DOI:
10.1039/D0RA00656D
(Paper)
RSC Adv., 2020,
10, 13076-13082
Enhanced yellow afterglow in Ca2SnO4:Dy3+ by co-doping Na+/K+
Received
21st January 2020
, Accepted 11th March 2020
First published on 31st March 2020
Abstract
A new yellow long afterglow luminescent material Ca2SnO4:Dy3+ was prepared by the solid state method at 1350 °C. The yellow LLP (long-lasting phosphorescent) material Ca2SnO4:Dy3+ was prepared by co-doping alkaline ions (K+ and Na+) and investigated using an X-ray diffractometer (XRD), photoluminescence spectrometer, afterglow meter and thermoluminescence spectrometer. The excitation and emission spectra showed an excitation peak at 350 nm and an emission peak at 573 nm. The optimized Dy3+ dopant concentration was found to be 1 mol%. For the improvement in the photoluminescence performance, we can co-dope it with alkaline ions (K+ and Na+), with the optimal concentration of the alkaline ions being 1 mol%. On the basis of the experimental results, we constructed a probable model for explaining the mechanism of LAG in detail.
1. Introduction
Long afterglow (LAG) luminescence is an interesting optical process with the decay time extending to seconds, minutes, or even hours after the removal of the excitation source at room temperature. As a unique luminescence phenomenon, recently, LAG has been paid a lot of attention with practical applications in various fields, for example, safety lighting, dial-up displays, decoration, storage media, high-energy ray detection, optical fiber thermometers and live biological imaging.1–5 In this phenomenon, energy is absorbed and stored under excitation (usually ultraviolet and visible light), and emission is visible for a long period even after the excitation stops.6–9 Because of their exceptional properties in luminescence intensity, afterglow time, and chemical stability, a lot of blue and green afterglow phosphors of silicates and aluminates have been sold in the market, while yellow long-lasting phosphors remain relatively backward.10,11 Some reported yellow-emitting long persistent phosphors are Sr3SiO5:Eu2+, Dy3+,12 Ca2Al2Si2O7:Mn2+, Ce3+,13 Sr3Al2O5Cl2:Eu2+, Tm3+,14 Ca2ZnSi2O7:Eu2+, Dy3+,15 Ca2BO3Cl:Eu2+, Dy3+,16 Ca6BaP4O17:Eu2+, and Ho3+.17 However, they have short persistence time and low brightness. Hence, it is urgent and very desirable to explore new types of yellow LAG.
Recently, Shi et al.18 have reported a new yellow long-persistent luminescent Ca2SnO4:Dy3+ whose wideband emission peak is at 573 nm, where we can observe the afterglow for several minutes in the dark after removing the excitation source. Unfortunately, the brightness and duration are not enough for practical applications. In order to use yellow LAG phosphors in practical applications, it is necessary to further enhance their initial intensity and afterglow duration.
At the same time, alkali ions (Li+, K+, Na+, etc.) are well-known high-efficiency sensitizers, thereby enhancing the luminescence performance of the phosphors by charge compensation.19 Therefore, we believe that some intensive studies are still needed to investigate the photoluminescence behavior and afterglow performance of the Dy3+ mono-doped and alkali ion (Li+, K+, Na+) co-doped Ca2SnO4 phosphors. However, Li+ will volatilize when heated at a temperature higher than 1310 °C, and all test reagents may transition to a glassy state, which affects the experimental results.20 To improve the properties of the yellow afterglow phosphors, in this study, we used alkali ions (K+ and Na+) for the improvement in the afterglow performance of the Ca2SnO4:Dy3+ phosphors. The Ca2SnO4 phosphors doped with Dy3+ and alkali metal ions (K+ and Na+) were obtained using the solid state reaction. We studied the luminescence properties and afterglow behavior of the phosphors. The results show that the basic ions K+ and Na+ can effectively improve the photoluminescence and afterglow luminescence of the phosphors.
2. Experimental
All the powder samples were prepared by a high-temperature solid-state reaction. Analytical reagents CaCO3, SnO2, Na2CO3, K2CO3, and Dy2O3 (99.99%) were used as raw materials. The Dy3+ doping concentrations were varied from 0.5 to 5 mol% and the concentration of the co-doped M+ (M = Na, K) was adjusted from 0.2 to 1.5 mol%. In a typical process, raw materials with different stoichiometric amounts were ground together in an agate mortar for 4 h and then pressed into tablets. The tablets were heated up to 1350 °C and held at this temperature for 10 h. Then, the samples were quickly cooled to room temperature with a rate of 20 °C min−1. The fired tablets were ground to obtain the phosphorous powders.
We analyzed the crystalline structure of the samples within the range of 10–70° by X-ray diffraction (XRD) with a TD3500 X-ray diffractometer (Bruker AXS, Karlsruhe, Germany). We obtained the excitation and emission spectra using Xe 900 (450 W xenon arc lamp) by an FLS-920T fluorescence spectrophotometer. The scanning step is 1 nm. We measured the thermoluminescence curve using an FJ-427-A1 thermoluminometer (Beijing Nuclear Instrument Factory) when the heating rate was 1 K s−1. Before the measurement, we irradiated the sample with ultraviolet (350 nm) radiation for different time periods.
3. Results and discussions
3.1 Structural determination
X-ray diffraction (XRD) was used to detect the purity of all the prepared samples. In Fig. 1, we can see the typical XRD patterns of different concentrations of Dy3+ (0.5 mol%, 1 mol%, and 2 mol%) doped in Ca2SnO4 and Ca2SnO4:1 mol% Dy3+, 1 mol% K+ and Ca2SnO4:1 mol% Dy3+, 1 mol% Na+ phosphor samples. We have also shown the pattern for the PDF card no. 46-0112 in Fig. 1; after comparison with it, we can index all the observed peaks to the pure phase of Ca2SnO4. In this system, the Dy3+ ions were incorporated into the Ca2SnO4 lattice by substituting Ca2+ because of their similar ionic radii. All the diffraction peaks can be indexed to an orthorhombic structure according to the reference file PDF #46-0112.
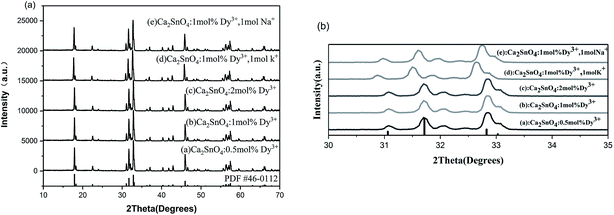 |
| Fig. 1 XRD patterns of (a) Ca2SnO4:0.5 mol% Dy3+, (b) Ca2SnO4:1 mol% Dy3+, (c) Ca2SnO4:2 mol% Dy3+, (d) Ca2SnO4:1 mol% Dy3+, 1 mol% K+ and (e) Ca2SnO4:1 mol% Dy3+, 1 mol% Na+ phosphors. | |
From Fig. 1a, we can see that in Ca2SnO4, the mono-doping of Dy3+ and the co-doping of K+ and Na+ do not result in the formation of impurities or secondary phase. The diffraction peaks of the lattice plane, which are magnified in Fig. 1b, clearly shift towards smaller angles after doping K+ and Na+. Moreover, the shift in the diffraction peak is more obvious when K+ is doped. This is expected since the larger K+ ions replace the smaller Ca2+ ions in the Ca2SnO4 structure, consequently affecting the luminescence of the phosphors (Fig. 2).
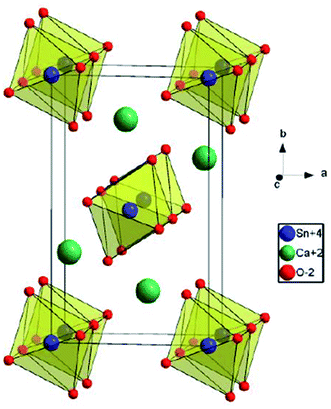 |
| Fig. 2 The crystal structure of Ca2SnO4. | |
Ca2SnO4 crystallizes in the orthorhombic crystal system with the space group Pbam; the unit cell parameters are a = 0.5753 nm, b = 0.9701 nm, and c = 0.3266 nm.21 In the Ca2SnO4 crystal, the shared edges of the SnO6 octahedron are connected into a one-dimensional chain. SnO6 octahedron and 4 of 6 oxygens of the adjacent 2Sn4+ ions form Sn4+–O2− –Sn4 +. The other two O2− ions are connected to the Sn4+ –O2− bond at the end of Sn4+ ion.
SEM was carried out to confirm the morphology of the Ca1.98Dy0.02SnO4 phosphors synthesized at 1350 °C by the solid state reaction in air. The morphological images are shown in Fig. 3. It can be clearly seen from Fig. 3a that the particles are of uniform size with a good degree of dispersion. From Fig. 3b, it is clear that the prepared particles are almost spherical with a dimension in the range of 15–20 μm.
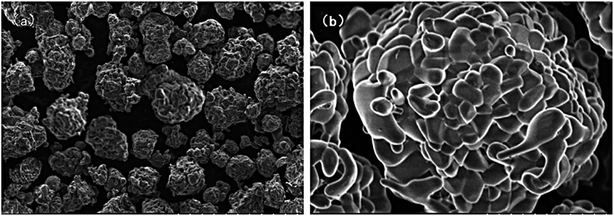 |
| Fig. 3 SEM images of Ca1.98Dy0.02SnO4 phosphor (a) particle uniformity and dispersion, (b) particle shape and size. | |
3.2 Photoluminescence characteristics
From Fig. 4, we can see the PLE as well as PL spectra of the prepared Dy3+-doped Ca2SnO4 phosphor. There is a very strong band peak in the PLE spectrum at 350 nm because of the charge transfer of Dy3+–O2−. When the optimum excitation wavelength is 350 nm, there are several strong peaks in the range of 300–400 nm and 450–700 nm. They consist of the main emission and Dy3+ 4f–4f characteristic transition emission lines near 402 nm as well as the transition of the 4F9/2 excited states to the 6HJ/2 ground states, covering 4F9/2 → 6H15/2 (482 nm), 4F9/2 → 6H13/2 (573 nm) and 4F9/2 → 6H11/2 (672 nm) separately. We can find that the intensity of the magnetic dipole transition 4F9/2 → 6H15/2 around 482 nm is fairly lower than that of the electric dipole transition 4F9/2 → 6H13/2 around 573 nm, implying that the Dy3+ ions occupy the positions with no inversion symmetry in Ca2SnO4.22,23 This is in good agreement with the upper structural analysis results.
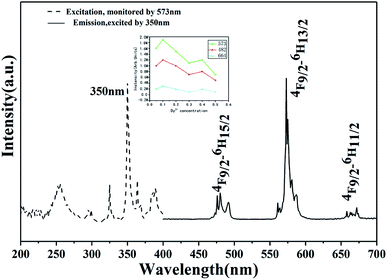 |
| Fig. 4 Excitation (λem = 573 nm) and emission spectra (λex = 350 nm) of Ca2SnO4:1 mol% Dy3+phosphor. | |
To further investigate how the doping of alkaline ions affects the properties of the Ca2SnO4:1 mol% Dy3+ phosphor, the excitation and emission spectra of five phosphors with various concentrations of the alkali ions were tested.24 Fig. 5a, for the Na+-doped phosphor, shows that the excitation and emission intensities increase as the Na+ doping concentration increases. The intensity reaches a maximum for 1% doping and then gradually decreases. Therefore, the optimal doping concentration of sodium ions is 1 mol%. Similarly, we can also observe the results for K+ co-doped Ca2SnO4:1 mol% Dy3+ phosphor in Fig. 5b, which shows that the excitation and emission intensities increase as the doped K+ concentration increases; also, it is determined that the K+ concentration is 1 mol% and the intensity is the largest.
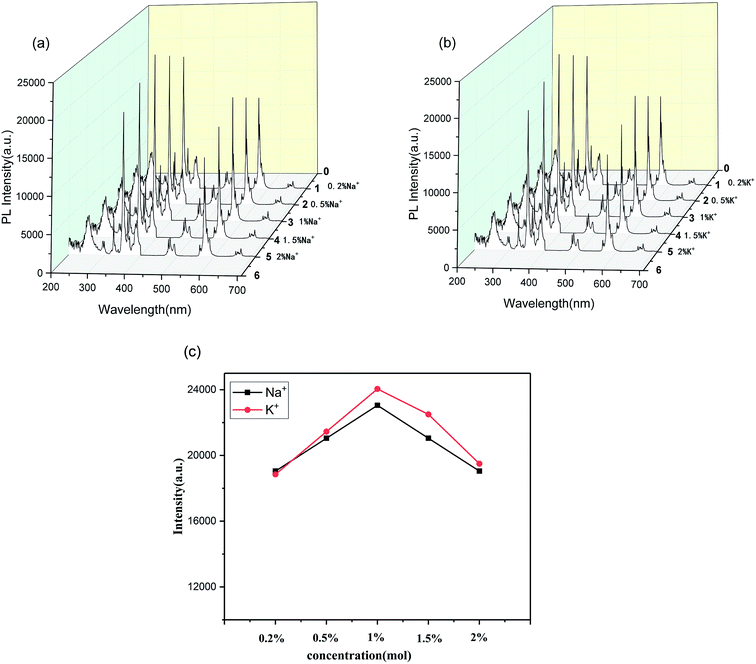 |
| Fig. 5 PL spectra of Ca2SnO4:1 mol% Dy3+ phosphors with (a) Na+ doping and (b) K+ doping, and (c) the difference in K+, Na+ excitation intensities at different concentrations at 350 nm. | |
The effect of K+ and Na+ doping on the PL intensity of the samples was further studied (Fig. 5c). Fig. 5c shows the difference in the excitation intensities of K+ and Na+ at different concentrations at 350 nm. Interestingly, the PL intensity of the Na+-doped phosphors is lower than that of the K+-doped phosphors, which indicates that the K+ ions are better for the luminescence of the developed phosphors. These results largely indicate that we can dope alkali ions to improve the PL characteristics of the developed phosphor.
According to the measured emission spectra, we calculated the CIE chromaticity coordinates of different Ca2SnO4:1 mol% Dy3+, Ca2SnO4:1 mol% Dy3+, 1 mol% K+ and Ca2SnO4:1 mol% Dy3+, 1 mol% Na+ phosphors, as shown in Fig. 6. We calculated the CIE chromaticity coordinates as x = 0.4535, y = 0.4022. The samples co-doped with Na+ and K+ are also labeled in this figure. It is not difficult to see that the co-doping of Na+ or K+ ions has no significant effect on the color of the developed phosphor. Therefore, the as-prepared yellow phosphors have wide application prospects in w-LEDs and display applications.
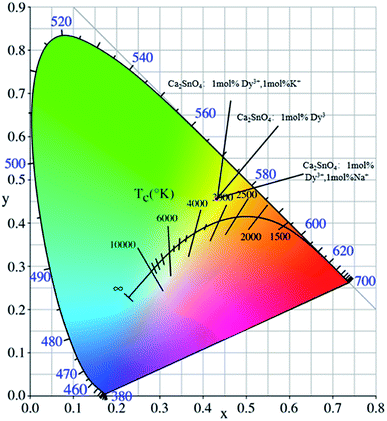 |
| Fig. 6 The CIE 1931 chromaticity coordinates of the Ca2SnO4:1 mol% Dy3+, Ca2SnO4:1 mol% Dy3+, 1 mol% K+ and Ca2SnO4:1 mol% Dy3+, 1 mol% Na+ phosphors. | |
3.3 Afterglow properties
We recorded the decay curves of Ca2SnO4:1 mol%Dy3+, Ca2SnO4:1 mol% Dy3+, 1 mol% K+ and Ca2SnO4:1 mol% Dy3+, Na+ (Fig. 7) immediately after exposure to 350 nm irradiation for 5 minutes. Generally, a decay curve indicates two processes: a fast decay process and a slow decay process. We also measured the afterglow decay curve of the sample for comparison. From Fig. 7, we can see that at the beginning, the decay rate is high; then, we observe a slow decay process mainly because of the faster release rate of the charge carriers in shallow traps and the slower release rate of the charge carriers in deeper traps. It can also be seen from the figure that the initial afterglow intensity and duration of the co-doped phosphors are different from that for the rare earth element-doped phosphors. The afterglow emission and initial photoluminescence intensity of the original Ca2SnO4:1 mol% Dy3+ phosphors are lower than those of the co-doped phosphors. For a further investigation of the reason for the change in the afterglow performance, we fitted the afterglow curves of Ca2SnO4:1 mol% Dy3+, Ca2SnO4:1 mol% Dy3+, 1 mol% K+ and Ca2SnO4:1 mol% Dy3+, 1 mol% Na+ phosphors with the following three attenuation equations:18,25 |
I = I0 + A1 exp(−t/τ1) + A2 exp(−t/τ2) + A3 exp(−t/τ3)
| (1) |
Here, I and I0 represent the phosphorescence intensities, A1, A2 and A3 represent the constants, t represents the time and τ1, τ2, and τ3 represent the decay times for the three exponential components.26 The results obtained using Origin 2017 software can be seen in Table 1. We can see the parameters from Table 1: a three index decay model represents the decay process of these phosphors, and the τ value is an important factor for the evaluation of the afterglow characteristics of the phosphors. The attenuation time τ increases as the afterglow characteristics get better. Single-doped samples have lower brightness than co-doped samples, which signifies the existence of traps. It is confirmed that co-doping with alkaline ions results in better afterglow properties.
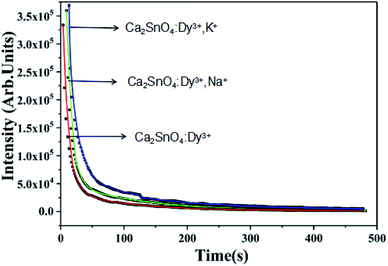 |
| Fig. 7 Afterglow of Ca2SnO4:Dy3+, Ca2SnO4:Dy3+, K+ and Ca2SnO4:Dy3+, Na+ phosphors. | |
Table 1 Simulated results for the afterglow curves of Ca2SnO4:Dy3+, Ca2SnO4:Dy3+, K+ and Ca2SnO4:Dy3+, Na+ phosphors
Composition |
I0 |
A1 |
τ1 (s) |
A2 |
τ2 (s) |
A3 |
τ3 (s) |
Ca2SnO4:Dy3+ |
959 |
1.4 × 107 |
0.8 |
1.2 × 106 |
3.9 |
7.9 × 104 |
27.0 |
Ca2SnO4:Dy3+, K+ |
1658 |
1.7 × 107 |
0.9 |
1.5 × 106 |
4.0 |
9.6 × 104 |
27.0 |
Ca2SnO4:Dy3+, Na+ |
1543 |
1.6 × 107 |
0.8 |
1.4 × 106 |
4.2 |
8.9 × 104 |
2.0 |
It is also reported that the persistent time of the LAG phosphors is affected greatly by the depths, because of the slow release of the trapped charge carriers by thermal stimulation after UV irradiation, traps play an important role in the decay process.27 Hysteresis materials that have an appropriate trap depth and large trap density usually have good properties. Hence, for the determination of the trap center, the thermally stimulated luminescence (TSL) of Dy3+ and alkali ion (K+, Na+) co-doped as well as Dy3+ single-doped Ca2SnO4 phosphors was determined, as shown in Fig. 7.
Once the phosphor is excited, electrons and holes will be produced. These electrons and holes will be captured and released step by step due to thermal disturbance. Then, light can be emitted by the electrons and holes through radiative transitions. In general, these traps can be characterized by thermoluminescence. For the verification of the trap change, we obtained the thermoluminescence (TL) curves (Fig. 8). We can get the trap depth through the following formula:28,29
|
 | (2) |
Here,
I represents the intensity of the luminescence peak,
s represents the frequency factor,
n0 represents the capture concentration of capture carriers,
E represents the capture depth (activation energy),
k represents the Boltzmann constant,
β represents the heating rate (1 K s
−1 in this paper), and
b represents the kinetic order or attempt-to-escape frequency, indicating the order of the capture process:
b = 1 for direct recombination and
b > 1 for remapping the carriers. On the basis of the above-mentioned kinetic equation, all the thermoluminescence curves can be well-fitted by the multi-peak method. Usually, each TL peak is a capture center.
30 We can observe at least two traps of different depths. Accordingly, we can see the cutting parameters of the samples from
Table 2. It can be found that such suitable trap depths were obtained with TSL peaks at low temperature (around 300–400 K). As mentioned above, the obtained phosphors have better decay performance because of the suitable capture types and capture depth.
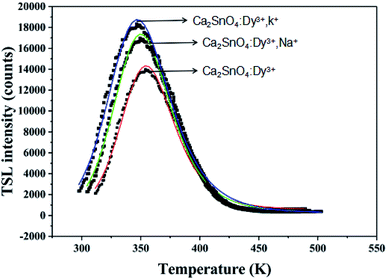 |
| Fig. 8 TSL curves of Ca2SnO4:Dy3+, Ca2SnO4:Dy3, K+ and Ca2SnO4:Dy3, Na+ phosphors. | |
Table 2 TSL parameters from the curve fitting technique
Composition |
Peak temp. (K) |
Et (eV) |
S |
n0 |
Ca2SnO4:Dy3+ |
320 |
0.478 |
1682, 466 |
1.16 × 106 |
Ca2SnO4:Dy3+, K+ |
353 |
0.582 |
3140, 233 |
1.58 × 106 |
Ca2SnO4:Dy3+, Na+ |
347 |
0.571 |
1774, 536 |
1.47 × 106 |
From the fitting peaks, by using the equation E = 25kTm, the trap depths related to the peaks located in the range of 350–375 K were calculated as 0.478 eV for the Ca2SnO4:Dy3+ phosphor, 0.582 eV for Ca2SnO4:Dy3+, K+ and 0.571 eV for Ca2SnO4:Dy3+, Na+.31 Traps of appropriate depths are reported to play an important role in capturing electrons and slowly transporting them to the light-emitting center to produce afterglow.29 If the depth of the traps is very deep, it is difficult for the trapped electrons to escape from the traps and release photons, leading to the degradation of the LLP performance.32,33 If the trap depth is shallow, the trapped electrons can easily go back to the ground state and emit light and thus, the life of LLP is short.32 The results show that the n0 value increases obviously after the co-doping of K+ and Na+. This means that more free electrons can be trapped in more electron traps formed. Thus, we obtained brighter initial phosphorescence and a longer afterglow time. As reported before, Dy3+ acts as a trap supplier. In the trap, replacing Ca2+ will cause defects in the crystal. We can express the process as 3Ca = 2DyCa* + VCa. Two positive defects of electrons captured by DyCa* and a negative vacancy of VCa will be produced due to each substitution by two Dy3+ ions .24,25 The doping of Dy3+ will produce deeper traps and determine the afterglow in the later stage. In addition, the introduction of basic ions M+ (K+, Na+) can deepen the traps and increase the trap density, thus capturing more electrons and producing longer and stronger afterglow. We can write the equation as follows:
|
Dy3+ + M+ + 2CaCa2+ = DyCa + MCa′ + 2Ca2+
| (3) |
Based on the experimental results, for a clearer study of the afterglow process, we can see the phosphorescence mechanism of Ca2SnO4:Dy3+ (Fig. 9) and it is described by the following key steps: (1) under UV irradiation, the electrons in the ground state of Dy3+ are excited to enter the conduction band (CB), due to which the holes remain in the ground state. (2) Some of the excited electrons are transferred to the excited levels of Dy3+ through relaxation; then, yellow photoluminescence of Dy3+ and the recombination of the electrons and holes occur. (3) In VB, electron traps can trap and store some excited electrons, while hole traps can capture and store holes. Therefore, part of the excitation energy is stored instead of returning to the ground state for photoluminescence.34 (4) After UV irradiation is cut off, the electrons are released slowly from the traps under the action of thermal activation and transferred to high-energy Dy3+ and then, the electrons and holes combine, resulting in yellow light emission of the Dy3+ ions.
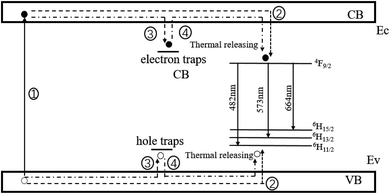 |
| Fig. 9 A schematic graph of the phosphorescence mechanism for Ca2SnO4:1 mol% Dy3+; ○ represent hole; ● represent electron. | |
4. Conclusion
We obtained a yellow LLP phosphor Ca2SnO4:Dy3+ by the conventional solid state method at 1350 °C in air. XRD analysis showed that all the samples exhibited a pure phase. Under the excitation of UV light, a rare earth activator has the ability of bright self-activation and sensitization. The luminescence and afterglow properties improved by doping basic ions (K+, Na+). The best doping concentration of K+ and Na+ was 1 mol%. On this basis, a simplified hysteretic mechanism model was proposed.
Conflicts of interest
There are no conflicts to declare.
Acknowledgements
This work was supported by the National Natural Science Foundation of China (61901270), the Shanghai Sailing Program (17YF1419200) and the Science and Technology Planning Project of Zhejiang Province, China (2018C01046).
References
- H. A. Höppe, H. Lutz, P. Morys, W. Schnick and A. Seilmeier, J. Phys. Chem. Solids, 2000, 61, 2001–2006 CrossRef.
- M. Kowatari, D. Koyama, Y. Satoh, K. Iinuma and S. Uchida, Nucl. Instrum. Methods Phys. Res., Sect. A, 2002, 480, 431–439 CrossRef CAS.
- X. Wang, D. Jia and W. M. Yen, J. Lumin., 2003, 102–103, 34 CrossRef CAS.
- J. Chen, H. Guo, Z. Li, H. Zhang and Y. Zhuang, Opt. Mater., 2010, 32, 998 CrossRef CAS.
- Z. Pan, Y.-Y. Lu and F. Liu, Nat. Mater., 2011, 11, 58 CrossRef PubMed.
- W. Zeng, Y. H. Wang, S. C. Han, W. B. Chen and G. Li, Investigation on long-persistent luminescence of Ca2BO3Cl:Eu2+, Ln3+ (Ln = Nd, Dy, Er), Opt. Mater., 2014, 36, 1819–1821 CrossRef CAS.
- S. Som, S. Dutta, V. Kumar, A. Pandey, V. Kumar, A. K. Kunti, J. Priya, S. K. Sharma, J. J. Terblans and H. C. Swart, CaTiO3:Eu3+, a potential red long lasting phosphor: energy migration and characterization of trap level distribution, J. Alloys Compd., 2015, 622, 1068–1073 CrossRef CAS.
- J. C. Zhang, Q. S. Qin, M. H. Yu, M. J. Zhou and Y. H. Wang, The photoluminescence, afterglow and up conversion photostimulated luminescence of Eu3+ doped Mg2SnO4 phosphors, J. Lumin., 2012, 13, 23–26 CrossRef.
- W. Y. Li, Y. L. Liu and P. F. Ai, Synthesis and luminescence properties of red long-lasting phosphor Y2O2S:Eu3+, Mg2+, Ti4+ nanoparticles, Mater. Chem. Phys., 2010, 119, 52–56 CrossRef CAS.
- B. F. Lei, B. Li, H. R. Zhang and W. L. Li, Preparation and luminescence properties of CaSnO3:Sm3+ phosphor emitting in the reddish orange region, Opt. Mater., 2007, 29, 1491–1494 CrossRef CAS.
- B. F. Lei, B. Li, H. R. Zhang, L. M. Zhang, Y. Cong and W. L. Li, Synthesis and luminescence properties of cube-structured CaSnO3/RE3+(RE = Pr, Tb) long-lasting phosphors, J. Electrochem. Soc., 2007, 154, H623–H630 CrossRef CAS.
- P. Dorenbos, J. Lumin., 2003, 104, 239 CrossRef CAS.
- X.-J. Wang, D. Jia and W. M. Yen, J. Lumin., 2003, 102–103, 34 CrossRef CAS.
- Y. Li, Y. Wang, Y. Gong, X. Xu and M. Zhou, Opt. Express, 2010, 18, 24853 CrossRef CAS PubMed.
- L. Jiang, S. Xiao, X. Yang, X. Zhang, X. Liu, B. Zhou and X. Jin, Mater. Sci. Eng., B, 2013, 178, 123 CrossRef CAS.
- W. Zeng, Y. Wang, S. Han, W. Chen, G. Li, Y. Wang and Y. Wen, J. Mater. Chem. C, 2013, 1, 3004 RSC.
- H. Guo, W. Chen, W. Zeng, G. Li, Y. Wang, Y. Li, Y. Li and X. Ding, J. Mater. Chem. C, 2015, 3, 5844 RSC.
- M. Shi, D. Zhang and C. Chang, Dy3 :Ca2SnO4, a new yellow phosphor with afterglow behavior, J. Alloys Compd., 2015, 639, 168–172 CrossRef CAS.
- A. K. Bedyal, V. Kumar and H. C. Swart, Charge compensated derived enhanced red emission from Sr3(VO4)2:Eu3+ nanophosphors for white light emitting diodes and flat panel displays, J. Alloys Compd., 2017, 709, 362–372 CrossRef CAS.
- C. S. Sunandana and T. Kumaraswami, Li4TeO4: a new, glassy Li+-ion conductor, J. Non-Cryst. Solids, 1986, 85(1–2), 247–250 CrossRef CAS.
- R. Gupta, S. M. Kumar and F. Hussain, Syntheses, Crystal Structures and Solid-State Properties of the Lanthanoid-Containing Nanoclusters, Eur. J. Inorg. Chem., 2014, 2014(35), 6031–6038 CrossRef CAS.
- F. Shi-Liu, T. Yin and C. Fei, Synthesis and characterization of Ca2Sn1−xCexO4 with blue luminescence originating from Ce4+ charge transfer transition, Chin. Phys., 2007, 16(10), 3129–3133 CrossRef.
- M. Irfanullah and K. Iftikhar, A comparative study of 1H NMR and sensitized visible light emission of an extended series of dinuclear lanthanide complexes, J. Photochem. Photobiol., A, 2011, 224(1), 91–101 CrossRef CAS.
- L. Ü. Xiao, M. Sun and J. Zhang, Effect of mixing process on the luminescent properties of SrAl2O4:Eu2+, Dy3+ long afterglow phosphors, J. Rare Earths, 2010, 28(1), 150–152 CrossRef.
- Y. P. Huang and J. W. Lin, The trap bag concept of afterglow luminescence, Sci. Rep., 2017, 7(1), 8475 CrossRef PubMed.
- J.-Y. Bae, Reevaluation of dietary methionine requirement by plasma methionine and ammonia concentrations in surgically modified rainbow trout, Oncorhynchus mykiss, J. Appl. Ichthyol., 2010, 27(3), 887–891 CrossRef.
- G. Li, Y. Wang, W. Zeng, W. Chen, S. Han, H. Guo and X. Wang, Luminescence properties of a new green afterglow phosphor NaBaScSi2O7:Eu2+, Dalton Trans., 2015, 44, 17572–17578 RSC.
- S. W. S. McKeever and R. Chen, Luminescence models, Radiat. Meas., 1997, 27, 625–661 CrossRef CAS.
- J. N. Demas, B. A. DeGraff and W. Xu, Modeling of luminescence quenching-based sensors: comparison of multisite and nonlinear gas solubility models, Anal. Chem., 1995, 67, 1377–1380 CrossRef CAS.
- Z. Zou, C. Cao and T. Zhan, Chem Inform Abstract: Structure, Long Persistent Luminescent Properties and Mechanism of a Novel Efficient Red Emitting Ca2Ga2GeO7:Pr3+ Phosphor, Cheminform, 2016, 47(30), 397–405 CrossRef.
- C. S. Shalgaonkar and A. V. Narlikar, Review: a review of the recent methods for determining trap depth from glow curves, J. Mater. Sci., 1972, 7, 1465e1471 CrossRef.
- X. Ting, G. Hongxu, J. Zhang, C. Odetola, Y. He, H. Lin, G. Chen and Z. Zheng, Phosphorescence behavior and photoluminescence mechanism of Dy3+, sensitized β-Zn3(PO4)2:Mn2+, phosphor, J. Alloys Compd., 2015, 642, 225e231 Search PubMed.
- Y. Li, Y. Wang, Y. Xiong, T. Peng and M. Mo, Effect of electron traps on long afterglow behavior of Sr3Al2O6:Eu0.012+, Dy0.02−x3+, Hox3+, J. Rare Earths, 2012, 30, 105e109 Search PubMed.
- Z. Zehua, Structure, long persistent luminescent properties and mechanism of a novel efficient red emitting Ca2Ga2GeO7:Pr3+ phosphor, J. Alloys Compd., 2016, 397 Search PubMed.
|
This journal is © The Royal Society of Chemistry 2020 |