectively that were higher than −10 ppm and agree with a closo metallacarborane cluster.56–58 In addition, the 1JHB higher than 100 Hz were found in the 11B NMR spectra giving a clear indication that all B–H protons from the C2B9H11 cage remain intact.
The sensitivity of the electron distribution in closo icosahedral carborane/metallacarborane derivatives due to the presence of substituents at the vertexes is well known.59,60 The averaged chemical shift values move upfield (Table S1†) when the ligand is bipyridyl indicating shielding of the cluster in the closo ruthenacarboranes RuCB1 and RuCB2 relatively to its precursor [3,3,3-(CO)3-closo-3,1,2-RuC2B9H11] (〈δ〉 −7.7 ppm). This result correlates well with the CHcage resonances in the 1H NMR that are shifted upfield in the closo ruthenacarboranes RuCB1 and RuCB2 relatively to the tricarbonyl starting complex (δ 4.15 ppm).
The general shielding effect observed for the cage and the carbonyl nuclei resonances combined with the confirmed deshielding of the 2,2′-bipyridyl protons gives clear evidence of electron flow from the 2,2′-bipyridyl through the ruthenium center towards the boron cage and the carbonyl co-ligand. The presence of a more donating group at the 2,2′-bipyridine substituent improves the electronic flow to the carboranyl moiety.
FT-IR spectroscopy
The FT-IR spectra of the closo-ruthenacarborane complexes presented the characteristic bands of the carboranyl moiety (νC–H,stretching ∼ 3040 cm−1 and νB–H,stretching ∼ 2550 cm−1), the bipyridyl derivatives ligands (ca. 1520–1400 cm−1 and at ∼2960 cm−1 for νC–H,stretching) and a single absorption band attributed to the vibrational frequency of the metallic carbonyl (approximately at 1960 cm−1). It is also noticeable the presence of the stretching frequency of the alcohol functional group in complex RuCB2 spectrum (νO–H,stretching ∼ 3310 cm−1). The carbonyl bonded to the metal center is coordinated in an almost linear fashion (confirmed by crystallographic data) and its binding to the ruthenium ion can be explained by two synergetic contributions: σ-donation from the ligand to the metal and π-back donation from the metal to the ligand. This type of interaction between the orbitals of the metal and the ligand led, in both cases, to a negative shift of the νC
O (av. −93 cm−1).
UV-Vis spectroscopy
The optical absorption spectra of the ruthenacarborane complexes were recorded using 1.0 × 10−5 to 1.0 × 10−6 M solutions in dichloromethane and dimethyl sulfoxide (Table S3†). The trend observed in the electronic absorption spectra of both complexes follows the same pattern and Fig. 1 is representative of their behavior. Despite the strong absorption bands characteristic of each bipyridyl derivative and the {Ru(CO)(C2B9H11)} organometallic fragment (appearing below 300 nm), typical electronic spectra of these compounds are characterized by two broad, medium-strength absorption bands appearing in the range of 330 to 530 nm. The solvatochromic effect observed at the medium-strength transitions of complex RuCB2 showed that these bands are blue-shifted with the increase of the polarity of the solvent (368 nm in CH2Cl2 vs. 347 nm in DMSO for the stronger absorption; 453 nm in CH2Cl2 vs. 434 nm in DMSO for the weaker absorption). Based on their position, intensity and similar behavior in related compounds, we can attribute them as charge transfer (presumably ligand to metal charge transfer) and d–d transitions.51
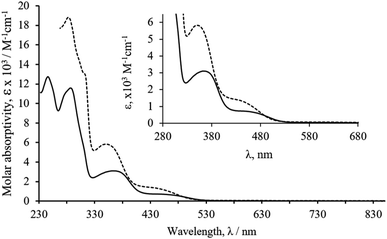 |
| Fig. 1 Electronic spectra of complex RuCB2 in dichloromethane (full line) and dimethylsulfoxide (dotted line). Expansion of the spectra in the LMCT region. | |
Electrochemical studies
The electrochemical behavior of organometallic ruthenacarborane complexes was studied by cyclic voltammetry in acetonitrile and dichloromethane solutions using ammonium hexafluorophosphate as supporting electrolyte (Table S4, see ESI†). Complex RuCB1 (Fig. 2) showed an irreversible oxidation process (Epa = 1.12 V in both solvents) that retains its irreversibility when isolated and studied at different scan rates. This behaviour suggests a rapid irreversible chemical reaction following the electron transfer process, attributed to the ruthenium centre, in accordance with the literature data for similar complexes.51 Along with this oxidative process, and after scanning for negative potentials, two other reductive processes appeared (Epc = −1.45 V and E1/2 = −1.54 V in acetonitrile), being the first irreversible and the second quasi-reversible. These processes can be addressed to the bipyridyl ligand as previously reported for related ruthenium–cyclopentadienyl complexes47 or to a reduction in the ruthenium centre as stated before for similar complexes.51,61 Complex RuCB2 showed a similar behavior in both solvents. Considering the potentials attributed to the RuII/RuIII redox pair for these compounds and the absence of oxidation processes for the analogue [Ru(η5-C5H5)(CO)(bipy)][CF3SO3], in the same experimental conditions,47 one can conclude that the presence of the carboranyl ligand facilitates the oxidation of the metal center, as it was expected by the ability of higher oxidation states stabilization characteristic of the [nido-7,8-C2B9H11]2− ligand.60
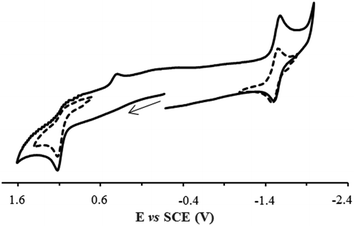 |
| Fig. 2 Cyclic voltammogram of complex RuCB1 in acetonitrile, at 100 mV s−1, showing the reversibility of the isolated redox processes (dashed lines). | |
Single crystal X-ray diffraction of complexes RuCB1 and RuCB2
Single crystal suitable for X-ray diffraction studies were obtained from slow evaporation of acetone (RuCB1) or slow diffusion of hexane solutions into tetrahydrofuran solution (RuCB2) to give an orange to yellow needle-shaped crystals suitable for X-ray diffraction analysis. The molecular structures obtained are shown in Fig. 3, and selected distances and angles and representative crystallographic data are given at Tables 1 and S5.†
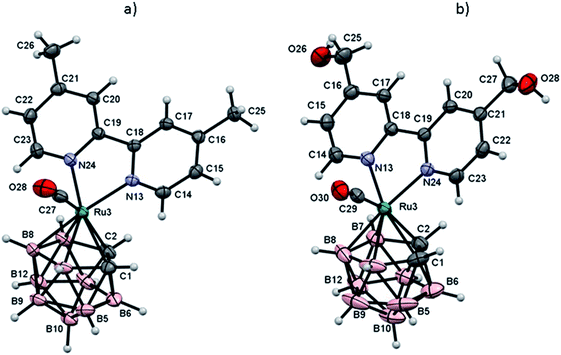 |
| Fig. 3 ORTEP representation of complex RuCB1 (a) and RuCB2 (b). The disordered of –OH groups have been omitted for clarity. | |
Table 1 Selected bond lengths (Å) and angles (deg) for RuCB1 and RuCB2
|
RuCB1 |
RuCB2 |
Ru(3)–C(1) |
2.174(2) |
2.179(3) |
Ru(3)–C(2) |
2.218(2) |
2.220(3) |
Ru(3)–C(27) |
1.863(2) |
— |
Ru(3)–C(29) |
— |
1.865(3) |
Ru(3)–N(13) |
2.1317(17) |
2.104(2) |
Ru(3)–N(24) |
2.1018(18) |
2.125(2) |
Ru(3)–B(4) |
2.214(3) |
2.210(4) |
Ru(3)–B(7) |
2.247(3) |
2.239(4) |
Ru(3)–B(8) |
2.266(3) |
2.272(4) |
C(1)–C(2) |
1.658(3) |
1.642(5) |
C(27)–Ru(3)–N(13) |
94.12(9) |
— |
C(27)–Ru(3)–N(24) |
91.04(9) |
— |
C(27)–Ru(3)–C(1) |
114.93(10) |
— |
C(27)–Ru(3)–C(2) |
158.44(10) |
— |
C(29)–Ru(3)–N(13) |
— |
91.61(12) |
C(29)–Ru(3)–N(24) |
— |
91.17(11) |
C(29)–Ru(3)–C(1) |
— |
114.10(14) |
C(29)–Ru(3)–C(2) |
— |
157.52(13) |
Complex RuCB1 crystallizes in the monoclinic system, space group P21/c whereas complex RuCB2 crystallizes in the monoclinic system, space group P21/n. In the crystallographic studies, it was possible to see that the basic structural skeleton of this type of complexes is the expected three-legged piano stool structure. Each correspondent crystallographic data revealed that the presence of the 2,2′-bipyridyl derivatives does not affect the full engagement of the C2B3 face of the carboranyl ligand towards the ruthenium center, once it remains coordinated in its pentahapto fashion. No evidence of cage slippage or distortion between the carbon atoms of the carboranyl was noticed for any of the complexes. In addition to the fully-coordinated planar 2,2′-bipyridyl ligand (C(1)–C(2) 1.658 Å, Ru–Ccage (average) 2.196 Å, Ru–B (average) 2.240 Å for complex RuCB1, and C(1)–C(2) 1.642 Å, Ru–Ccage (average) 2.200 Å, Ru–B (average) 2.456 Å for complex RuCB2) there is the lone carbonyl co-ligand, bounded in the expected linear form.
The crystal structure of RuCB2 reveals two orientations of molecules (head-to-tail) arranged in infinite double zig-zag chains running parallel to the c crystallographic axis (Fig. 4). The two carborane B–H vertices located at the B5 plane that are trans to the two carbon cluster atoms participate in the B–H⋯O–H intermolecular interactions. These intermolecular B–H⋯H–O interactions are strong since the contacts are shorter than the sum of the van der Waals radii minus 0.70 Å.62
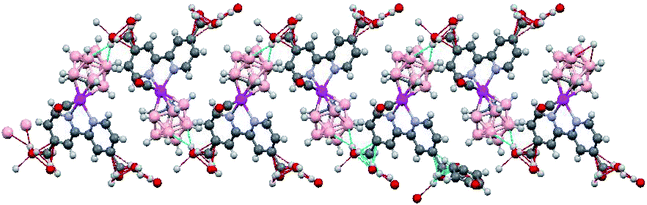 |
| Fig. 4 Crystal structure of complex RuCB2 showing the B–H⋯H–O dihydrogen bonding which results in a head to tail arrangement of molecules forming an infinite double zig-zag chain running parallel to the c crystallographic axis. | |
Stability studies in aqueous media
The behavior of the compounds in cellular media was evaluated to infer about their stability in similar conditions to the biological assays. The study was performed by UV-Vis spectroscopy during 24 h (Fig. S11†). The UV-Vis absorption spectra of the complexes in 3% DMSO/97% DMEM exhibit one strong absorption band in the UV range and two broad absorptions in the visible range, similar to the correspondent spectra observed in organic solvents. Variations lower than 10% over the 24 hours challenge for complex RuCB1 and RuCB2 were observed, supporting that the original three-legged piano-stool geometry is kept over the assay time.
Biological assays
Analysis of the cytotoxicity in cancer cell lines
The cytotoxic activity of RuCB1 and RuCB2 was determined by the colorimetric MTT assay in melanoma (A375) and glioblastoma (U87) cancer cell lines. These cell lines were selected considering that this type of tumors have shown favorable clinical responses with BNCT.63 As indicated in Table 2, after 24 h of treatment with RuCB1 no cytotoxic activity up to 100 μM was observed and RuCB2 showed moderate activity for both cell lines, although more active in the glioblastoma cells. These results indicate that these compounds show the potential to be further explored regarding their boron accumulation in cancer cells.
Table 2 IC50 values (μM) for complexes RuCB1 and RuCB2, at 24 h incubation, in A375 and U87 cancer cells
Compounds |
A375 |
U87 |
RuCB1 |
>100 |
107 ± 46 |
RuCB2 |
57.0 ± 1.8 |
25.5 ± 8.3 |
Intracellular distribution of the ruthenium complexes
The intracellular distribution of the complexes RuCB1 and RuCB2 was performed using A375 cells following exposure to each complex for 24 h at a concentration equivalent to their IC50 values (for compound RuCB1 a concentration of 100 μM was used). Cytosol, membrane, nucleus, and cytoskeletal fractions were extracted using a commercial kit as described in the Experimental section. One can observe, the different substituents at the bipyridine lead to different accumulation patterns (10B and 102Ru quantification) (Fig. 5). While the methylated compound RuCB1 is distributed through cytoskeleton, membranes, and nucleus, the hydroxymethylated compound RuCB2 is mainly accumulated at the membrane of cells (∼90%). It is interesting to observe that RuCB2 keeps the 10B/102Ru ratio unchangeable in all cell compartments indicating that probably the Ru–carborane moiety is stable. However, RuCB1 shows considerable differences indicating that, at some point after internalization, the Ru is detached from the carborane. For RuCB1, the main difference is observed at the nucleus, where the 10B/102Ru ratio increases by ∼3-fold.
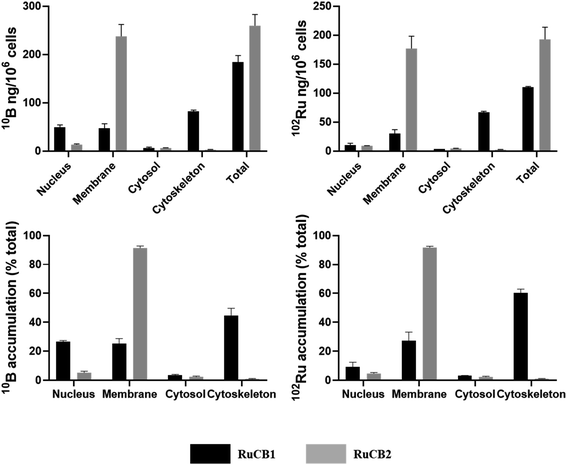 |
| Fig. 5 Cellular 10B and 102Ru distribution in A375 cells treated with compounds RuCB1 and RuCB2 at a concentration equivalent to their IC50 values found at 24 h challenge. Results are expressed in ng of 10B and 102Ru per million of cells (top) or in total percentage (bottom). Results are expressed as mean ± SD of two independent experiments. | |
Normalizing the results to the administered doses of compounds, RuCB2 uptake is 2.6-fold higher than RuCB1 (10B and 102Ru quantification).
For BNCT to be successful, enough 10B should be delivery to the tumor cells. Therefore, experiments were conducted to determine the amount of B that was taken up by the cells as a function of the concentrations of the two compounds.
As shown in Fig. S12,† the most promising compound seems to be RuCB2 since it is the compound presenting higher boron internalization that is proportional to the concentration used (Table 3). Even if the boron internalized by cells is not enriched in 10B, the amount internalized is enough to perform BNCT on cells.64,65 On the contrary, RuCB1 has a low B accumulation within cells, reaching a threshold at ∼30 μM. These results indicate that RuCB2 is a potential candidate to be evaluated as a BNCT agent.
Table 3 Amount of B internalized in A375 cells, determined by ICP-MS
Incubated RuCB2 (μM) |
Internalized B (μg g−1) |
Internalized 10B μg g−1 to perform BNCTa |
10B μg g−1 were calculated from its natural abundance (20%). |
19 |
71 |
14 |
37 |
137 |
27 |
74 |
250 |
50 |
Conclusion
Two new ruthenium carboranyl complexes with 2,2′-bipyridine derivatives were synthesized and characterized by several analytical and spectroscopic techniques. Both compounds crystallize in the monoclinic system, showing the expected three-legged piano stool structure.
The compounds' cytotoxicity was evaluated in melanoma (A375) and glioblastoma (U87) cell lines showing low to moderate cytotoxicity. Their uptake into A375 cells was determined via 10B and 102Ru quantification by ICP-MS showing that RuCB2, bearing a hydroxymethyl group at the bipyridine, fulfills the prerequisites to be further evaluated as a potential bimodal agent (chemo + radiotherapy). As far as we know, this is the first time that a ruthenacarborane bearing 2,2′-bipyridine derivatives has been considered for this application.
Experimental section
General procedures
All reactions and manipulations were performed under nitrogen atmosphere using Schlenk techniques. All solvents used were dried and freshly distilled under nitrogen before use, using standard methods. The carboranyl ligand (C2B9H13) and the [3,3,3-(CO)3-closo-3,1,2-RuC2B9H11] precursor were synthesized as described in the literature.7,66 1H, 11B, and 13C and NMR spectra were recorded on a Bruker Avance 400 spectrometer at probe temperature using commercially available deuterated acetone. 1H and 13C chemical shifts (s = singlet; d = duplet; t = triplet; m = multiplet; comp = complex) are reported in parts per million (ppm) downfield from internal standard Me4Si and the 11B and 11B{1H} NMR spectra are reported in ppm downfield from external standard BF3·OEt2. Coupling constants are reported in Hz. All assignments were attributed using DEPT-135, COSY, HMBC, and HMQC NMR techniques. Infrared spectra were recorded on KBr pellets using a Mattson Satellite FT-IR spectrophotometer. Only considered relevant bands were cited in the text. Electronic spectra were obtained at room temperature on a Jasco V-660 spectrometer from solutions of 10−4 to 10−6 M in quartz cuvettes (1 cm optical path). Elemental analyses were performed at Laboratório de Análises, at Instituto Superior Técnico, using a Fisons Instruments EA1 108 system. Data acquisition, integration and handling were performed using a PC with the software package EAGER-200 (Carlo Erba Instruments).
Syntheses
[3-CO-3,3-{κ2-4,4′-(CH3)2-2,2′-bipy}-closo-3,1,2-RuC2B9H11] (RuCB1). [3,3,3-(CO)3-closo-3,1,2-RuC2B9H11] (0.20 g, 0.63 mmol) was combined with 1 equivalent of 4,4′-dimethyl-2,2′-bipyridine (0.10 g, 0.63 mmol) and 2 equivalents of Me3NO (0.10 g, 1.26 mmol) in a Schlenk and MeCN (40 mL) was added to the reactants. The reaction mixture was stirred for 24 hours at room temperature. After that, the solvent was removed in vacuum and the residue obtained was chromatographed in silica gel using as eluent a mixture of dichloromethane and n-hexane (4
:
1). Extraction yielded a bright canary yellow band that was removed from the column with an increased proportion of the n-hexane phase. After removing all the yellow fraction, the solvent was removed by vacuum. The residue obtained was recrystallized by slow diffusion of n-hexane in dichloromethane. Yellow needle-shaped single crystals of RuCB1 were obtained from slow evaporation of an acetone solution, under air.Yield: 24%; yellow needle-shaped single crystals, recrystallized from dichloromethane/n-hexane. 1H NMR [(CD3)2CO, Me4Si, δ/ppm]: 9.00 (d, 2H, 3JHH = 5.6, H6), 8.56 (s, 2H, H3), 7.56 (d, 2H, 3JHH = 5.2, H5), 3.26 (s, 2H, CHcage), 2.62 (s, 6H, CH3). APT 13C{1H} NMR [(CD3)2CO, Me4Si, δ/ppm]: 198.7 (CO), 156.2 (C2), 155.5 (C6), 151.8 (C4), 128.4 (C5), 124.9 (C3), 44.9 (br, Ccage), 21.2 (CH3). 11B NMR [(CD3)2CO, δ/ppm]: −0.9 (d, 1B, 1JBH = 129.0), −7.3 (m, 5B), −20.9 (d, 3B, 1JBH = 150.2). FTIR [KBr pellets, cm−1]: 2960–2850 cm−1 (νC–H), 2549 cm−1 (νB–H), 1967 cm−1 (νC
O). UV-Vis in CH2Cl2 [λmax/nm (ε × 103/M−1 cm−1)]: 246 (11.28), 287 (10.25), 311 (Sh), 362 (2.74), 451 (0.60). UV-Vis in DMSO [λmax/nm (ε × 103/M−1 cm−1)]: 283 (18.71), 311 (13.01), 350 (5.80), 438 (1.35). Elemental analysis [calculated for RuCB1 ¼CH2Cl2] found (calculated): C 39.1 (39.2), H 5.2 (5.1), N 5.5 (6.0).
[3-CO-3,3-{κ2-4,4′-(CH2OH)2-2,2′-bipy}-closo-3,1,2-RuC2B9H11] (RuCB2). [3,3,3-(CO)3-closo-3,1,2-RuC2B9H11] (0.20 g, 0.63 mmol) was combined with 1 equivalent of 4,4′-dihydroxymethyl-2,2′-bipyridine (0.13 g, 0.63 mmol) and 2 equivalents of Me3NO (0.10 g, 1.26 mmol) in a Schlenk and MeCN (40 mL) was added to the reactants. The reaction mixture was stirred for 24 hours at room temperature. After that period, the solvent was removed by vacuum and the residue obtained was chromatographed in silica gel using as eluent a mixture of dichloromethane and methanol (2
:
0.2). Elution yielded a dark orange band that was removed from the column gradually. Then all the pure fractions were collected and the solvent was removed by vacuum. The residue obtained was recrystallized by slow diffusion of n-hexane in tetrahydrofuran affording light orange needle-shaped single crystals of RuCB2.Yield: 19%; orange needle-shaped single crystals, recrystallized from tetrahydrofuran/n-hexane. 1H NMR [(CD3)2CO, Me4Si, δ/ppm]: 9.09 (d, 2H, 3JHH = 5.6, H6), 8.64 (s, 2H, H3), 7.70 (d, 2H, 3JHH = 5.6, H5), 5.05 (t, 2H, JHH = 5.6, OH), 4.96 (d, 4H, JHH = 4.8, CH2OH), 3.30 (s, 2H, CHcage). APT 13C{1H} NMR [(CD3)2CO, Me4Si, δ/ppm]: 198.5 (CO), 156.2 (C2), 156.1 (C4), 155.7 (C6), 124.6 (C5), 121.0 (C3), 62.7 (CH2OH), 44.9 (br, Ccage). 11B NMR [(CD3)2CO, δ/ppm]: −2.1 (d, 1B, 1JBH = 129.0), −8.6 (m, 5B), −22.3 (d, 3B, 1JBH = 150.2). FTIR [KBr pellets, cm−1]: 3310 cm−1 (νO–H), 2920–2850 cm−1 (νC–H), 2520 cm−1 (νB–H), 1950 cm−1 (νC
O). UV-Vis in CH2Cl2 [λmax/nm (ε × 103/M−1 cm−1)]: 245 (18.92), 289 (16.84), 314 (Sh), 368 (4.33), 453 (1.07). UV-Vis in DMSO [λmax/nm (ε × 103/M−1 cm−1)]: 284 (16.06), 313 (10.45), 347 (4.32), 434 (1.08). Elemental analysis [calculated for RuCB2 ¼THF found (calculated)]: C 38.8 (38.8), H 5.1 (5.1), N 5.1 (5.7).
Electrochemical studies
The electrochemical experiments were performed with an EG&G Princeton Applied Research Model 273A potentiostat/galvanostat and controlled by a personal computer using Electrochemistry PowerSuite v2.51 data acquisition software from Princeton Applied Research. Cyclic voltammograms were obtained in solutions of [NBu4][PF6] in acetonitrile (0.1 M) or dichloromethane (0.2 M) at room temperature using a three-electrode configuration with a platinum-disk working electrode (1.0 mm diameter) probed by a Luggin capillary connected to a silver-wire pseudo-reference electrode; a Pt wire auxiliary electrode was employed. The redox potentials of the complexes were measured in the presence of ferrocene as the internal standard and the redox potential values are normally quoted relative to the ferrocene/ferrocenium redox couple (E1/2 = 0.40 V and 0.46 V vs. SCE for acetonitrile and dichloromethane, respectively). The solutions were purged with nitrogen and kept under an inert atmosphere throughout the measurements. Reagent grade solvents were dried, purified by standard procedures and distilled under nitrogen atmosphere before use.
X-ray structure analysis
The measurements were carried out on a BRUKER SMART APEX CCD diffractometer using graphite-monochromated Mo Kα radiation (λ = 0.71073 Å) from an X-ray Tube. For RuCB1 the measurement was made in the range 1.836 to 28.363° for θ. Hemi-sphere data collection was carried out with ω and φ scans. A total of 13
262 reflections were collected of which 4534 [R(int) = 0.0272] were unique.
For RuCB2 the measurement was made in the range 2.237 to 28.386° for θ. Full-sphere data collection was carried out with ω and φ scans. A total of 35
439 reflections were collected of which 5582 [R(int) = 0.0317] were unique.
The structures were solved by the dual-space algorithm and refined by full-matrix least-squares methods on F2. The non-hydrogen atoms were refined anisotropically. The H-atoms were placed in geometrically optimized positions and forced to ride on the atom to which they are attached, except the carborane B–H and C–H, which were located in the difference Fourier map and refined freely.
The C atoms in the carborane were located using the VCD and BHD methods.67,68
For RuCB2 a considerable amount of electron density attributable to half a disordered THF solvent molecule per asymmetric unit was removed with the SQUEEZE option of PLATON.69 Those solvent molecules are, however, included in the reported chemical formula and derived values (e.g. formula weight, F(000), etc.).
Programs used: data collection, Smart;70 data reduction, Saint+;71 absorption correction, SADABS.72,73 Structure solution and refinement was done using SHELXT – SHELXL.74,75
Stability studies
For the stability studies, both complexes were dissolved in 100% DMSO and a sample containing each compound in 3% DMSO/97% DMEM at ca. 200 μM was prepared. Their electronic spectra were recorded in the range allowed by the solvent mixture at set time intervals. The samples used were protected from light sources and stored at room temperature between measurements.
Cytotoxic activity
The cytotoxic activity of the tested compounds was determined in melanoma A375 and glioblastoma U87 cancer cell lines obtained from ATCC, using the colorimetric MTT (3-(4,5-dimethylthiazol-2-yl)-2,5-diphenyltetrazolium bromide) assay. Both cell lines were grown in DMEM-GlutamaxI medium supplemented with 10% FBS and maintained in a humidified incubator at 37 °C (Heraeus, Germany) with 5% CO2. In a typical assay, cells (1–2 × 104 cells per well) were seeded into 96 well plates and allowed to adhere overnight. Then, cells were treated with crushed dried crystalline samples of the compounds, first diluted in DMSO for complete solubilization, and then in medium to prepare serial dilutions within the concentration range of 0.1–100 μM. After 24 h incubation, the medium was discarded and 200 μL of MTT solution in PBS (0.5 mg mL−1) were added to each well. After 3 h at 37 °C, the MTT solution was removed and replaced by DMSO (200 μL) to solubilize the formazan crystals formed. The percentage of cellular viability was assessed measuring the absorbance at 570 nm using a plate spectrophotometer (Power Wave Xs, Bio-Tek). The IC50 values were calculated using the GraphPad Prism software (version 5.0). Results are shown as the mean ± SD of at least two independent experiments done with six replicates each.
Cellular uptake measured by ICPMS analysis
For the cellular uptake experiments, A375 human melanoma cells (ca. 1 × 106 cells/5 mL) were seeded into t25 flasks and allowed to adhere overnight in a 5% CO2 incubator at 37 °C. Cells settled for 24 h, followed by the addition of RuCB1 and RuCB2, at a concentration equivalent to their IC50 values found for 24 h challenge at 37 °C. After incubation, cells were washed with ice-cold PBS and treated in order to obtain a cellular pellet. The cytosol, membranes/particulate, cytoskeletal and nuclear fractions were extracted using a FractionPREP™ (BioVision, USA) cell fractionation kit according to the manufacturer's protocol. The Ru (102Ru) and B (10B) content in each fraction was measured by a Thermo X-Series Quadrupole ICPMS (Thermo Scientific) after digestion of the samples and using a procedure similar to a previously described.76
Internalization studies as a function of the concentration
A375 human melanoma cells were seeded in 6 cm diameter dishes. After 24 h, cells were incubated for 24 h with increasing concentrations of RuCB1 and RuCB2. At the end of the incubation, cells were washed three times with PBS and detached with trypsin/EDTA. A375 cells were resuspended in 200 μL of PBS, sonicated for 30′′ at 30% power in ice and their protein concentration was measured by the Bradford method. Boron amount [μg g−1] in each cell sample was evaluated by Inductively Coupled Mass Spectrometry (ICP-MS) (Element-2; Thermo-Finnigan, Rodano (MI), Italy) at medium mass resolution. Sample digestion was performed with 1 mL of concentrated HNO3 (70%) using a high-performance Microwave Digestion System (ETHOS UP Milestone, Bergamo, Italy). A natural abundance B standard solution was analyzed during sample runs in order to check changing in the systematic bias. The calibration curve was obtained using four B absorption standard solutions (Sigma-Aldrich) in the range 0.2–0.01 μg mL−1.
Conflicts of interest
There are no conflicts to declare.
Acknowledgements
Centro de Química Estrutural and Centro de Ciências e Tecnologias Nucleares acknowledge Fundação para a Ciência e Tecnologia (FCT) for the Projects UIDB/00100/2020 and UID/MULTI/04349/2013, respectively. This work was also funded in the scope of the project PTDC/QUI-QIN/28662/2017 (FCT). R. G. Teixeira thanks FCT for his Ph.D. Grant (SFRH/BD/135830/2018). A. Valente acknowledges the CEECIND 2017 Initiative (CEECIND/01974/2017) and the COST Actions 17104 STRATAGEM and CM1302 (SIPs) (European Cooperation in Science and Technology). C. Viñas thanks MINECO (CTQ2016-75150-R) and Generalitat de Catalunya (2017 SGR 1720) for the financial support.
References
- P. Zhang and P. J. Sadler, Advances in the design of organometallic anticancer complexes, J. Organomet. Chem., 2017, 839, 5–14 CrossRef CAS.
- L. Zeng, P. Gupta, Y. Chen, E. Wang, L. Ji, H. Chao and Z. S. Chen, The development of anticancer ruthenium(II) complexes: From single molecule compounds to nanomaterials, Chem. Soc. Rev., 2017, 46(19), 5771–5804 RSC.
- R. N. Grimes, Carboranes, Elsevier Inc., 3rd edn, 2016, p. 1058 Search PubMed.
- D. Olid, R. Núñez, C. Viñas and F. Teixidor, Methods to produce B–C, B–P, B–N and B–S bonds in boron clusters, Chem. Soc. Rev., 2013, 42(8), 3318–3336 RSC.
- Y. Taoda, T. Sawabe, Y. Endo, K. Yamaguchi, S. Fujii and H. Kagechika, Identification of an intermediate in the deboronation of ortho-carborane: An adduct of ortho-carborane with two nucleophiles on one boron atom, Chem. Commun., 2008, 17, 2049–2051 RSC.
- L. I. Zakharkin and V. N. Kalinin, On the reaction of amines with barenes, Tetrahedron Lett., 1965, 6(7), 407–409 CrossRef.
- R. A. Wiesboeck and M. F. Hawthorne, Dicarbaundecaborane(13) and Derivatives, J. Am. Chem. Soc., 1964, 86(8), 1642–1643 CrossRef CAS.
- M. G. Davidson, M. A. Fox, T. G. Hibbert, J. A. K. Howard, A. Mackinnon, I. S. Neretin and K. Wade, Deboronation of ortho-carborane by an iminophosphorane: Crystal structures of the novel carborane adduct nido ortho carborane tris(dimethylamino)iminophosphorane complex and the borenium salt [(Me2N)3PNHBNP(NMe2)3]2O2+(C2B9H12−)2, Chem. Commun., 1999,(17), 1649–1650 RSC.
- J. Yoo, J. W. Hwang and Y. Do, Facile and mild deboronation of o-carboranes using cesium fluoride, Inorg. Chem., 2001, 40(3), 568–570 CrossRef CAS PubMed.
- M. A. Fox and K. Wade, Cage-fluorination during deboronation of meta-carboranes, Polyhedron, 1997, 16(14), 2517–2525 CrossRef CAS.
- M. A. Fox, J. A. H. MacBride and K. Wade, Fluoride-ion deboronation of p-fluorophenyl-ortho- and -meta-carboranes. NMR evidence for the new fluoroborate, HOBHF-2, Polyhedron, 1997, 16(14), 2499–2507 CrossRef CAS.
- M. A. Fox, W. R. Gill, P. L. Herbertson, J. A. H. MacBride, K. Wade and H. M. Colquhoun, Deboronation of C-substituted ortho- and meta-closo-carboranes using “wet” fluoride ion solutions, Polyhedron, 1996, 15(4), 565–571 CrossRef CAS.
- L. I. Zakharkin and V. S. Kirillova, Izv. Akad. Nauk SSSR, Ser. Khim., 1975, 2596 CAS.
- R. F. Barth, P. Mi and W. Yang, Boron delivery agents for neutron capture therapy of cancer, Cancer Commun., 2018, 38(1), 1–15 Search PubMed.
- Boron-Based Compounds. Potential and Emerging applications in Medicine, ed. E. Hey-Hawkins and C. Viñas Teixido, John Wiley & Sons Ltd, Chichester, UK, 2018 Search PubMed.
- Boron Science, New Technologies and Applications, ed. N. S. Hosmane, CRC Press, Boca Raton, FL, USA, 2012 Search PubMed.
- Boron, in Medicine in Handbook of Boron Chemistry in Organometallics, Catalysis, Materials and Medicine, ed. N. S. Hosmane and R. Eagling, World Science Publishers, New Jersey, 2018, vol. 4 Search PubMed.
- R. F. Barth, M. G. H. Vicente, O. K. Harling, W. S. Kiger, K. J. Riley, P. J. Binns, F. M. Wagner, M. Suzuki, T. Aihara, I. Kato and S. Kawabata, Current status of boron neutron capture therapy of high grade gliomas and recurrent head and neck cancer, Radiat. Oncol., 2012, 7(146), 1–21 Search PubMed.
- G. Calabrese, A. Daou, E. Barbu and J. Tsibouklis, Towards carborane-functionalised structures for the treatment of brain cancer, Drug Discovery Today, 2018, 23(1), 63–75 CrossRef CAS PubMed.
- Z. Zheng, C. B. Knobler, M. D. Mortimer, G. Kong and M. Frederick Hawthorne, Hydrocarbon-Soluble Mercuracarborands: Syntheses, Halide Complexes, and Supramolecular Chemistry, Inorg. Chem., 1996, 35(5), 1235–1243 CrossRef CAS PubMed.
- E. L. Bregadze and L. P. Beletskaya, Synthesis of binuclear C-carboranylmercury and B-carboranylthallium complexes containing cyclopentadienyl and (dimethylaminomethylcyclopentadienyl)manganese tricarbonyl ligands, Russ. Chem. Bull., 1993, 42(3), 587–589 Search PubMed.
- J. Poater, M. Solà, C. Viñas and F. Teixidor, π Aromaticity and Three-Dimensional Aromaticity: Two sides of the Same Coin, Angew. Chem., Int. Ed., 2014, 53(45), 12191–12195 CrossRef CAS PubMed.
- G. M. A. Junqueira, Remarkable aromaticity of cobalt bis(dicarbollide) derivatives: a NICS study, Theor. Chem. Acc., 2018, 137(92), 1–7 Search PubMed.
- M. Couto, C. Alamón, C. Sánchez, B. Dávila, M. Fernández, N. Lecot, P. Cabral, F. Teixidor, C. Viñas and H. Cerecetto, Carboranylanilinoquinazoline stage in the drug-development pipeline, Future Med. Chem., 2019, 11(17), 2273–2285 Search PubMed.
- E. Oleshkevich, A. Morancho, A. Saha, K. M. O. Galenkamp, A. Grayston, S. G. Crich, D. Alberti, N. Protti, J. X. Comella, F. Teixidor, A. Rosell and C. Viñas, Combining magnetic nanoparticles and icosahedral boron clusters in biocompatible inorganic nanohybrids for cancer therapy, Nanomedicine, 2019, 20(101986), 1–10 Search PubMed.
- I. Fuentes, T. García-Mendiola, S. Sato, M. Pita, H. Nakamura, E. Lorenzo, F. Teixidor, F. Marques and C. Viñas, Metallacarboranes on the Road to Anticancer Therapies: Cellular Uptake, DNA Interaction, and Biological Evaluation of Cobaltabisdicarbollide [COSAN]−, Chem.–Eur. J., 2018, 24(65), 17239–17254 CrossRef CAS PubMed.
- M. Couto, I. Mastandrea, M. Cabrera, P. Cabral, F. Teixidor, H. Cerecetto and C. Viñas, Small-Molecule Kinase-Inhibitors-Loaded Boron Cluster as Hybrid Agents for Glioma-Cell-Targeting Therapy, Chem.–Eur. J., 2017, 23(39), 9233–9238 CrossRef CAS PubMed.
- M. Couto, M. F. García, C. Alamón, M. Cabrera, P. Cabral, A. Merlino, F. Teixidor, H. Cerecetto and C. Viñas, Discovery of Potent EGFR Inhibitors through the Incorporation of a 3D-Aromatic-Boron-Rich-Cluster into the 4-Anilinoquinazoline Scaffold: Potential Drugs for Glioma Treatment, Chem.–Eur. J., 2018, 24(13), 3122–3126 CrossRef CAS PubMed.
- Z. J. Lesnikowski, Boron units as pharmacophores - New applications and opportunities of boron cluster chemistry, Collect. Czech. Chem. Commun., 2007, 72(12), 1646–1658 CrossRef CAS.
- S. Wang, C. Blaha, R. Santos, T. Huynh, T. R. Hayes, D. R. Beckford-Vera, J. E. Blecha, A. S. Hong, M. Fogarty, T. A. Hope, D. R. Raleigh, D. M. Wilson, M. J. Evans, H. F. Vanbrocklin, T. Ozawa and R. R. Flavell, Synthesis and Initial Biological Evaluation of Boron-Containing Prostate-Specific Membrane Antigen Ligands for Treatment of Prostate Cancer Using Boron Neutron Capture Therapy, Mol. Pharm., 2019, 16(9), 3831–3841 CrossRef CAS PubMed.
- S. Sato, S. Ishii and H. Nakamura, Development of Albumin-closo-Dodecaborate Conjugates as Boron Carriers for Neutron-Capture Therapy by Ru(bpy)3-Photocatalyzed Modification of Tyrosine, Eur. J. Inorg. Chem., 2017, 2017(38), 4406–4410 CrossRef CAS.
- T. He and R. A. Musah, Evaluation of the Potential of 2-Amino-3-(1,7-dicarba-closo-dodecaboranyl-1-thio)propanoic acid as a boron neutron capture therapy agent, ACS Omega, 2019, 4(2), 3820–3826 CrossRef CAS.
- A. Isono, M. Tsuji, Y. Sanada, A. Matsushita, S. Masunaga, T. Hirayama and H. Nagasawa, Design, Synthesis, and Evaluation of Lipopeptide Conjugates of Mercaptoundecahydrododecaborate for Boron Neutron Capture Therapy, ChemMedChem, 2019, 823–832 CrossRef CAS PubMed.
- I. Nar, S. Bortolussi, I. Postuma, A. Atsay, E. Berksun, E. Viola, C. Ferrari, L. Cansolino, G. Ricciardi, M. P. Donzello and E. Hamuryudan, A Phthalocyanine-ortho-Carborane Conjugate for Boron Neutron Capture Therapy: Synthesis, Physicochemical Properties, and in vitro Tests, ChemPlusChem, 2019, 84(4), 345–351 CrossRef CAS PubMed.
- A. N. Ay, H. Akar, A. Zaulet, C. Viňas, F. Teixidor and B. Zumreoglu-Karan, Carborane-layered double hydroxide nanohybrids for potential targeted- and magnetically targeted-BNCT applications, Dalton Trans., 2017, 46(10), 3303–3310 RSC.
- D. Kaniowski, K. E. O. Nska, M. Sobczak, B. Wojtczak, S. Janczak, Z. J. Lésnikowski and B. Nawrot, High boron-loaded DNA-oligomers as potential boron neutron capture therapy and antisense oligonucleotide dual-action anticancer agents, Molecules, 2017, 22(9), 1–20 CrossRef PubMed.
- C. Viñas, R. Núñez, I. Bennour and F. Teixidor, Periphery Decorated and Core Initiated Neutral and Polyanionic Borane Large Molecules: Forthcoming and Promising Properties for Medicinal Applications, Curr. Med. Chem., 2019, 26(26), 5036–5076 CrossRef PubMed.
- R. Frank, V. M. Ahrens, S. Boehnke, A. G. Beck-Sickinger and E. Hey-Hawkins, Charge-Compensated Metallacarborane Building Blocks for Conjugation with Peptides, ChemBioChem, 2016, 17(4), 308–317 CrossRef CAS PubMed.
- Z. J. Lesnikowski, Nucleoside-boron cluster conjugates - Beyond pyrimidine nucleosides and carboranes, J. Organomet. Chem., 2009, 694(11), 1771–1775 CrossRef CAS.
- Z. J. Leśnikowski, E. Paradowska, A. B. Olejniczak, M. Studzińska, P. Seekamp, U. Schüßler, D. Gabel, R. F. Schinazi and J. Plešek, Towards new boron carriers for boron neutron capture therapy: Metallacarboranes and their nucleoside conjugates, Bioorg. Med. Chem., 2005, 13(13), 4168–4175 CrossRef PubMed.
- M. Białek-Pietras, A. B. Olejniczak, S. Tachikawa, H. Nakamura and Z. J. Leśnikowski, Towards new boron carriers for boron neutron capture therapy: Metallacarboranes bearing cobalt, iron and chromium and their cholesterol conjugates, Bioorg. Med. Chem., 2013, 21(5), 1136–1142 CrossRef PubMed.
- M. Gozzi, B. Schwarze, M. B. Sárosi, P. Lönnecke, D. Drača, D. Maksimović-Ivanić, S. Mijatović and E. Hey-Hawkins, Antiproliferative activity of (η6-arene)ruthenacarborane sandwich complexes against HCT116 and MCF7 cell lines, Dalton Trans., 2017, 46(36), 12067–12080 RSC.
- M. Gozzi, B. Schwarze and E. Hey-Hawkins, Half- and mixed-sandwich metallacarboranes for potential applications in medicine, Pure Appl. Chem., 2019, 91(4), 563–573 CAS.
- N. P. E. Barry, A. Pitto-Barry, I. Romero-Canelón, J. Tran, J. J. Soldevila-Barreda, I. Hands-Portman, C. J. Smith, N. Kirby, A. P. Dove, R. K. O'Reilly and P. J. Sadler, Precious metal carborane polymer nanoparticles: Characterisation of micellar formulations and anticancer activity, Faraday Discuss., 2014, 175, 229–240 RSC.
- I. Romero-Canelón, B. Phoenix, A. Pitto-Barry, J. Tran, J. J. Soldevila-Barreda, N. Kirby, S. Green, P. J. Sadler and N. P. E. Barry, Arene ruthenium dithiolato-carborane complexes for boron neutron capture therapy (BNCT), J. Organomet. Chem., 2015, 796, 17–25 CrossRef.
- M. Gozzi, B. Murganic, D. Drača, J. Popp, P. Coburger, D. Maksimović-Ivanić, S. Mijatović and E. Hey-Hawkins, Quinoline-Conjugated Ruthenacarboranes: Towards Hybrid Drugs with Dual Mode of Action, ChemMedChem, 2019, 14, 2061–2074 CrossRef CAS PubMed.
- L. Côrte-Real, M. Paula Robalo, F. Marques, G. Nogueira, F. Avecilla, T. J. L. Silva, F. C. Santos, A. Isabel Tomaz, M. Helena Garcia and A. Valente, The key role of coligands in novel ruthenium(II)-cyclopentadienyl bipyridine derivatives: Ranging from non-cytotoxic to highly cytotoxic compounds, J. Inorg. Biochem., 2015, 150, 148–159 CrossRef PubMed.
- L. Côrte-Real, B. Karas, A. R. Brás, A. Pilon, F. Avecilla, F. Marques, A. Preto, B. T. Buckley, K. R. Cooper, C. Doherty, M. Helena Garcia and A. Valente, Ruthenium-Cyclopentadienyl Bipyridine-Biotin Based Compounds: Synthesis and Biological Effect, Inorg. Chem., 2019, 58(14), 9135–9149 CrossRef PubMed.
- T. S. Morais, A. Valente, A. I. Tomaz, F. Marques and M. H. Garcia, Tracking antitumor metallodrugs: Promising agents with the Ru(II)- and Fe(II)-cyclopentadienyl scaffolds, Future Med. Chem., 2016, 8(5), 527–544 CrossRef CAS PubMed.
- L. Côrte-Real, F. Mendes, J. Coimbra, T. S. Morais, A. I. Tomaz, A. Valente, M. H. Garcia, I. Santos, M. Bicho and F. Marques, Anticancer activity of structurally related ruthenium(II) cyclopentadienyl complexes, J. Biol. Inorg Chem., 2014, 19(6), 853–867 CrossRef PubMed.
- P. A. Jelliss, J. Mason, J. M. Nazzoli, J. H. Orlando, A. Vinson, N. P. Rath and M. J. Shaw, Synthesis and characterization of ruthenacarborane complexes incorporating chelating N-donor ligands: Unexpected luminescence from the complex [3-CO-3,3-{κ2-Me2N(CH2)2NMe2}-closo-3,1,2-RuC2B9H11], Inorg. Chem., 2006, 45(1), 370–385 CrossRef CAS PubMed.
- O. Tutusaus, C. Viñas, R. Kivekäs, R. Sillanpää and F. Teixidor, Neutral nido-heteroboranes with non ionisable hydrogen as arenes in coordination, Chem. Commun., 2003, 2458–2459 RSC.
- O. Tutusaus, C. Viñas, R. Núñez, F. Teixidor, A. Demonceau, S. Delfosse, A. F. Noels, I. Mata and E. Molins, The modulating possibilities of dicarbollide clusters: Optimizing the Kharasch catalysts, J. Am. Chem. Soc., 2003, 125(39), 11830–11831 CrossRef CAS PubMed.
- O. Tutusaus, R. Núñez, C. Viñas, F. Teixidor, I. Mata and E. Molins, Synthesis, characterization, and dynamic studies of 12-vertex η5-ruthenium(II) closo-phosphine complexes with monoanionic [10-L-nido-7-R-7,8-C2B9H9] - ligands, Inorg. Chem., 2004, 43(19), 6067–6074 CrossRef CAS PubMed.
- R. Núñez, O. Tutusaus, F. Teixidor, C. Viñas, R. Sillanpää and R. Kivekäs, Formation of new η5-rhodium(III) complexes from η5-Rh(I) rhodacarborane-containing charge-compensated ligands, Organometallics, 2004, 23(10), 2273–2280 CrossRef.
- A. J. Welch, What can we learn from the crystal structures of metallacarboranes?, Crystals, 2017, 7(8), 1–19 CrossRef.
- K. J. Adams, J. Cowie, S. G. D. Henderson, G. J. McCormick and A. J. Welch, Steric effects in heteroboranes, J. Organomet. Chem., 1994, 481(2), C9–C11 CrossRef CAS.
- U. Gradler, A. S. Weller, A. J. Welch and D. Reedb, Synthesis, characterisation and molecular structures of the closo and pseudocloso heptamethylindenyl carbarhodaboranes 1-Ph=3-(eta-C9Me7)-3,1,2-closo-RhC2B9H10 and 1,2-Ph2-3-(eta-C9Me7)-3,1,2-pseudocloso-RhC2B9H9. Experimental assignment of the11B NMR, J. Chem. Soc., Dalton Trans., 1996, 335–342 RSC.
- S. Hermanek, 11B NMR Spectra of Boranes,
Main-Group Heteroboranes, and Substituted Derivatives. Factors Influencing Chemical Shifts of Skeletal Atoms, Chem. Rev., 1992, 92(2), 325–362 CrossRef CAS.
- R. J. Wilson, L. F. Warren and M. F. Hawthorne, The Existence of the Nickel(IV) Dication Derived from Nickelocene and a Cationic Boron Hydride Analog, J. Am. Chem. Soc., 1969, 91(3), 758–759 CrossRef CAS.
- S. Chardon-noblat, A. Deronzier, D. Zsoldos, R. Ziessel, M. Haukka, T. Pakkanen and T. Venalainen, Mode of formation of polymeric [{Ru(bipy)(CO)2}n] (bipy = 2,2′-bipyridine) films, J. Chem. Soc., Dalton Trans., 1996, 2582–2583 Search PubMed.
- L. Pauling, The Nature of the Chemical Bond, Cornell University Press, Ithaca, NY, 3rd edn, 1960 Search PubMed.
- R. F. Barth, Z. Zhang and T. Liu, A realistic appraisal of boron neutron capture therapy as a cancer treatment modality, Cancer Commun., 2018, 38(36), 1–7 Search PubMed.
- R. F. Barth, J. A. Coderre, M. G. H. Vicente and T. E. Blue, Boron neutron capture therapy of cancer: Current status and future prospects, Clin. Cancer Res., 2005, 11(11), 3987–4002 CrossRef CAS PubMed.
- R. F. Barth, Boron neutron capture therapy at the crossroads: Challenges and opportunities, Appl. Radiat. Isot., 2009, 67(7–8 suppl.), 67–70 Search PubMed.
- S. Anderson, D. F. Mullica, E. L. Sappenfield and F. G. A. Stone, Carborane Complexes of Ruthenium: A Convenient Synthesis of [Ru(CO)3(η5-7,8-C2B9H11)] and a Study of Reactions of This Complex, Organometallics, 1995, 14(7), 3516–3526 CrossRef CAS.
- A. McAnaw, G. Scott, L. Elrick, G. M. Rosair and A. J. Welch, The VCD method - A simple and reliable way to distinguish cage C and B atoms in (hetero)carborane structures determined crystallographically, Dalton Trans., 2013, 42(3), 645–664 RSC.
- J. Catterick, M. B. Hursthouse, P. Thornton and A. J. Welch, Crystal and Molecular Structure of Tetra-p-benzoato-bisquinolinedi- cobalt(II), a Binuclear Cobalt(II) Carboxylate, J. Chem. Soc., Dalton Trans., 1977, 223–226 RSC.
- A. L. Spek, Structure validation in chemical crystallography, Acta Crystallogr., Sect. D: Biol. Crystallogr., 2009, 65(2), 148–155 CrossRef CAS PubMed.
- Bruker Advanced X-ray Solutions, SMART: Version 5.631 Search PubMed.
- Bruker Advanced X-ray Solutions, SAINT+, Version 6.36A, 2001 Search PubMed.
- G. M. Sheldrick, Empirical Absorption Correction Program, Universität Göttingen, 1996 Search PubMed.
- Bruker Advanced X-ray Solutions, SADABS Version 2.10, 2001 Search PubMed.
- G. M. Sheldrick, Program for Crystal Structure Refinement, Universität Göttingen, 1997 Search PubMed.
- G. M. Sheldrick, SHELXT-SHELXL-2014/7, 2014 Search PubMed.
- L. Côrte-Real, A. P. Matos, I. Alho, T. S. Morais, A. I. Tomaz, M. H. Garcia, I. Santos, M. P. Bicho and F. Marques, Cellular uptake mechanisms of an antitumor ruthenium compound: The endosomal/lysosomal system as a target for anticancer metal-based drugs, Microsc. Microanal., 2013, 19(5), 1122–1130 CrossRef PubMed.
Footnote |
† Electronic supplementary information (ESI) available: Spectroscopic data for RuCB1 and RuCB2 (NMR, FTIR and UV-Vis); crystallographic data and structural refinement details for X-ray data for RuCB1 and RuCB2; stability curves; in vitro uptake experiments on A375 cells. CCDC 1980631 and 1980632. For ESI and crystallographic data in CIF or other electronic format see DOI: 10.1039/d0ra01522a |
|
This journal is © The Royal Society of Chemistry 2020 |
Click here to see how this site uses Cookies. View our privacy policy here.