DOI:
10.1039/D0RA01307B
(Paper)
RSC Adv., 2020,
10, 14670-14678
The design and growth of peanut-like CuS/BiVO4 composites for photoelectrochemical sensing†
Received
11th February 2020
, Accepted 18th March 2020
First published on 14th April 2020
Abstract
In this study, the CuS/BiVO4-X (where X represents the mass percentage of CuS associated with CuS/BiVO4; X = 2%, 5% and 7%) p–n heterostructures were fabricated using a two-step hydrothermal method. The structural and morphological features were ascertained in great detail using several physical characterization processes. According to the results of the photoelectrochemical (PEC) experimental processes, the PEC properties of CuS/BiVO4-5% were much more obvious as compared to those of pure BiVO4, CuS and CuS/BiVO4-X. Moreover, the photoluminescence (PL) and UV-vis diffuse reflection spectra (DRS) affirmed that the CuS/BiVO4-5% demonstrates an excellent capacity for absorbing visible light and low electron recombination rate as compared with the other composites. Accordingly, PEC sensors with CuS/BiVO4-5% were fabricated for the detection of dopamine (DA) and bisphenol A (BPA) with outstanding selectivity and stability. For DA, it implied a broad linear range from 0.01–10 μM and 10–120 μM, and for BPA, the broad linear range was 0.01–90 μM. Thus, the PEC sensor has significant potential application when it comes to DA and BPA detection.
Introduction
Bisphenol A (BPA, 2,2′-bis(4-hydroxyphenyl)propane) is a characteristic environmental incretin-disrupting element that can adversely affect multiple systems in humans and cause Parkinson's disease (PD) by inducing oxidative stress and inflammation.1–3 Moreover, the basic principle of treating this disease is to increase the content of dopamine (DA) in the substantia nigra.4 Therefore, it is important to adopt a fast and feasible method to detect the levels of DA and BPA.5,6
Many different approaches have been adopted to detect DA and BPA, such as fluorescence capillary electrophoresis, electrochemical and photoelectrochemical (PEC).7–9 To be specific, PEC analysis, with high sensitivity and ideal signal ratio, has drawn significant attention for its high cost-effectiveness, quick response as well as its excellent stability.10–12 The PEC method requires semiconductor materials with electrical and optical properties.13,14 Thus, for ultra-sensitive PEC approaches, semiconductor materials with easy fabrication and broad optical response should be developed.
To date, photocatalysts with a bismuth-system have found application in PEC study, including BiVO4, Bi2MoO6, Bi2WO6, BiPO4 and so on.15–18 Bismuth-system oxides, which are composed of 6s Bi orbitals and 2p oxygen orbitals, display outstanding electrical and optical characteristics.19 Moreover, they enjoy several popular strengths, e.g., abundance, low toxicity, and low cost.20 Among them, bismuth vanadate (BiVO4), an n-type semiconductor, is regarded to be a photocatalyst that is full of potential due to its narrow band gap (2.4 eV),21 together with its wide use in photoelectrocatalytic water splitting,22 PEC sensors23 and PEC CO2 reduction.24 However, it is not efficient in energy conversion due to the rate of rapid charge recombination.25 The combination of other semiconductor elements and BiVO4 will help to improve the electron transfer efficiency and facilitate electron–hole pair separation, thus enhancing the energy conversion efficiency.26
In recent years, sulfide-based photocatalysts (MoS2, WS2, CdS et al.) have become the focus of most exploration as they enjoy a relatively narrow band gap as well as a greater range of light absorption.27–29 Copper sulfide (CuS), a promising p-type semiconductor, can absorb and utilize ultraviolet (UV) and visible light due to its small band gap (2.1 eV).30,31 The heterojunction formed between a p-type and an n-type semiconductor is capable of enhancing the ability to absorb visible light as well as facilitating the separation process of charge carriers.32,33 As such, we designed a complex of BiVO4 and CuS to improve the PEC performance.
In this paper, we adopted a two-step hydrothermal method and successfully synthesized CuS/BiVO4 heterojunction composites. The PEC activity was assessed by detecting DA and BPA under visible light irradiation. The results demonstrate that the CuS/BiVO4 composites developed greater PEC efficiency as compared with CuS and BiVO4, and showed outstanding selectivity, low detection limits and a wide linear range for detecting DA and BPA. According to the results, the sensors with CuS/BiVO4 have great potential for applications in the practical detection of DA and BPA.
Experimental
Reagents
All reagents were of analytical grade and were used without further purification. Bismuth nitrate pentahydrate (Bi(NO3)3·5H2O), ammonium metavanadate (NH4VO3), copper nitrate trihydrate (Cu(NO3)2·3H2O), sodium thiosulfate pentahydrate (Na2S2O3·5H2O) and ethylene glycol (EG) were purchased from Sinopharm Chemical Reagent Co. Ltd. (https://www.sinoreagent.com). Dopamine was purchased from Aladdin Chemical Reagent Co. Ltd. (https://www.aladdin-e.com). Human serum samples were purchased from the People's Hospital of Zhengzhou University, Clinical Bioinformatics Experimental Center.
Preparation of the CuS/BiVO4 heterojunction
The CuS/BiVO4 heterojunction was prepared by a two-step hydrothermal method. To synthesize BiVO4, Bi(NO3)3·5H2O and NH4VO3 were dissolved in a mixture solution of EG and hot water. The solution was transferred into a 100 mL Teflon-lined stainless autoclave and kept at 120 °C for 12 h. The prepared BiVO4 powder was dispersed in distilled water by ultrasonication for 30 min, and appropriate amounts of Cu(NO3)2·3H2O and Na2S2O3·5H2O were added successively. After the addition of 10 mL of ethanol to the above solution under stirring, the mixture was transferred to a 50 mL Teflon-lined stainless autoclave and heated at 200 °C for 12 h. Finally, the product was cleaned and then dried at 60 °C. The CuS/BiVO4 heterojunction composed of different CuS contents was labeled as CuS/BiVO4-X, where X represents the mass percentage of CuS associated with the CuS/BiVO4 heterojunction. For comparison, different CuS/BiVO4 heterojunctions having different mass ratios of CuS (2 wt%, 5 wt% and 7 wt%) were also prepared.
Electrochemical experiments
All electrochemical experimental processes were conducted using a three-electrode system (CHI 660E). Pt wire and a saturated calomel electrode (SCE) played the roles of the counter electrode and reference electrode, respectively. We performed electrochemical impedance spectroscopy (EIS) in the frequency range of 1 to 1
000
000 Hz in 0.1 M phosphate buffer (pH 7.0) produced by mixing the stock solutions of Na2HPO4 and NaH2PO4. The indium tin oxide (ITO) glass served as the working electrode, a xenon lamp (PLS-SXE 300, 100 mW.cm−2, λ ≥ 420 nm) was utilized as the light source. The ITO electrodes (10 × 15 mm) were cleaned separately with ethanol, acetone and water for 5 min. Next, 3 mg catalyst powders were dispersed in a chitosan and ethanol mixed solution (0.5 mL) for the formation of a homogeneous suspension. Subsequently, 20 μL suspensions were coated on ITO electrodes (0.5 cm2).
Characterization
X-ray diffraction (XRD) patterns were obtained using a Bruker D8 Advance diffractometer with Cu Kα radiation. The X-ray photoelectron spectra were achieved with the help of an X-ray photoelectron spectroscope (XPS, ESCALAB 250Xi, Al Kα microfocus monochromator with variable spot size (30–400 μm and 5 μm step size)). Diffuse reflection spectra (DRS) were obtained for the materials, which were verified using a UV-vis spectrophotometer, with BaSO4 as the reference. The morphologies of the samples were characterized by scanning electron microscopy (SEM) and transmission electron microscopy (TEM).
Results and discussion
Choice of materials
The phases of all synthetic materials were studied by XRD (Fig. 1A). For pure CuS, the four typical characteristic peaks around 31.78°, 32.85° and 47.94° correspond to (103), (006) and (110) of CuS (JCPDS 06-0464), while the peaks at 18.98°, 28.82°, 30.54° and 47.30° were attributed to the (110), (121), (040) and (042) lattice planes of BiVO4 (JCPDS No. 14-0688). For CuS/BiVO4, the positions of the peaks were the same even for a variety of composites, with both CuS and BiVO4 peaks being clearly identifiable. More importantly, with the increase in CuS, the (110) peak became more and more prominent. All results indicate that we have successfully synthesized CuS/BiVO4.
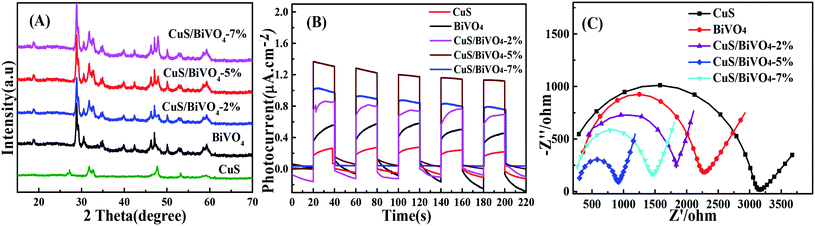 |
| Fig. 1 XRD spectra (A), photocurrent responses (B), and EIS (C) of the CuS, BiVO4 and CuS/BiVO4-X composites in 0.1 M PBS. | |
In order to conduct further evaluation of the PEC performances of CuS, BiVO4 and CuS/BiVO4-X, photocurrent densities of all materials were determined under visible light irradiation in 0.1 M PBS as shown in Fig. 1B. The photocurrent densities of all the materials were of the order CuS/BiVO4-5% > CuS/BiVO4-7% > CuS/BiVO4-2% > BiVO4 > CuS. These results show that CuS can efficiently improve the absorption of visible light and separate electron–hole pairs; excessive CuS will affect the capability of materials in absorbing visible light, thus reducing the PEC of the materials.34
Electrochemical impedance spectroscopy (EIS) was conducted to evaluate the electron transfer kinetics (Fig. 1C). The EIS was performed in 0.1 M PBS, which consisted of extruded semi-circular portions and linear portions. The electron transfer resistance (Rct) was quantified based on the semicircle diameter, and it was ranked as CuS > BiVO4 > CuS/BiVO4-2% > CuS/BiVO4-7% > CuS/BiVO4-5%. Double-layer capacitance (Cdl), which can be obtained from cyclic voltammetry (CV), is directly related to the electrochemical active surface area (A) and the scanning rate v in a linear relationship (Cdl ∝ v × A).35 Therefore, the values of Cdl can be used to indicate A. As shown in Fig. S1,† the Cdl values were ranked CuS (0.29 mF cm−2) < BiVO4 (0.58 mF cm−2) < CuS/BiVO4-2% (0.72 mF cm−2) < CuS/BiVO4-7% (1.31 mF cm−2) < CuS/BiVO4-5% (1.49 mF cm−2). These results are consistent with EIS. All the results indicate that CuS/BiVO4-5% has rapid electron transfer capability, the best electrical conductivity and a large electrochemical active area, which makes it a potential active material. Based on the results shown above, CuS/BiVO4-5% was selected for further investigation.
Physical characterization
SEM, TEM and HRTEM analysis clearly defined the morphology of the pure BiVO4 and CuS/BiVO4-5% composite. The results are shown in Fig. 2. Pure BiVO4 particles had the shape of a peanut with a smooth surface (Fig. 2A and C). As compared to the pure BiVO4, the surface of CuS/BiVO4-5% is rough (Fig. 2B and D). The CuS/BiVO4-5% composite structure was further verified by HRTEM analysis. As shown in Fig. 2E, the lattice fringes of 0.309 and 0.323 nm correspond to the (121) planes of BiVO4 and the (101) planes of CuS, respectively, which suggests the coexistence of BiVO4 and CuS in the composites. Fig. 2F shows the STEM-EDX mapping images of CuS/BiVO4-5%, which indicates that the Bi, V, O, Cu and S elements in the composite surface are uniformly distributed. The results further show that CuS/BiVO4-5% is composed of BiVO4 and CuS, which conforms to the XPS analysis.
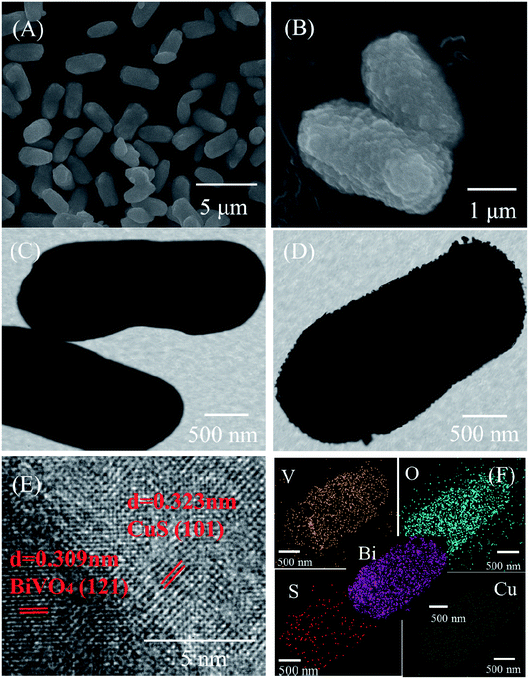 |
| Fig. 2 SEM images of BiVO4 (A) and CuS/BiVO4-5% (B). TEM images of BiVO4 (C) and CuS/BiVO4-5% (D). (E) HR-TEM images of CuS/BiVO4-5%. (F) STEM-EDX mapping images of CuS/BiVO4-5%. | |
We studied the absorption properties of BiVO4, CuS and CuS/BiVO4-5% by UV-vis DRS as shown in Fig. 3A. The pure CuS exhibited strong ultraviolet and visible absorption, while the pure BiVO4 showed the absorption edge at approximately 550 nm. Furthermore, the visible absorption ability of CuS/BiVO4 was significantly higher as compared to BiVO4. All these can be attributed to the inner absorption of CuS. Based on the fundamental idea of electronegativity,36 the band gap energy was calculated using eqn (1):
where
n is the optical transition type, BiVO
4 is a not direct semiconductor and the value of
n is 4. A is a constant,
ν represents the incident light frequency,
h is Planck's constant, and
α is the absorption coefficient. Fig. S2
† suggests that the
Eg energy gap of BiVO
4 is 2.52 eV. CuS refers to a direct semiconductor, so
n is 1.
32 Accordingly, the band gap of CuS was nearly 2.26 eV. Next, the ability of synthetic materials for separating holes and electrons was studied based on PL spectra.
Fig. 3B suggests that CuS/BiVO
4-5% has the lowest intensity as compared with CuS and BiVO
4, suggesting that CuS/BiVO
4-5% has a low electron recombination rate.
16 According to the discussion above, CuS/BiVO
4-5% displayed terrific PEC performance.
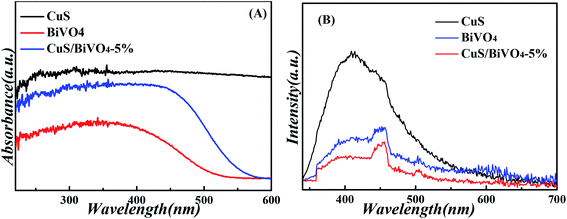 |
| Fig. 3 UV-vis diffuse reflectance spectra (A), and PL spectra (B) of the CuS, BiVO4 and CuS/BiVO4-5% composites. | |
Photoelectrochemical sensor
Fig. 4A and B suggests the photocurrent performance of CuS, BiVO4 and CuS/BiVO4-5% at 0 V vs. SCE with the addition of 1 μM DA and BPA under visible light excitation in 0.1 M PBS. The photocurrent of all synthetic materials increased with the addition of DA and BPA. Meanwhile, the photocurrent of CuS/BiVO4-5% was larger than that of BiVO4, since the p–n heterostructure improved the PEC efficiency for DA and BPA to a large extent.
To further evaluate the PEC performance of CuS/BiVO4-5% for DA and BPA, the photocurrent change was ascertained at many different concentrations of DA and BPA. By the respective addition of DA, the photocurrent response increased significantly as shown in Fig. 4C. A linear relationship was found between the concentration of DA and photocurrent (Fig. 4D), and it displays two linear associations based on the sensor of CuS/BiVO4-5% for DA. From 0.01–10 μM, the linear regressing equation is ΔI = 0.2807 + 0.5622c (R2 = 0.9918), ranging from 10 to 120 μM; the linear association is ΔI = 5.1966 + 0.0712c (R2 = 0.9942). The detection limit of the sensor is 3.4 nM (S/N = 3).
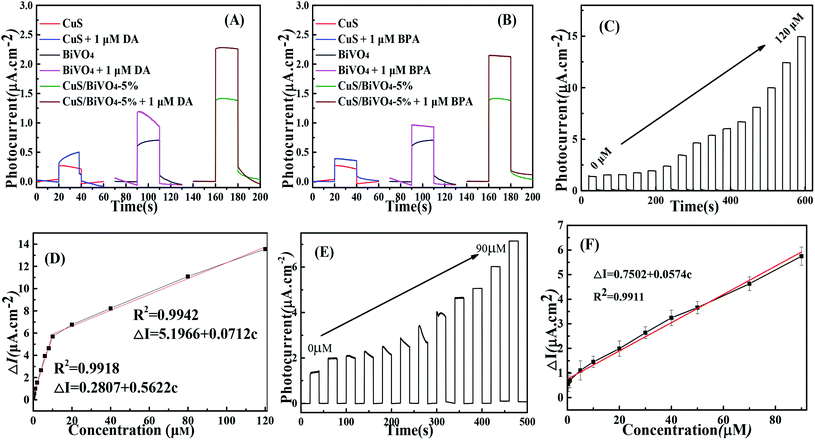 |
| Fig. 4 Photocurrent responses of the CuS, BiVO4 and CuS/BiVO4-5% composites with and without 1 μM DA (A) and 1 μM BPA (B). (C) Photocurrent responses of CuS/BiVO4-5% toward DA with increasing concentrations of DA. (D) The relevant calibration plots of the DA concentration. (E) Photocurrent responses of CuS/BiVO4-5% toward BPA with increasing concentrations of BPA. (F) The relevant calibration plots of the BPA concentration in 0.1 M PBS at 0 V vs. SCE as excited by visible light. | |
Experiments for BPA detection were performed in PBS (Fig. 4E and F). On increasing the BPA concentration in PBS, the photocurrent increased and the as-prepared CuS/BiVO4-5% photoelectrode detected BPA with a linear range from 0.01 μM to 90 μM. The linear regressing equation is ΔI = 0.7052 + 0.0574c (R2 = 0.9911) with a detection limit (S/N = 3) of 7 nM. In Table 1 a comparison is made of CuS/BiVO4-5% with other DA and BPA sensors in the literature. It is undeniable that the CuS/BiVO4-5% sensor outperforms the other DA and BPA sensors in several fields.
Table 1 Comparison of different methods for detecting DA and BPA
Method |
Materials |
Analyst |
Linear range (μM) |
LOD |
Ref. |
Fluorescent |
MoS2 |
DA |
0.1–100 |
10 nM |
42 |
Electrochemical |
CuO/CN |
DA |
0.2–78.7 |
0.06 μM |
4 |
Electrochemical |
RGO-ZnO |
DA |
1–70 |
0.33 μM |
43 |
PEC |
SnSe NSs |
DA |
0.01–10 |
3 nM |
7 |
PEC |
WO3 |
DA |
53–80, 85–155 |
0.3 μM |
44 |
PEC |
CuS/BiVO4 |
DA |
0.01–10 |
3.4 nM |
This work |
10–120 |
Fluorescence polarization immunoassay |
4,4-Bis(4-hydroxyphenyl)valeric acid |
BPA |
0.087–3.5 |
8.7 nM |
45 |
PEC |
TiO2/Au NTAs |
BPA |
0.1–28.9 |
0.047 μM |
9 |
PEC |
ZnPc/TiO2NRs |
BPA |
0.047–52.1 |
8.6 nM |
46 |
PEC |
CuS/BiVO4 |
BPA |
0.01–90 |
7 nM |
This work |
By monitoring the photocurrent of the repeated photoexcitation over 600 s, the CuS/BiVO4-5% stability was also checked (Fig. S3†). The response photocurrent of CuS/BiVO4-5% remained at 95.3% and 95.8% of its initial value towards 1 μM DA and 1 μM BPA within 18 days (Fig. 6A and C). XPS of CuS/BiVO4-5% before the stability test was also evaluated, as shown in Fig. 5A. For Bi 4f, O 1s, V 2p, Cu 2p and S 2s, the high resolution is shown in Fig. 5B–F. Fig. 5B shows two core peaks of the Bi 4f XPS spectra situated at 158.8 eV and 164.2 eV. The peaks at 164.2 eV and 158.8 eV are attributed to Bi 4f5/2 and Bi 4f7/2 in normal Bi3+.37 The two 2p3/2 (516.5 eV) and 2p1/2 (523.9 eV) peaks of biological iodine correspond to V5+ (Fig. 5C).38 The O 1s peak is shown in Fig. 5D, and the O 1s peak at 530.46 eV is due to the lattice oxygen.39 Cu 3p1/2 at 951.8 eV and Mo 3p3/2 at 931.9 eV are matched to Cu2+ in Fig. 5E.40 In Fig. 2F, the 2s peak at around 226.1 eV was attributed to S 2s, which strongly proved how CuS41 was formed. Fig. S4† shows that the XRD and XPS of CuS/BiVO4-5% after the stability test. All the results show that CuS/BiVO4-5% has the best stability. The reproducibility of CuS/BiVO4-5% was tested by detecting 1 μM DA and BPA using 5 parallel electrodes (Fig. 6B and D), and the photocurrent did not exhibit any significant variation, indicating its good reproducibility.
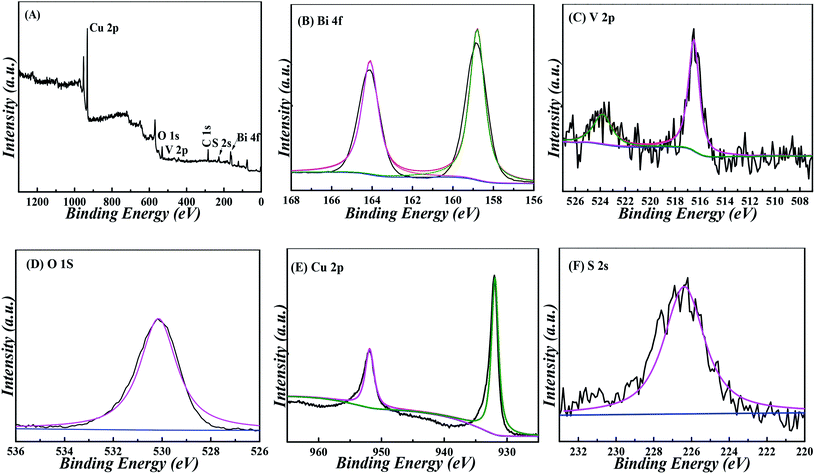 |
| Fig. 5 XPS spectra of CuS/BiVO4-5% composites: (A) survey, (B) Bi 4f, (C) V 2p, (D) O 1s, (E) Cu 2p and (F) S 2s. | |
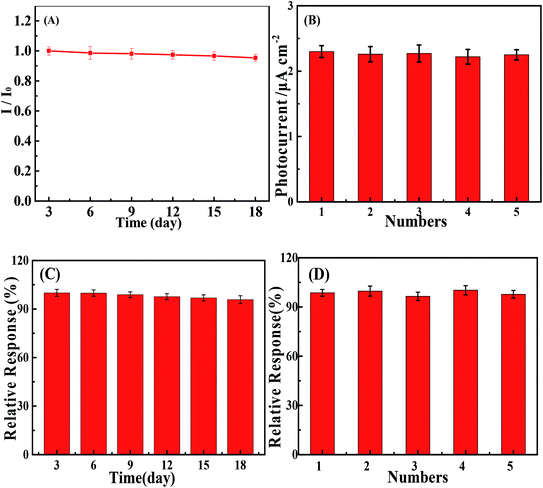 |
| Fig. 6 Stability and reproducibility tests of the PEC sensor based on CuS/BiVO4-5% towards DA (A and B) and BPA (C and D). | |
The selectivity of the PEC sensor based on CuS/BiVO4-5% towards DA and BPA is shown in Fig. S5;† the photocurrent responses of interferences can be ignored, implying that CuS/BiVO4-5% can selectively and effectively detect DA and BPA. To verify the practical reliability of the DA and BPA sensor that has been prepared, we used lake water containing BPA, and human blood serum containing DA as convincing samples. As shown in Table 2, for DA, the RSD values are less than 4.21%, and the recoveries are 99.80–101.0%. For BPA, the RSD values are less than 3.19%, and the recoveries are 98.00–100.12%. According to the mentioned results, the sensor based on CuS/BiVO4-5% is reliable for the detection of DA and BPA in actual samples.
Table 2 PEC detection of DA and BPA in real samples
Real samples |
Added (μM) |
Found (μM) |
Recovery (%) |
RSD (%) |
Human blood serum (DA) |
1.00 |
1.01 |
101.00 |
1.31 |
5.00 |
4.95 |
99.90 |
2.24 |
20.00 |
19.96 |
99.80 |
3.11 |
50.00 |
49.92 |
99.84 |
3.52 |
100.00 |
99.96 |
99.96 |
4.21 |
Lake water (BPA) |
1.00 |
0.98 |
98.00 |
1.01 |
5.00 |
4.98 |
99.60 |
1.95 |
20.00 |
20.02 |
100.10 |
2.33 |
40.00 |
40.05 |
100.12 |
2.71 |
80.00 |
80.09 |
100.11 |
3.19 |
Proposed mechanism of PEC
To explore the mechanism of PEC sensors based on CuS/BiVO4, the valence band energy (EVB) and the conduction band energy (ECB) of BiVO4 and CuS were investigated with the aid of the following equation:47
The value of Ee was almost 4.5 eV, indicating the energy of free the electrons at the scale of hydrogen. X represents the semiconductor electronegativity, and X was 6.04 eV and 5.29 eV for BiVO4 and CuS, respectively. The VB edges of BiVO4 and CuS were +2.8 eV and +1.92 eV, respectively. Besides, we determined the CB edge potentials of BiVO4 and CuS as +0.28 eV and −0.34 eV, respectively. As shown in Fig. 7, the migrating process of photogenerated electrons occurs from the CB of CuS to the CB of BiVO4 and the holes are transferred from the VB of BiVO4 to the VB of CuS due to the dynamic principle, which could help divide the photogenerated electron–hole pairs to effectively utilize the oxidization of holes. By the same token, the holes will be utilized to oxidize DA and BPA.
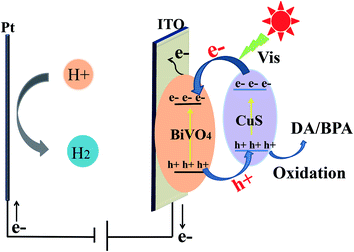 |
| Fig. 7 PEC mechanism of CuS/BiVO4 for the detection of DA and BPA. | |
Conclusion
In summary, we have designed a CuS/BiVO4 composite via a two-step hydrothermal method. CuS/BiVO4-5% exhibited superior PEC properties for DA and BPA as compared with pure BiVO4, CuS and CuS/BiVO4-X. The CuS content had an impact on the PEC efficiency of CuS/BiVO4. The PEC efficiency and stability of CuS/BiVO4 can be attributed to the appropriate CuS content as well as the influential electron–hole pair separation. The study indicates that the sensor based on CuS/BiVO4-5% is sure to possess great application prospects for DA and BPA PEC detection.
Conflicts of interest
There are no conflicts to declare.
Acknowledgements
This work was supported by the Natural Science Foundation of Henan Province (Grant No.: 162300410290) and the National Key Research and Development Program of China (Grant No.: 2016YFC1303204).
References
- T. D. P. Allsop, R. Neal, C. Wang, D. A. Nagel, A. V. Hine, P. Culverhouse, J. D. A. Castañón, D. J. Webb, S. Scarano and M. Minunni, Biosens. Bioelectron., 2019, 135, 102–110 CrossRef CAS PubMed
. - M. K. Li, L. Y. Hu, C. G. Niu, D. W. Huang and G. M. Zeng, Sens. Actuators, B, 2018, 266, 805–811 CrossRef CAS
. - A. S. Alnaimat, M. C. B. Alonso and P. B. Barrera, Microchem. J., 2019, 147, 598–604 CrossRef CAS
. - S. Reddy, B. E. K. Swamy and H. Jayadevappa, Electrochim. Acta, 2012, 61, 78–86 CrossRef CAS
. - M. Annalakshmi, P. Balasubramanian, S. M. Chen, T. W. Chen and P. H. Lin, Inorg. Chem. Front., 2019, 6, 2024–2034 RSC
. - L. A. Goulart, S. A. Alves and L. H. Mascaro, Electroanal. Chem., 2019, 839, 123–133 CrossRef CAS
. - X. Hun, S. S. Wang, S. Y. Mei, H. Q. Qin, H. Zhang and H. L. Luo, Microchim. Acta, 2017, 184, 3333–3338 CrossRef CAS
. - Y. H. Song, J. J. Han, L. J. Xu, L. F. Miao, C. W. Peng and L. Wang, Sens. Actuators, B, 2019, 298, 126949 CrossRef CAS
. - L. S. Hu, C. C. Fong, X. M. Zhang, L. L. Chan, P. K. S. Lam, P. K. Chu, K. Y. Wong and M. S. Yang, Environ. Sci. Technol., 2016, 50, 4430–4438 CrossRef CAS PubMed
. - A. M. Ahmed, F. Mohamed, A. M. Ashraf, M. Shaban, A. A. P. Khan and A. M. Asiri, Chemosphere, 2019, 124554 Search PubMed
. - Y. Liu, Y. S. Chang, Y. J. Hsu, B. J. Hwang and C. H. Hsueh, Fabrication of WO3 photoanode decorated with Au nanoplates and its enhanced photoelectrochemical properties, Electrochim. Acta, 2019, 134674 CrossRef CAS
. - X. L. Tu, M. Li, Y. J. Su, G. L. Yin, J. Lu and D. N. He, Self-templated growth of CuInS2 nanosheet arrays for photoelectrochemical water splitting, J. Alloys Compd., 2019, 151794 CrossRef CAS
. - L. F. Wang, W. Q. Zhu, W. B. Lu, L. N. Shi, R. Wang, R. X. Pang, Y. Y. Cao, F. Wang and X. H. Xu, One-step electrodeposition of AuNi nanodendrite arrays as photoelectrochemical biosensors for glucose and hydrogen peroxide detection, Biosens. Bioelectron., 2019, 111577 CrossRef CAS PubMed
. - F. G. Cai, X. Chen, L. X. Qiu, L. L. Jiang, S. D. Ma, Q. Y. Zhang and Y. Zhao, Controlled hydrothermal synthesis and photoelectrochemical properties of Bi2S3/TiO2 nanotube arrays heterostructure, J. Alloys Compd., 2019, 151770 CrossRef CAS
. - H. L. Tong, Y. Jiang, Q. Zhang, W. C. Jiang, K. L. Wang, X. X. Luo, Z. Lin and L. X. Xia, Boosting Photoelectrochemical Water Oxidation with Cobalt Phosphide Nanosheets on Porous BiVO4, ACS Sustainable Chem. Eng., 2019, 7, 769–778 CrossRef CAS
. - W. J. He, Y. J. Sun, G. M. Jiang, Y. H. Li, X. M. Zhang, Y. X. Zhang, Y. Zhou and F. Dong, Defective Bi4MoO9/Bi metal core/shell heterostructure: Enhanced visible light photocatalysis and reaction mechanism, Appl. Catal., B, 2018, 239, 619–627 CrossRef CAS
. - F. Rong, Q. Y. Wang, Q. F. Lu, L. B. Yao and M. Z. Wei, Rational Fabrication
of Hierarchical Z-Scheme WO3/Bi2WO6 Nanotubes for Superior Photoelectrocatalytic Reaction, ChemistrySelect, 2019, 4, 2676–2684 CrossRef CAS
. - J. Qian, Z. T. Yang, C. Q. Wang, K. Wang, Q. Liu, D. Jiang, Y. T. Yan and K. Wang, One-pot synthesis of BiPO4 functionalized reduced graphene oxide with enhanced photoelectrochemical performance for selective and sensitive detection of chlorpyrifos, J. Mater. Chem. A, 2015, 3, 13671–13678 RSC
. - J. L. Lv, J. F. Zhang, J. Liu, Z. Li, K. Dai and C. H. Liang, Bi SPR-promoted Z-scheme Bi2MoO6/CdS-diethylenetriamine composite with effectively enhanced visible light photocatalytic hydrogen evolution activity and stability, ACS Sustainable Chem. Eng., 2017, 6, 696–706 CrossRef
. - F. Yang, X. M. Zhu, J. Z. Fang, D. D. Chen, W. H. Feng and Z. Q. Fang, One step solvothermal synthesis of Bi/BiPO4/Bi2WO6 heterostructure with oxygen vacancies for enhanced photocatalytic performance, Ceram. Int., 2018, 44, 6918–6925 CrossRef CAS
. - H. L. Li, Y. J. Chen, W. Zhou, H. Y. Jiang, H. Liu, X. Chen and T. G. Hui, WO3/BiVO4/BiOCl porous nanosheet composites from a biomass template for photocatalytic organic pollutant degradation, J. Alloys Compd., 2019, 802, 76–85 CrossRef CAS
. - H. D. She, M. Jiang, P. F. Yue, J. W. Huang, L. Wang, J. Z. Lie, G. Q. Zhu and G. Z. Wang, Metal (Ni2+/Co2+) sulfides modified BiVO4 for effective improvement in photoelectrochemical water splitting, J. Colloid Interface Sci., 2019, 549, 80–88 CrossRef CAS PubMed
. - L. X. Sun, J. H. Sun, N. Han, D. K. Liao, S. L. Bai, X. J. Yang, R. X. Luo, D. Q. Li and A. F. Chen, rGO decorated W doped BiVO4 novel material for sensing detection of trimethylamine, Sens. Actuators, B, 2019, 298, 126749 CrossRef CAS
. - K. J. Li, J. Han, Y. Yang, T. Wang, Y. Q. Feng, S. Ajmal, Y. Y. Liu, Y. Deng, M. A. Tahir and L. W. Zhang, Simultaneous SO2 removal and CO2 reduction in a nano-BiVO4|Cu-In nanoalloy photoelectrochemical cell, Chem. Eng. J., 2019, 355, 11–21 CrossRef CAS
. - F. Li, W. Zhao and D. Y. C. Leung, Enhanced photoelectrocatalytic hydrogen production via Bi/BiVO4 photoanode under visible light irradiation, Appl. Catal., B, 2019, 258, 117954 CrossRef CAS
. - H. C. He, Y. Zhou, G. L. Ke, X. H. Zhong, M. J. Yang, L. Bian, K. L. Lv and F. Q. Dong, Improved Surface Charge Transfer in MoO3/BiVO4 Heterojunction Film for Photoelectrochemical Water Oxidation, Electrochim. Acta, 2017, 257, 181–191 CrossRef CAS
. - T. Kosmala, D. Mosconi, G. Giallongo, G. A. Rizzi and G. Granozzi, Highly Efficient MoS2/Ag2S/Ag Photoelectrocatalyst Obtained from a Recycled DVD Surface, ACS Sustainable Chem. Eng., 2018, 66, 7818–7825 CrossRef
. - C. E. Chieh, C. Jian, C. Wan, Z. J. Huei, H. J. Hsien, L. K. Chan, H. B. Cheng and H. Y. Sheng, Microwave-assisted synthesis of TiO2/WS2 heterojunctions with enhanced photocatalytic activity, J. Taiwan Inst. Chem. Eng., 2018, 91, 489–498 CrossRef
. - H. Wang, H. L. Ye, B. H. Zhang, F. Q. Zhao and B. Z. Zeng, Electrostatic Interaction Mechanism Based Synthesis of Z-Scheme BiOI-CdS Photocatalyst for Selective and Sensitive Detection of Cu2+, J. Mater. Chem. A, 2017, 5, 10599 RSC
. - Y. F. Tang, Y. Chai, X. Q. Liu, L. L. Li, L. W. Yang, P. P. Liu, Y. M. Zhou, H. X. Ju and Y. Z. Cheng, A photoelectrochemical aptasensor constructed
with core-shell CuS-TiO2 heterostructure for detection of microcystin-LR, Biosens. Bioelectron., 2018, 117, 224–231 CrossRef CAS PubMed
. - S. F. Jia, X. Y. Li, B. P. Zhang, J. Yang, S. W. Zhang, S. Li and Z. T. Zhang, TiO2/CuS heterostructure nanowire array photoanodes toward water oxidation: The role of CuS, Appl. Surf. Sci., 2019, 463, 829–837 CrossRef CAS
. - C. Lai, M. M. Zhang, B. S. Li, D. L. Huang, G. M. Zeng, L. Qin, X. G. Liu, H. Yi, M. Cheng, L. Li, Z. Chen and L. Chen, Fabrication of CuS/BiVO4 (040) binary heterojunction photocatalysts with enhanced photocatalytic activity for Ciprofloxacin degradation and mechanism insight, Chem. Eng. J., 2019, 358, 891–902 CrossRef CAS
. - J. Cao, B. Y. Xu, H. L. Lin and S. F. Chen, Highly improved visible light photocatalytic activity of BiPO4 through fabricating a novel p–n heterojunction BiOI/BiPO4 nanocomposite, Chem. Eng. J., 2013, 228, 482–488 CrossRef CAS
. - P. C. Yan, D. S. Jiang, H. N. Li, M. Cheng, L. Xu, J. C. Qian, J. Bao, J. X. Xia and H. M. Li, Exploitation of a photoelectrochemical sensing platform for catechol quantitative determination using BiPO4 nanocrystals/BiOI heterojunction, Anal. Chim. Acta, 2018, 1042, 11–19 CrossRef CAS PubMed
. - J. D. Benck, Z. Chen, L. Y. Kuritzky, A. J. Forman and T. F. Jaramillo, Amorphous Molybdenum Sulfide Catalysts for Electrochemical Hydrogen Production: Insights into the Origin of Their Catalytic Activity, ACS Catal., 2012, 2, 1916–1923 CrossRef CAS
. - W. S. Kuo and P. H. Ho, Solar photocatalytic decolorization of dyes in solution with TiO2 film, Dyes Pigm., 2006, 71, 212–217 CrossRef CAS
. - X. Zhang, X. B. Wang, L. M. Wang, W. K. Wang, L. L. Long, W. W. Li and H. Q. Yu, Synthesis of a highly efficient BiOCl single-crystal nanodisk photocatalyst with exposing {001} Facets, ACS Appl. Mater. Interfaces, 2014, 6, 7766–7772 CrossRef CAS PubMed
. - Z. H. Wei, Y. F. Wang, Y. Y. Li, L. Zhang, H. C. Yao and Z. J. Li, Enhanced photocatalytic CO2 reduction activity of Z-scheme CdS/BiVO4 nanocomposite with thinner BiVO4 nanosheets, J. CO2 Util., 2018, 28, 15–25 CrossRef CAS
. - X. L. Luo, Z. Y. Chen, S. Y. Yang and Y. H. Xu, Two-step hydrothermal synthesis of peanut-shaped molybdenum diselenide/bismuth vanadate (MoSe2/BiVO4) with enhanced visible-light photocatalytic activity for the degradation of glyphosate, J. Colloid Interface Sci., 2018, 532, 456–463 CrossRef CAS PubMed
. - Y. P. Bhoi and B. G. Mishra, Photocatalytic degradation of alachlor using type-II CuS/BiFeO3 heterojunctions as novel photocatalyst under visible light irradiation, Chem. Eng. J., 2018, 344, 391–401 CrossRef CAS
. - Y. P. Bhoi, C. Behera, D. Majhi, S. M. Equeenuddin and B. G. Mishra, Visible light-assisted photocatalytic mineralization of diuron pesticide using novel type II CuS/Bi2W2O9 heterojunctions with a hierarchical microspherical structure, New J. Chem., 2018, 42, 281–292 RSC
. - X. N. Liu, W. T. Zhang, L. J. Huang, N. Hu, W. Liu, Y. N. Liu, S. H. Li, C. Y. Yang, Y. R. Suo and J. L. Wang, Fluorometric determination of dopamine by using molybdenum disulfide quantum dots, Microchim. Acta, 2018, 185, 234 CrossRef PubMed
. - X. Zhang, Y. Zhang and L. Ma, One-pot facile fabrication of graphene-zinc oxide composite and its enhanced sensitivity for simultaneous electrochemical detection of ascorbic acid, dopamine and uric acid, Sens. Actuators, B, 2016, 227, 488–496 CrossRef CAS
. - S. A. Alves, L. L. Soares, L. A. Goulart and L. H. Mascaro, Solvent effects on the photoelectrochemical properties of WO3 and its application as dopamine sensor, J. Solid State Electrochem., 2016, 9, 2461–2470 CrossRef
. - X. L. Wu, L. B. Wang, W. Ma, Y. Y. Zhu, L. G. Xu, H. Kuang and C. L. Xu, A Simple, Sensitive, Rapid and Specific Detection Method for Bisphenol A based on Fluorescence Polarization Immunoassay, Immunol. Invest., 2012, 41, 38–50 CrossRef CAS PubMed
. - Z. Z. Fan, L. F. Fan, S. M. Shuang and C. Dong, Highly sensitive photoelectrochemical sensing of bisphenol A based on zinc phthalocyanine/TiO2 nanorod arrays, Talanta, 2018, 189, 16–23 CrossRef CAS PubMed
. - M. Y. Li, Y. L. Huang, S. Q. Wang, C. Q. Feng, H. M. Wu and H. Mei, Visible light driven photoelectrochemical sensor for chromium(VI) BiOI microspheres decorated with metallic bismuth, Microchim. Acta, 2019, 186, 345 CrossRef PubMed
.
Footnotes |
† Electronic supplementary information (ESI) available. See DOI: 10.1039/d0ra01307b |
‡ Co-first author. |
|
This journal is © The Royal Society of Chemistry 2020 |