DOI:
10.1039/C9RA10344A
(Paper)
RSC Adv., 2020,
10, 16928-16941
Bioprocessing of soybeans (Glycine max L.) by solid-state fermentation with Eurotium cristatum YL-1 improves total phenolic content, isoflavone aglycones, and antioxidant activity
Received
10th December 2019
, Accepted 22nd April 2020
First published on 30th April 2020
Abstract
In this study, soybean (Glycine max L.) was bioprocessed with fungal strain Eurotium cristatum YL-1 by using the solid-state fermentation (SSF) technique. The effect of SSF on total phenolic content (TPC), isoflavone compositions, and antioxidant activity of soybean during different fermentation periods was evaluated. Results showed that TPC and isoflavone aglycones were significantly increased, whereas glucoside isoflavones were remarkably reduced during SSF. After 15 days of SSF, the TPC, daidzein, genistein, and total aglycones of soybeans were approximately 1.9-, 10.4-, 8.4-, and 9.4-fold higher, respectively, than those of non-fermented soybeans. During SSF, β-glucosidase activity was very high, whereas α-amylase and protease activities were at moderate levels, and cellulase activity was relatively low. A highly positive correlation was found between TPC and the activities of α-amylase (correlation coefficient R2 = 0.9452), β-glucosidase (R2 = 0.9559), cellulase (R2 = 0.9783), and protease (R2 = 0.6785). Linear analysis validated that the β-glucosidase produced by E. cristatum contributed to the bioconversion of soybean isoflavone glucosides into their aglycone forms. The DPPH radical and ABTS˙+ scavenging activity, reducing power, and ferric reducing antioxidant power of soybeans were considerably enhanced during SSF. Principal component analysis and Pearson's correlation analysis verified that the improvement in TPC and isoflavone aglycone content during SSF was mainly responsible for the improved antioxidant capacity of soybeans. Thus, our results demonstrated that solid-state bioprocessing with E. cristatum is an effective approach for the enhancement of the TPC, isoflavone aglycones, and antioxidant capacity of soybeans. Bioprocessed soybean products might be a healthy food supplement rich in antioxidants compared with non-fermented soybean and thus could be a source of natural antioxidants.
1. Introduction
The development of economical technologies to enhance the nutritional value and bioactive compounds of natural resources (e.g., legumes and cereals) has received considerable attention in the past years.1–4 Solid-state fermentation (SSF) bioprocessing has emerged as a rapidly growing food process technology due to its ability to improve the nutritional quality and biological activity of food products and reduce production costs.5–9 Furthermore, SSF is quite safe, non-toxic, and eco-friendly and depends on the use of specific starter cultures.4,10 Filamentous fungi require very low levels of water activity and substrates for their growth. Many previous studies have reported that filamentous fungi exhibit a great potential to modulate bioactive compounds and augment the bioactivities of legume and cereal products by SSF, making them the ideal and most commonly applied microorganisms for SSF. Thus, filamentous fungi have received considerable interest from researchers/food scientists.6,11 For instance, SSF with Aspergillus awamori Nakazawa was used to improve the total phenolic content (TPC) and antioxidant properties of different wheat cultivars.12 Salar et al.13 reported that SSF with Aspergillus sojae fungal mycelia enhances the bioactive profile, antioxidant potential, and protection against DNA damage of pearl millet. Previous studies have shown that most phenolic compounds in cereals, legumes, and fruits are present in complex insoluble-bound forms as conjugates with cell wall components (such as lignin, cellulose, and polysaccharides) through ether, ester, or glycosidic bonds.6,14 During SSF, filamentous fungi can produce different types of carbohydrate-cleaving enzymes, such as α-amylase, pectinases, cellulases, and β-glucosidase, which can all effectively hydrolyze ether, ester, or glycosidic bonds; liberate the insoluble bound; and improve the antioxidant activity of food products.6,10 Thus, SSF is considered as a promising process technology for enhancing the nutritional value of food products.4,7
In various edible sources, soybean (Glycine max L.) is one of the most important cultivated legumes that exhibit numerous health beneficial properties, such as antioxidant, anticancer, and anti-atherosclerosis, which are mainly attributed to the richness of primary and secondary metabolites, including protein, amino acids, carbohydrate fractions, isoflavone, phenolic acid, and saponin.15,16 Soybeans and soybean-based products (e.g., natto, douchi, and doenjang) have been greatly consumed in Asian countries (e.g., China, South Korea, Japan, and India) for several centuries and now are gaining an increasing interest from Western countries due to their high nutritional qualities and physicochemical functions.17,18 Soybeans contain abundant isoflavones, which possess potent biological activities, including antioxidant, anti-obesity, estrogenic activity, immunomodulatory, and cholesterol-reducing activities.19 The biological effects of isoflavones are significantly affected by their chemical structure.20 Some earlier studies have reported that aglycone forms (e.g., daidzein and genistein) exhibit higher biological effect and are absorbed faster and in greater amounts than their glucoside forms (e.g., daidzin and genistin).21,22 However, glucoside forms are predominantly present in soybeans, which account for 68.0–93.0% of total isoflavone content.21,23 As a result, the healthy beneficial effect of soybean is greatly influenced. Previous studies have verified that the SSF of soybean is a valuable approach to accumulate isoflavone aglycones and phenolic compounds and consequently enhance its biological activity. For example, Ahmad et al.24 demonstrated that the SSF of soybean with Rhizopus sp. contributes to the transformation of glucoside forms (i.e., daidzin and genistin) into their corresponding aglycone forms (i.e., daidzein and genistein) and enhances their β-secretase inhibitory and antioxidant activities. Lee et al.15 also found that the TPC and isoflavone aglycone content of soybeans are considerably increased by SSF with Tricholoma matsutake mycelia at different fermentation times (0–12 days) and that the radical scavenging activities (including DPPH, ABTS, and hydroxyl free radicals) and α-glucosidase inhibitory property of soybean are significantly augmented.
Eurotium cristatum is a predominant probiotic fungus traditionally used in manufacturing Fu-brick dark tea and plays a crucial role on the quality and function of this tea.25–27 E. cristatum forms a colony of golden yellow-like flowers, commonly known as “gold flower.” E. cristatum is safe, nontoxic, and generally recognized as a safe fungal strain.28,29 This fungus is characterized by its low free water content requirement of the substrate during fermentation and is easily grown on various substrate materials. During incubation periods, E. cristatum effectively biotransforms compounds into many natural materials and thus improves their bioactivities. Many previous studies have focused specifically on the transformation of medicinal materials or tea compounds by SSF with E. cristatum.29–32 For instance, Gu et al.30 found that the processing of Hippophae rhamnoides leaves with E. cristatum leads to the bioconversion of rutin into quercetin, kaempferol, and isorhamnetin and enhances radical scavenging activities and ferric reducing antioxidant power (FRAP). Yao et al.28 and Jiang et al.31 reported that loose tea and Pu'er tea processed by E. cristatum exhibit significantly increased antioxidant potential and lipid-lowering activities, respectively. Nevertheless, to the best of our knowledge, the influences of SSF with E. cristatum on the chemical compositions and biological effects of legumes and cereals have received little attention.
The present study aimed to investigate the influence of SSF bioprocessing with E. cristatum on the contents and compositions of phenolics and isoflavones and the antioxidant capacity (evaluated by scavenging activity of DPPH and ABTS radical, reducing power, and FRAP) of soybeans. Furthermore, the hydrolytic enzymes (including cellulase, β-glucosidase, α-amylase, and protease) produced by E. cristatum during SSF and their relationship with phenolic liberation and isoflavone transformation were investigated. Principal component and correlation analyses were carried out to determine which components are the crucial factors that contributed to the change in antioxidant activity during soybean bioprocessing. This work provides a theoretical basis for the enhancement of phenolic and isoflavone aglycone of soybean matrices during SSF and lays a solid foundation for providing more effective process approaches to enhance the biological effect of soybean.
2. Materials and methods
2.1 Materials
2,4,6-Tris(2-pyridyl)-S-triazine (TPTZ), Folin–Ciocalteu reagent, gallic acid, 2,2-azinobis(3-ethylbenzothiazoline-6-sulphonic acid)diammonium salt (ABTS), 2,2-diphenyl-1-picrylhydrazyl (DPPH), daidzin, genistin, daidzein and genistein, p-nitrophenol, p-nitrophenyl-β-D-glucoside (pNPβG) were obtained from Sigma-Aldrich Chemical Co. (St. Louis, MO, USA). Acetonitrile was HPLC grade and purchased from Tedia (Fairfield, OH, USA). All other used reagents and chemicals in this study were of analytical grade. Soybeans (Glycine max L.) were obtained from the Dongzhiyuan supermarket (Changsha, Hunan Province, China). Eurotium cristatum YL-1, which was firstly isolated from Fuzhuan brick-tea (Anhua, Hunan, China) in our group, was stored on M40Y agar slant and subculture monthly. E. cristatum slants were incubated for 7 days at 28 °C and preserved at 4 °C subsequently.
2.2 Preparation of inoculum starter
The inoculum starter used for bioprocessing soybean was prepared based on the described method as follows. Briefly, the preserved E. cristatum YL-1 was carried out for two successive transfers in M40Y agar medium. Firstly, E. cristatum was inoculated into M40Y medium for 7 days at 28 °C. Then, the activated culture was again propagated in M40Y plates at 28 °C to generate large amount of golden spores. The golden mycelia and spores were scraped from the plate into sterile water using a sterilized glass rod. The mycelia and spores were then filtering with absorbent cotton into a flask and shaken with several sterilized small glass. A hemocytometer was performed to determine the spore counts and working spore concentration were adjusted to 106 to 107 spores per mL. All microbiological experiments were performed in a sterilized environment.
2.3 Solid-state bioprocessing of soybeans
The soybeans were firstly rinsed under tap water and then with deionized water. The seeds were immersed in deionized water for 60 min at room temperature. The resulting wet soybean seeds were sterilized in an autoclave for 25 min at 121 °C. After cooling to 25 °C, the soybeans were inoculated with E. cristatum spore suspension (5 mL/100 g) and the inoculated soybeans were placed for 15 days at 28 °C for bioprocessing. Samples were aseptically withdrawn at 0, 2, 4, 6, 8, 10 and 15 days for further analyses. All the samples obtained were freeze-dried and crushed using an electric grinder (FW 100, Beijing Yongguangming Medical Instrument Co., Ltd., Beijing, China) separately. The crushed soybean flour was then passed through a 60-mesh sieve and store at 4 °C in the dark in order to further analysis.
2.4 Enzyme extraction
The enzyme solution was prepared in light of the method reported by Bhanja et al.14 and Salar et al.33 Soybean samples mixed with 20 folds (w/v) of deionized water in extraction bottle which put in a water bath shaker and then extracted at 30 °C for 1 h. The supernatant was collected after the mixtures were centrifuged at 8000g for 10 min at 4 °C. The obtained supernatant was used as the crude enzyme solutions and assayed for α-amylase, β-glucosidase and carboxymethylcellulase activities.
2.4.1 Assay of α-amylase activity. The α-amylase activity was assayed based on the method of Bhanja et al.14 reported. Briefly, 1.0 mL of extracted enzyme solution with appropriate dilution mixed with 0.1 M sodium citrate–citrate buffer (2.0 mL, pH 5.0) and kept at 50 °C for 5 min. After that, 5 mL of 1% soluble starch solution (w/v) added into the mixture and incubated for 10 min at 50 °C. The content of glucose released from the soluble starch was determined by the 3,5-dinitrosalicylic acid (DNS) approach as follows. 1 mL of test sample boiled with 2 mL of DNS reagent and then cooling down to room temperature. The absorbance of the solution was measured at 540 nm after the mixture diluted with distilled water to 10 mL. A standard curve was plotted using glucose. A unit of α-amylase activity was described as the amount of enzyme that hydrolyzes starch to release one micromole of glucose per minute at 50 °C under the evaluation conditions. The results obtained were presented as U g−1 of dry soybean flour.
2.4.2 β-Glucosidase activity assay. The activity of β-glucosidase was assayed based on the method of Salar et al.33 0.1 mL of enzymes solution was mixed with 0.8 mL of 100 mM acetate buffer (pH 5.0) and 0.1 mL of 5 mM p-nitrophenyl-β-D-glucoside (pNPβG). After 30 min incubated at 50 °C, 2 mL of 1 M Na2CO3 solution was added to stop the reaction mixture, the released p-nitrophenol was recorded at 405 nm. Different concentrations of pure p-nitrophenol were prepared to establish the standard curve. A unit of β-glucosidase activity was calculated as the amount of enzyme that catalyzes pNPβG to liberate one micromole of p-nitrophenol per minute at pH 5.0 under the incubation temperature (50 °C). The β-glucosidase activity was recorded as U per gram of dry soybean substrate performed in initial extraction.
2.4.3 Assay of carboxymethylcellulase (CMCase) activity. The assay of CMCase activity is referred to the approach of Ang et al.34 Briefly, 0.5 mL of 2.0% (w/v) carboxymethylcellulose solution in 0.05 mol L−1 acetate buffer (pH 5.0) was added with 0.5 mL of suitably diluted crude enzyme solution, the mixture incubated at 50 °C for 30 min. The amount of released glucose was evaluated with the DNS approach as shown in Section 2.4.1. A unit of CMCase activity was calculated as the amount of enzyme that hydrolyzes carboxymethylcellulose to release one micromole of glucose per minute at pH 5.0 at 50 °C. The cellulase activity was recorded as U per gram of dry soybean substrate.
2.4.4 Assay of protease activity. Protease activity was assayed using casein as the substrate based on the procedure reported by Chen et al.35 with minor modifications. In brief, 1 mL of suitably diluted crude enzyme solution was mixed with 1 mL of 0.05 M phosphate buffer (pH 7.5) that containing 1% (w/v) casein. After 10 min incubated at 40 °C on a gyratory shaker, 2 mL of 0.4 M trichloroacetic acid (TCA) solution was added to terminate the reaction mixture, and centrifuged at 10
000g for 10 min at 4 °C. Then, 1 mL of collected supernatant was thoroughly mixed with 5 mL Na2CO3 and 1 mL of Folin–Ciocalteu reagent and incubated for 20 min at 40 °C. The absorbance of the solution was measured at 660 nm and tyrosine was prepared to establish the standard curve. One unit of protease activity was described as the amount of enzyme that hydrolyzes casein to release one microgram of tyrosine per minute at 40 °C under the evaluation conditions. The protease activity was presented as U per gram of dry soybean substrate.
2.5 Preparation of solvent extracts
Sample powder was extracted with 40 mL of 80% (v/v) aqueous ethanol for 40 min in an ultrasonic bath (40 kHz, 40 °C). The supernatants were obtained after the extracts were centrifuged at 4 °C for 15 min at 12
000g (3–18R refrigerated centrifuge, Hunan Hengnuo Instrument Equipment Co., Ltd., Hunan, China). The residues were then extracted with two additional 40 mL aqueous ethanol under the same conditions. The combined ethanolic extracts were evaporated to dryness at 40 °C using a vacuum evaporator RE-52 (Shanghai Yarong Biochemical Instrument Factory, Shanghai, Chins), and then re-suspended in 25 mL of 80% (v/v) aqueous ethanol. The resultant extracts stored at −20 °C in the dark for further analysis.
2.6 Evaluation of total phenolics content (TPC)
Folin–Ciocalteu method was applied to determine the total phenolics content of the studied samples.36 Briefly, 2.3 mL of deionized water was added into 0.2 mL of sample extracts and then mixed with 0.5 mL of Folin–Ciocalteu reagent. After 1 min of incubation, 2 mL of sodium carbonate solution (75 g L−1) was added to initiate the reaction and maintained for 2 h at ambient temperature (25 °C) in dark place. The absorbance was then recorded at 760 nm after incubation. Gallic acid solution with concentration of 10–200 μg mL−1 was prepared to establish the standard curve. The obtained standard curve equation is y = 0.0021x + 0.002 (R2 = 0.9996). Samples were conducted in triplicate for independent analysis and TPC were presented as μg gallic acid equivalent (GAE) in one-gram dry soybean (μg GAE per g d.w.).
2.7 Analysis of isoflavone compositions with high performance liquid chromatography (HPLC)
It was well established that isoflavones consist of the main phenolics in soybean seeds or its products, whereas genistin, genistein, daidzin and daidzein are the major isoflavones. Therefore, the four isoflavones of the soybean samples were determined using HPLC. First, a syringe filter equipped with 0.45 μm PVDF membrane was used to filter the soybean extracts (which obtained in part 2.5), then 20 μL of the obtained solution was injected to the Waters e2695 HPLC system (Waters Co., Milford, MA) for analysis of specific isoflavones. A ZORBAX SB-C18 reverse-phase column (Eclipse Plus, Agilent Technologies, USA) (5 μm particle size; 4.6 × 250 mm) was performed and maintained at 25 °C. The solvent flow rate was set at 0.8 mL min−1. Deionized water was used as mobile A and acetonitrile was used as mobile B. A gradient elution program was carried out during the separation process as follows: 0–3 min from 10% to 20% B; 3–8 min, 20–25% B; 8–30 min, from 25% to 60% B; 30–35 min, 60–100% B; 35–37 min from 100% to 10% B. The monitored wavelength was set at 254 nm and measured with a photodiode array detector (Waters 2998 PDA Detector; Waters Co., Milford, MA). The identification and quantification of genistin, genistein, daidzin and daidzein were carried out by comparing the UV spectra and retention times with their authentic standards, respectively.21,37 The isoflavones content were presented as μg in one-gram dry soybean (μg g−1 d.w.).
2.8 Evaluation of antioxidant activity
2.8.1 DPPH radical scavenging activity assay. Scavenging activity against DPPH radical was performed according to Xiao et al.38 reported. Briefly, 2 mL of 0.4 mmol L−1 DPPH solution (dissolved in methanol) was mixed with 2 mL of test sample solution, then the mixture was shaken vigorously using a vortex mixer and react for 30 min in the dark place at room temperature before measuring absorbance at 517 nm. Calibration curve of different vitamin C concentrations was prepared to determine the vitamin C-equivalent antioxidant capacity. Scavenging activity of DPPH radical results were presented in μg vitamin C equivalents in one-gram dry soybean (μg VCE per g d.w.).
2.8.2 Determination of ABTS free radical (ABTS˙+) scavenging activity. Scavenging activity against ABTS˙+ was evaluated in light of the approach of Lee et al.15 ABTS˙+ stock solution was conducted by mixing 2.45 mM K2S2O8 with 7 mM ABTS and incubated at room temperature for 12 h in the darkness. Ethanol was used to dilute the ABTS˙+ stock solution to obtain absorbance of 0.70 ± 0.02 (on the condition of 734 nm). 1 mL of diluted soybean sample extract was added into 4 mL of ABTS˙+ solution and shaken well, then the absorbance of the mixture was measured at 734 nm after it kept for 6 min in the dark. Calibration curve of different vitamin C concentrations was prepared to determine the vitamin C-equivalent antioxidant capacity, and ABTS˙+ scavenging activity results were presented in μg vitamin C equivalents in one-gram dry soybean (μg VCE per g d.w.).
2.8.3 Evaluation of ferric reducing antioxidant power (FRAP). The FRAP of the test soybean samples was evaluated using the procedure reported by Đorđević et al.39 Fresh FRAP reagent was performed by mixing 10 mL TPTZ (10 mM, dissolved in 40 mM HCl), with 10 mL ferric chloride (20 mM) in 100 mL of pH 3.6 acetate buffer (0.3 M). 1 mL of test sample was mixed with 5 mL of fresh prepared FRAP solution, and then incubated at 37 °C in the dark for 20 min. The absorbance was recorded at 593 nm against a blank. Different concentrations of FeSO4 (100–1600 μM) standard solutions were conducted to plot the calibration curve. The calibration curve equation was y = 0.0013x − 0.0012 (R2 = 0.9998). The results of FRAP were presented as μmol Fe(II) per g dry weight soybean. A greater of FRAP value is indication of a stronger antioxidant activity.
2.8.4 Reducing power assay. The assay of reducing power referred to the method of Zhang and Yu.23 Briefly, 2.5 mL of 1% (w/v) potassium ferricyanide solution and 2.5 mL of 0.2 M phosphate buffer (pH 6.6) were added into to 0.5 mL of the evaluated sample solution, then incubated for 20 min at 50 °C. The mixture reaction was terminated by adding 10% (w/v) trichloroacetic acid solution (2.5 mL) and then centrifuged at 420 g for 10 min. After that, 0.5 mL of FeCl3 solution 0.1% (w/v) was added into 2.5 mL of the collected supernatant and kept in the dark place at room temperature for 10 min. The absorbance was immediately recorded at 700 nm. A higher absorbance of mixture is indicative of a higher reducing power of the test sample. Standard calibration curve was plotted using different concentration of vitamin C. The result of reducing power was presented as μg vitamin C equivalent in one-gram dry soybean (μg VCE per g d.w.).
2.9 Statistical analysis
All the experiments were carried out in triplicate and the data were presented as the mean value ± standard deviation and conducted with one-way analysis of variance (ANOVA). Significant differences (p < 0.05) between the means were determined using the Duncan's multiple range test which conducted by SPSS version 17.0 (SPSS Inc., Chicago, USA). Correlation analysis was investigated to evaluate the relationship among parameters. Principal component analysis (PCA) was performed to obtain an overview of the relationships between total phenolic, isoflavones, enzymes activity and antioxidant activity. OriginPro 8.1 statistical software (OriginLab Co., USA) was carried out to prepare the data graphs.
3. Results and discussion
3.1 Evaluation of TPC and hydrolytic enzymes activities during SSF
Phenolic compounds are regarded as natural antioxidants and important biological compounds in food matrices.8,38,40,41 In the present study, the TPC changes in the soybean substrate bioprocessed by SFF with E. cristatum are illustrated in Fig. 1. The TPC of soybeans varied during SFF. After 4 days of fermentation, the TPC of soybeans significantly increased compared with that of the non-fermented counterpart (day 0, control). Thereafter, steady increasing levels were observed (days 4–10). As shown in Fig. 1, the TPC of soybeans increased to 5205 ± 27 μg GAE per g d.w. after 15 days of fermentation, which was approximately 1.9-fold higher than that of the non-fermented counterpart (2716 μg GAE per g d.w.). The enhanced TPC in soybean after SSF was consistent with previous findings reported by other investigators. Singh et al.,42 Salar et al.,13 and Kang et al.43 verified that the TPC of soybeans, pearl millet, and buckwheat are remarkably enhanced by SSF with Trichoderma harzianum, Aspergillus sojae, and Agaricus bisporus, respectively. Thus, the improvement of TPC during fermentation can be attributed to the mobilization of sample phenolics by starter microorganisms. These microorganisms produce some hydrolytic enzymes, such as β-glucosidase, α-amylase, and protease, which can hydrolyze the covalent bonds between the bound phenolics and other groups, such as proteins, amines, starches, and other some polysaccharides.14,44 Salar et al.13 found that α-amylase produced by Aspergillus sojae is mainly responsible for the enhanced phenolic compounds of pearl millet during SSF, and they reported that TPC and α-amylase enzyme are highly and positively correlated (correlation coefficient R2 = 0.901, p < 0.01). Earlier studies have demonstrated that most phenolic compounds in legume and cereal grains are covalently bound to cell wall structural components, such as cellulose, arabinoxylans, lignin, pectin, structural proteins, and hemicellulose, which are difficult to extract.45,46 SSF is a complex biochemical process in which several hydrolytic enzymes (e.g., cellulase, β-glucosidase, amylases, and proteases) are produced and contribute to the hydrolyzation of the covalent bonds between the cell wall structural components and phenolics; as a result, the insoluble-bound phenolics are released and facilitate extraction.7,47,48 For instance, insoluble-bound phenolics can form ester linkages with structural protein/carbohydrates and ether linkages with lignin, which can be hydrolyzed by cellulase and liberate free phenolics.46,49 Furthermore, the decomposition of cell wall structures induced by fermentation is favorable to the release of phenolic compounds.7,10 Some soluble phenolic compounds may be synthesized by microorganisms during fermentation.48 Hydrolytic enzymes can hydrolyze the protein, cellulose, or starch of soybean substrates into small available molecule peptides, amino acids, and oligosaccharides, which can be used as energy and make the microorganism grow well in soybean, thereby improving the biosynthesis of phenolic compounds.35,50
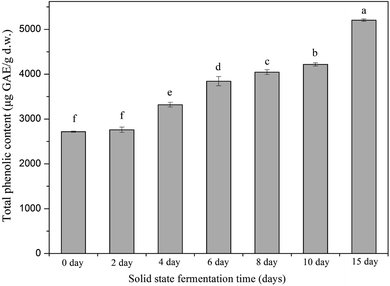 |
| Fig. 1 Changes in total phenolics content of soybean during solid-state bioprocessing with E. cristatum. Data were reported as the mean value ± standard deviation of three replicates. Mean values followed by the different letters demonstrated significantly (p < 0.05) different among the samples. | |
In this study, the key hydrolytic enzymes produced by E. cristatum were assayed to study the precise hydrolytic enzymes that mobilized the phenolic compounds of soybeans during SSF, and the results are depicted in Fig. 2. β-Glucosidase activity was very high during soybean bioprocessing, α-amylase and protease activities were at moderate levels, and cellulase (CMCase) activity was low during the whole fermentation period. However, all three carbohydrate-cleaving enzyme activities enhanced during SSF. α-Amylase activity showed steady increasing levels between days 2 and 10 (ranging from 1.8 U g−1 to 13.3 U g−1). Elevated α-amylase activity (39 U g−1) was observed at the later period of fermentation (day 15). Previous studies have shown that fungal mycelia can produce abundant α-amylase during fermentation, and the produced α-amylase is effective in releasing phenolic compounds. Wang et al.50 and Salar et al. found that α-amylase and phenolic compounds are significantly correlated (p < 0.01). In the present study, α-amylase also displayed highly positive correlations (R2 = 0.9452, p < 0.01) with the liberation of phenolics, as shown in Fig. 3A. β-Glucosidase activity was low during the early growth phase (days 0–2) and then significantly increased until day 4 (473 U g−1). After 6 days of bioprocessing, the β-glucosidase activity of the soybean substrate increased up to 2152 ± 146 U g−1 and continued to increase with the fermentation time. β-Glucosidase activity reached up to 4216 U g−1 at the late stage of SSF (day 15). Previous studies have also reported that other various filamentous fungi such as R. oligosporus, A. awamori, and A. oryzae grown on soybeans and cereal substrates can produce β-glucosidase during fermentation.3,12,51 Other studies showed that β-glucosidase can catalyze the hydrolysis of partial glycosidic bonds between two or more carbohydrates or between carbohydrates and non-carbohydrates.52 In the present study, the produced β-glucosidase during the fungal bio-fermentation changed the phenolic compounds in the fermented substrate. Cheng et al.51 reported that the R. oligosporus-produced β-glucosidase during the SSF of black soybeans can hydrolyze the phenol-bound glucosyl group and release free phenolics to improve the phenolic content of black soybeans. Zambrano et al.53 stated that apples and grapes fermented with Rhizomucor miehei can produce β-glucosidase, which contributes to the release of phenolic compounds from the substrates. As shown in Fig. 3B, linear correlation analysis demonstrated a closely positive relationship (R2 = 0.9559, p < 0.01) between β-glucosidase and TPC. Therefore, the highly produced β-glucosidase observed in the present study was strongly involved in the liberation of phenolic compound in the fermented soybeans. Although cellulase activity was low during SSF with E. cristatum, it enhanced with the increase in fermentation time. Correlation analysis also revealed that the phenolic mobilization of soybeans during SSF was closely associated (R2 = 0.9783, p < 0.01) with cellulase activity, as shown in Fig. 3C, which coincided with some previous studies. For instance, Wang et al.50 stated that cellulase is closely related to the mobilization of phenolic compounds during guava leaf tea fermentation with Monascus anka and Bacillus sp. Protease activity was also determined in the present study, and the result is shown in Fig. 2D. The result revealed that the protease activity was at a moderate level similar to α-amylase activity, steadily increased between days 2 and 8 (ranging from 24 U g−1 to 51 U g−1), and then rapidly increased to a maximum value of 75 U g−1 after day 10 of solid-state bioprocessing. However, the protease activity decreased at the later period of fermentation (day 15), which was possibly due to the enzyme feedback regulated or inhibited immediately by the increased amount of phenolic or enzyme denaturation and/or decomposition.14,54 The protease produced during fermentation was effectively involved in the mobilization of phenolic compounds, which can contribute to the enhancement of TPC.35 Fig. 3D shows that protease activity was significantly correlated (p < 0.05) with the liberation of phenolics, whereas R2 (0.6785) was much lower than those in the three carbohydrate-cleaving enzymes.
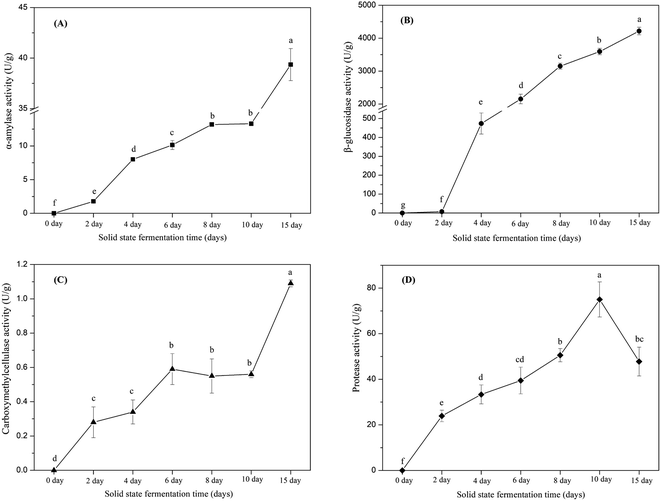 |
| Fig. 2 (A) α-Amylase activity, (B) β-glucosidase activity, (C) carboxymethylcellulase activity and (D) protease activity of soybean substrate during solid-state bioprocessing by E. cristatum. Data were presented as the mean value ± standard deviation of triplicate experiments. Mean values marked by the different letters were significant (p < 0.05) difference among the samples. | |
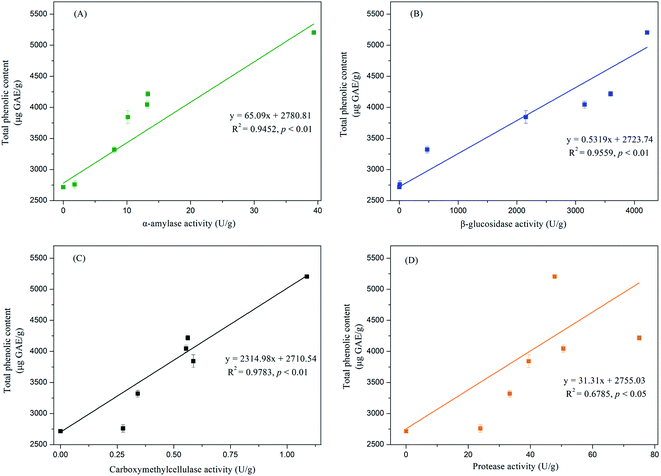 |
| Fig. 3 Line relationship among changes of total phenolics content and the activities of (A) α-amylase, (B) β-glucosidase, (C) carboxymethylcellulase and (D) protease during solid-state bioprocessing by E. cristatum. | |
As mentioned above, the hydrolytic enzymes (i.e., β-glucosidase, α-amylase, cellulase, and protease) produced by E. cristatum played a prominent role in liberating phenolics from the soybean substrate. In summary, the role of hydrolytic enzymes in the mobilization of soybean phenolics during SSF with E. cristatum is as follows. First, the cellulase produced by the fungus decomposed the plant cell wall structure, leading to the release of phenolic compounds.6,43 Second, cellulase, α-amylase, and β-glucosidase produced by E. cristatum hydrolyzed the covalent bond between the insoluble-bound phenolic and starches, cellulose, hemicellulose, lignin, and other polysaccharide structures; thus, the insoluble-bound phenolics were bioconverted into soluble phenolic fractions prior to extraction.6,48 Third, the β-glucosidase produced by E. cristatum catalyzed the glycosidic linkage hydrolysis in alkyl and aryl β-D-glucosides and the glycosides containing merely carbohydrate residues. As a result, the corresponding flavonoid aglycone compounds, such as daidzein and genistein, could be released. Oboh et al.55 stated that protease can efficiently convert protein-bound polyphenols into free phenolics; thus, protease is possibly one of the contributors to the enhancement of phenolics during fermentation.
3.2 Changes in isoflavone compositions of soybeans during SSF and its relationship with β-glucosidase activity
In the present study, four major isoflavones, including genistin, genistein, daidzin, and daidzein, in the soybean extracts during SSF were identified and qualified by HPLC, as shown in Fig. 4 and Table 1. The quantity of glucoside isoflavones significantly decreased as SSF progressed, with the largest reduction observed after 15 days of bioprocessing. At the late period (day 15) of bioprocessing, the contents of daidzin, genistin, and total glucosides were significantly decreased to 150 ± 18, 361 ± 9, and 511 ± 26 μg g−1, respectively. By contrast, aglycone isoflavone concentration in fermented soybeans remarkably increased during SSF. As illustrated in Table 1, daidzein, genistein, and total aglycones were significantly increased (p < 0.05) to 777 ± 12, 669 ± 11, and 1446 ± 23 μg g−1 after 15 days of fermentation, which were approximately 10.4, 8.4, and 9.4-fold higher, respectively, than those in non-fermented soybeans. The accumulation of flavonoid aglycones via fermentation techniques has been reported by previous investigators. For instance, Lee et al.15 reported that SSF with Tricholoma matsutake also contributes to the enhancement of isoflavone aglycones in soybeans. Santos et al.37 demonstrated that soybean okara processed by SSF with Saccharomyces cerevisiae significantly (p < 0.05) increases daidzein and genistein. Gu et al.30 recently reported that the glucoside flavonoid (i.e., rutin) of Hippophae rhamnoides leaves are bio-transformed into aglycone flavonoid (i.e., quercetin, kaempferol, and isorhamnetin) by SSF with Eurotium amstelodami. Previous studies have attributed the enhancement of aglycone forms to the action of β-glucosidase produced by inoculated fungal mycelia, which can catalyze the hydrolysis of flavonoid glucosides into their corresponding aglycone forms during fermentation.15 Gu et al.30 verified that glucoside flavonoid geniposide is converted into its corresponding aglycone genipin by Eurotium sp. for the produced β-glucosidase, which catalyzes the hydrolysis of the glycosidic bond between genipin and glucose, thus releasing the aglycone flavonoid genipin.
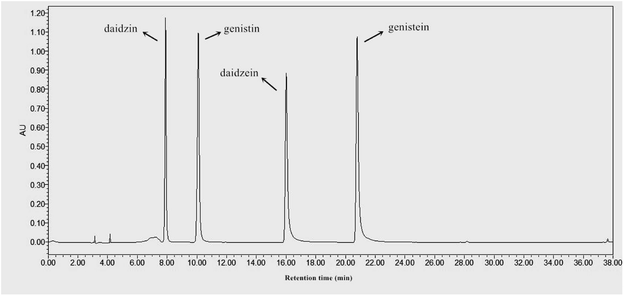 |
| Fig. 4 High performance liquid chromatographic chromatogram of the four major isoflavones (daidzin, daidzein, genistin and genistein) detected in the soybeans and soybeans bioprocessed by E. cristatum. | |
Table 1 HPLC results of the biotransformation of glucosides isoflavone to aglycones during soybean bioprocessed with E. cristatum. Isoflavones content were presented as μg g−1 d.w.a
Isoflavone isomers |
Solid state fermentation time (days) |
0 day |
2 day |
4 day |
6 day |
8 day |
10 day |
15 day |
Each value was represented as the mean value ± standard deviation of three replicates. Mean values followed by different superscript letters in the same row denoted significant difference (p < 0.05) to each other. |
Glucosides |
Daidzin |
730 ± 19a |
724 ± 20a |
644 ± 17b |
597 ± 14c |
475 ± 11d |
384 ± 11e |
150 ± 18f |
Genistin |
954 ± 9a |
940 ± 10a |
890 ± 2b |
873 ± 19b |
793 ± 19c |
685 ± 19d |
361 ± 9e |
Total glucosides |
1685 ± 24a |
1664 ± 25a |
1534 ± 19b |
1471 ± 29c |
1268 ± 29d |
1069 ± 22e |
511 ± 26f |
![[thin space (1/6-em)]](https://www.rsc.org/images/entities/char_2009.gif) |
Aglycones |
Daidzein |
74 ± 6f |
84 ± 3f |
149 ± 3e |
248 ± 5d |
303 ± 5c |
497 ± 16b |
777 ± 12a |
Genistein |
79 ± 3f |
85 ± 6f |
128 ± 6e |
212 ± 5d |
269 ± 6c |
359 ± 10b |
669 ± 11a |
Total aglycones |
154 ± 4f |
170 ± 7f |
277 ± 9e |
459 ± 10d |
572 ± 5c |
856 ± 24b |
1446 ± 23a |
Correlation analysis was performed to obtain the precise interrelationship between the change in isoflavone compositions and β-glucosidase activity, and the results are exhibited in Fig. 5. Daidzin, genistin, and total glucosides were highly negatively correlated with β-glucosidase activity (R2 = 0.8072, 0.8698, and 0.7834, respectively, p < 0.01). By contrast, β-glucosidase activity displayed highly positive correlations with the contents of aglycone isoflavones (i.e., daidzein, genistein, and total aglycones), with R2 values reaching 0.7676, 0.7759, and 0.8393 (p < 0.01), respectively. Therefore, β-glucosidase was strongly involved in the bioconversion of glucoside isoflavones into their corresponding aglycone forms. The remarkable enhancement of aglycone isoflavone in the bioprocessed soybean by E. cristatum mycelia can be ascribed to the obvious increase in β-glucosidase activity during SSF. This result is in agreement with those of many previous investigations that β-glucosidase plays a crucial role in the transformation of isoflavone glucosides into aglycones during SSF.15 The increased total isoflavone aglycones was more than the reduced total isoflavone glucosides (Table 1), which can be attributed to some isoflavone aglycones being synthesized or bio-transformed into other compounds by E. cristatum during fermentation. Further research is needed to perform on how isoflavone aglycones are synthesized during SSF with E. cristatum.
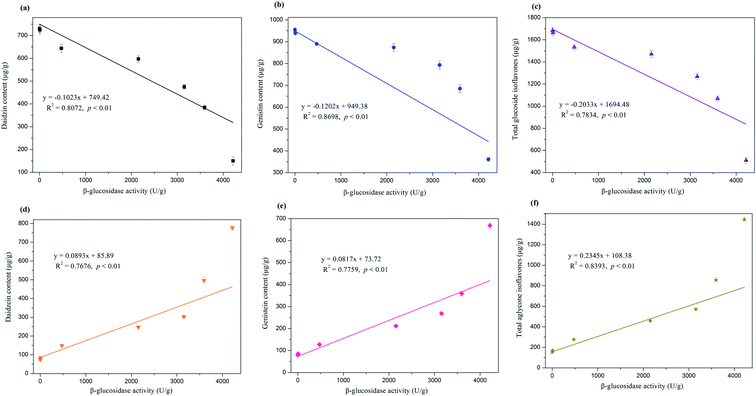 |
| Fig. 5 Relationship among change of glucoside isoflavones (a–c), aglycone isoflavones (d–f) and β-glucosidase activity during solid-state bioprocessing by E. cristatum. | |
Aglycone flavonoids exhibit stronger anticancer, antioxidant, and anti-atherosclerotic effects than glucosides.22,56 Ahmad et al.24 and Lee et al.15 demonstrated that isoflavone aglycones display stronger antioxidant property than glucosides. In addition, Kim et al.56 illustrated that the side glycosidic linkage of ginsenoside is broken down by the action of β-glucosidase, which then enhances its anticancer activity. Thus, the solid-state bioprocessed soybeans using E. cristatum enhances isoflavone aglycones, which may be applied to the commercial production of nutraceuticals and functional foods.
3.3 Changes in antioxidant activity of soybeans during SSF
In recent years, there have been many methods to evaluate the antioxidant effects of complex food constituents due to the distinctive antioxidant mechanism of different antioxidant compounds. The specificity and sensitivity of one method does not adequately detect all antioxidant compounds in the studied sample. Consequently, it is very essential to use a series of approaches for the antioxidant evaluation of samples to take into consideration of the different mechanisms of antioxidants. In the present investigation, four common antioxidant property assays with different mechanisms were carried out to assess the change of antioxidant activity of soybeans during SSF, and the results are illustrated in Fig. 6A–D.
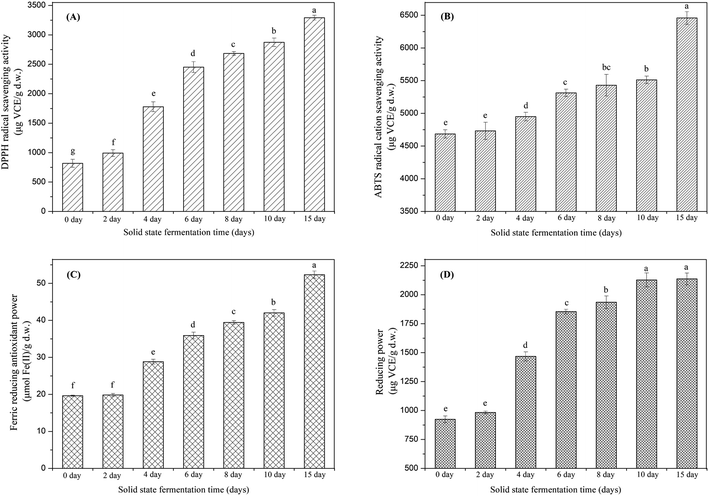 |
| Fig. 6 Effect of solid-state bioprocessing by E. cristatum on the DPPH radical scavenging activity (A), ABTS˙+ scavenging activity (B), ferric reducing antioxidant power (C) and reducing power of soybeans. Data were recorded as the mean value ± standard deviation of three replicates. Mean values marked by the different letters among the samples denoted significant difference (p < 0.05). | |
3.3.1 DPPH radical scavenging activity. DPPH is stable organic free radical which generally applied for the assessment of in vitro antioxidant property. The distinct purple color of DPPH radical solution disappeared as it reacts with antioxidants which prevent the radical chain reaction by providing hydrogen atoms. In this study, the scavenging activity against DPPH radical of fermented (days 2–15) and non-fermented soybeans (day 0, control) is shown in Fig. 6A. The result revealed that days of fermentation displayed a significant (p < 0.01) influence on DPPH radical scavenging activity. It was noted that DPPH radical scavenging activity changed slightly at the early growth phase (from day 0 to day 2), and then started to rapidly increase from day 4 onwards. DPPH radical scavenging activity enhanced about 4 times at the late periods of SSF (day 15). In detail, during the different bioprocessed periods (days 0, 2, 4, 6, 8, 10, and 15), the values of scavenging activity against DPPH radical of soybeans were 818, 992, 1779, 2453, 2684, 2874, and 3292 μg VCE per g, respectively. SSF's ability to remarkably enhance the scavenging activity against DPPH radical of the soybean samples was also elucidated in earlier literatures. For example, Lee et al.15 and Santos et al.37 have verified that soybean samples processed by SSF with Tricholoma matsutake and Saccharomyces cerevisiae, respectively, significantly enhanced its scavenging activity against DPPH radical and the magnitude enhancement of the antioxidant activity depended on the fermentation time, which is in consistence with the result from our study. It was well illustrated in earlier researches that the augment of scavenging activity against DPPH radical of fermented samples was mainly associated with the released phenolic compounds during SSF process. In addition, the structure of phenolic compounds would also significantly influence the radical scavenging activity, which is widely reported in previous studies. In general, polyphenols are more efficient as antioxidants than monophenols, and the monophenols antioxidant activity can also be enhanced by methoxy substitution, however, this effect is less important than a hydroxyl group addition.57 Furthermore, Gu et al.30 have also stated that the phenolic compound derivatives (e.g., flavonoids derivatives) having a hydroxyl group which substituted on the C-ring were noted to exhibit higher radical scavenging activity. The chemical structures of the phenolic compounds might be changed by SSF, which would significantly influence the antioxidant activity of the fermented samples. In fact, this study found that glucosides isoflavone (a special kind of phenolic compounds) were transformed into aglycones isoflavone of soybeans via processed with E. cristatum mycelia, thus a hydroxyl group which in the C-7 position of the C-ring was obtained after SSF. Accordingly, the enhanced DPPH radical scavenging activity might be caused by the increase of aglycones isoflavone during SSF. Furthermore, Zhang and Yu23 and Santos et al.37 have reported that soybeans processed with microorganisms promoted the biotransformation of soybean isoflavone from glucosides to aglycones, which contribute to the enhanced antioxidant activity of products during SSF.Hence, as discussed above, in order to further elucidate the precise relationship between TPC, glucosides isoflavones, aglycone isoflavones and antioxidant activity, Pearson's correlation analysis was conducted, and the results are displayed in Table 2. It was revealed that there was a highly positive correlation (p < 0.01) between TPC, aglycone isoflavones and DPPH radical scavenging activity (TPC, r = 0.961; daidzein, r = 0.877; genistein, r = 0.855; total aglycones, r = 0.868). Thus, it seems that the improved scavenging activity against DPPH radical of fermented soybeans mainly attributed to the enhancement of phenolic compounds as well as the isoflavone aglycones (e.g., daidzein and genistein) formed during SSF process.
Table 2 Pearson's correlation coefficients among total phenolics content (TPC), contents of glucoside isoflavones and aglycone isoflavones, and antioxidant activitya,b
|
TPC |
Daidzin |
Genistin |
TGI |
Daidzein |
Genistein |
TAI |
DPPH |
ABTS |
FRAP |
RP |
DPPH, DPPH radical scavenging activity; ABTS, ABTS˙+ scavenging activity; FRAP, ferric reducing antioxidant power; RP, reducing power; TGI, total glucoside isoflavones; TAI, total aglycone isoflavones. **Correlation was significant at the 0.01 level (two-tailed). |
TPC |
1.000 |
−0.969** |
−0.927** |
−0.954** |
0.959** |
0.957** |
0.960** |
0.961** |
0.975** |
0.993** |
0.920** |
Daidzin |
|
1.000 |
0.977 |
0.994** |
−0.990** |
−0.986** |
−0.990** |
−0.898** |
−0.967** |
−0.958** |
−0.849** |
Genistin |
|
|
1.000 |
0.994** |
−0.983** |
−0.992** |
−0.989** |
−0.808** |
−0.960** |
−0.898** |
−0.741** |
TGI |
|
|
|
1.000 |
−0.993** |
−0.995** |
−0.996** |
−0.858** |
−0.969** |
−0.933** |
−0.800** |
Daidzein |
|
|
|
|
1.000 |
0.992** |
0.998** |
0.877** |
0.966** |
0.942** |
0.826** |
Genistein |
|
|
|
|
|
1.000 |
0.998** |
0.855** |
0.978** |
0.932** |
0.791** |
TAI |
|
|
|
|
|
|
1.000 |
0.868** |
0.973** |
0.939** |
0.812** |
DPPH |
|
|
|
|
|
|
|
1.000 |
0.896** |
0.980** |
0.986** |
ABTS |
|
|
|
|
|
|
|
|
1.000 |
0.954** |
0.839** |
FRAP |
|
|
|
|
|
|
|
|
|
1.000 |
0.953** |
RP |
|
|
|
|
|
|
|
|
|
|
1.000 |
3.3.2 ABTS˙+ scavenging activity. ABTS˙+ scavenging effect is applicable for both hydrophilic and antioxidants assay. ABTS is associated with the scavenging abilities of hydrogen donating and chain breaking antioxidants, which has documented in previous literatures.12 Scavenging effects of the fungus treated soybeans were evaluated and compared with the non-fermented counterpart. ABTS˙+ scavenging effect of soybeans changed depending on the SSF bioprocessing times as shown in Fig. 6B. However, at early stage (days 0–2), there was no significant difference for the activity which suggested that E. cristatum might not have entirely grown and biotransformation of soybean substance within 2 days. With the fermentation times increased (days 4–10), steady increasing of ABTS˙+ scavenging effects was observed. After 15 days of fermentation, remarkably elevated ABTS˙+ scavenging activity was observed for fermented soybeans, the increase rates was approximately 37.8% when compares with the non-fermented soybeans (day 0). This increase tendency was in agreement with the enhancement of TPC and isoflavone aglycones results obtained in the present study (Table 1). Table 2 shows that TPC, isoflavone aglycones and ABTS˙+ scavenging effect of soybean fractions were greatly correlated (r > 0.9, p < 0.01), which providing strong evidence that the improved ABTS˙+ scavenging effect noted might be ascribed to the augment of phenolics and isoflavone aglycones during SSF.
3.3.3 Ferric reducing antioxidant power (FRAP). FRAP was conducted to assay the ability of antioxidants that result in TPTZ–Fe3+ complex compound reducing to the TPTZ–Fe2+ form. The FRAP changes trend of soybeans during SSF process by E. cristatum are shown in Fig. 6C, which is like ABTS˙+ scavenging activity. The FRAP of fermented soybeans significantly increased with the fermentation time increased except for the early fermentation periods (day 0–2). Highest FRAP (52 μmol Fe(II) per g d.w.) of the bioprocessed soybeans was observed after 15 days of fermentation, which is approximately 3 times when in comparison with the non-fermented soybeans (19.7 μmol Fe(II) per g d.w.). This enhancement is most likely attributed to the liberation of phenolic from the matrices by the fungus. Solid-state bioprocessing with filamentous fungus improved the FRAP of sample was also verified in previous investigations. Razak et al.58 and Đorđević et al.39 have reported that rice bran fermented with R. oligosporus and M. purpureus, barley fermented with Lactobacillus rhamnosus and Saccharomyces cerevisiae significantly enhanced FRAP in comparison of their non-fermented counterpart, and both of them attributed to the released phenolics during SSF. In terms of this study, total phenolics content displayed highly positive correlation with FRAP, with the coefficient r values reaching 0.993 (p < 0.01). Besides, it was worth nothing that isoflavone aglycones also showed good correlations with FRAP concurrently (r > 0.9, p < 0.01). Therefore, it can be concluded that released phenolics and produced isoflavone aglycones via biotransformation of isoflavone glucosides during SSF were responsible for the increase of FRAP.
3.3.4 Reducing power (RP). The reducing power of fermented (days 2–15) and non-fermented soybeans (day 0) is shown in Fig. 6D. It was revealed that slight increases in the RP at the early period of SSF (days 0–2), but the increment is insignificant (p > 0.05), which is like ABTS˙+ scavenging effect and FRAP. However, with the proceeding of SSF (days 4–10), it was clearly found that RP enhanced significantly (p < 0.05) and thereafter the values remained constant for the late period of SSF (days 10–15). The results observed form RP demonstrated that as E. cristatum initially adapted itself according to surrounding conditions, RP value initially increased slightly and thereafter significantly enhanced till 10th day of SSF followed by remaining constant. Specifically, after 10 days of SSF, RP value of fermented soybeans is 2128 μg VCE per g, which is about 2.3 folds higher compared to the non-fermented soybeans (924 μg VCE per g). Earlier reports have also show that the RP value of cereal samples (e.g., oat, wheat, millet, sorghum, corn, rice and broomcorn millet) were significantly enhanced by solid-state fermentation with Agaricus blazei fungus.59 The enhanced RP revealed might be due to the increased phenolics during SSF (correlation coefficient r = 0.920, p < 0.01, Table 2), which could react with free radicals to stabilize and break radical chain reactions,36 consequently transforming them to more stable products. Besides, improvement of isoflavones aglycones during SSF is also partially responsible for the enhanced RP, in which the correlation analysis also displayed that they were remarkably correlated (r > 0.75, p < 0.01, Table 2). These explanations could also be proved by earlier studies that attributed to the enhanced RP to the increase of TPC and isoflavones aglycones during fermentation.24,37
3.4 Principal component analysis (PCA) of hydrolytic enzymes activities, total phenolics content, isoflavones and antioxidant activities
It has been well reported that phenolics and isoflavones were mainly contributed to the antioxidant activities of soybean samples. In the present study, the enhancement of phenolic compounds and isoflavone aglycones were mainly ascribed to the produced carbohydrate-cleaving enzymes (i.e., β-glucosidase, α-amylase and cellulase) and protease by E. cristatum during SSF process. Principal component analysis (PCA) is widely conducted by food scientists/researchers to evaluate the relationship of bioactive compounds and antioxidant activities of studied samples.36 Hence, in order to get an overview of the correlation between the hydrolysis enzyme activities and the change of phenolics and isoflavone content, as well as its subsequent closely effect on the enhanced antioxidant activities of soybeans during SSF, principal component analysis (PCA) model was created in this study, and the results are elucidated in Fig. 7. Fig. 7A shows the PCA loading plot of each evaluation index. The result revealed that the first two validated principal components (PCs) attributed to 97.09% of the total information in terms of the original variables, implying that the first two PCs could well reflect the information of the original variables. The PCA loading plot not only could clarify the how well the PCs related with original variables, but also reflect the relationship between the studied variables. The close position of the indices in the PCA loading plot is an indicative of the high relationship between these indices,57 which can described by the same PC. In contrast, less or no correlated indices were explained by different PCs. As illustrated in Fig. 7A, RP, DPPH, FRAP, ABTS, TPC, isoflavone aglycones were well correlated with PC1 and was noted to be highly similar loaded on PC1 as well, which suggests that RP, DPPH, FRAP, ABTS and TPC, isoflavone aglycones exhibited remarkably positive relationship. This result observed by PCA is coincided with the phenomenon obtained by the Pearson's correlation analysis (Table 2). Thereafter, as described above, PCA result further demonstrated that the increased antioxidant activity of soybeans was primarily attributed to the improvement of TPC and aglycone isoflavones during SSF process.
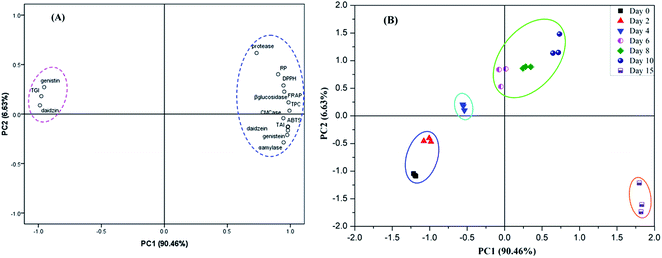 |
| Fig. 7 (A) Principal component analysis (PCA) loading plot of hydrolytic enzymes, total phenolic content (TPC), contents of glucoside isoflavones and aglycone isoflavones, and antioxidant activity of soybean during solid-state bioprocessing by E. cristatum. (B) PCA score plot of soybeans with different bioprocessing period with E. cristatum. | |
Besides, as seen from Fig. 7A, TPC, aglycone isoflavones and all the hydrolytic enzymes were observed to be similarly loaded on PC1, implying that the increase of phenolic and aglycone isoflavones content during SSF process might be due to the produced hydrolytic enzymes by E. cristatum, which could catalyze the liberation of free phenolics and aglycones from the soybeans substrate, hence resulting in an significant enhancement in their antioxidant potential. Furthermore, it was obviously noted that aglycone isoflavones (i.e., daidzein, genistein and TAI) was inversely displayed with glucoside isoflavones (daidzin, genistin and TGI) in light of the direction of the variables, which implied that the hydrolysis of this conjugated glucoside isoflavones and subsequently contributing to the liberation of aglycone isoflavones during SSF.
The score plot of PCA was also applied to investigate the differences and similarities of antioxidant activities among soybean samples at the different time points of fermentation, and the results are shown in Fig. 7B. The distance between the points of different samples on the scores plot demonstrated that the degree of similarity or variability of the antioxidant properties for these samples. According to Fig. 7B, it can be clearly found that the scores of the soybean samples moved from the negative axis to the positive axis of PC1 with the proceeding of SSF. Furthermore, it worth noting that all of the soybean samples could be divided into four stages along with the vector of PC1 from left to right. It can be revealed in Fig. 7B that 2 day fermented soybeans and non-fermented soybeans (day 0) are distributed in the left of PC1 and having high negative score, which can be interpreted that the bioactive compounds and antioxidant activity of these samples are lower. Besides, it was observed that close relationships among soybeans fermented with 6, 8 and 10 days, which suggested that these samples have similar TPC, aglycone isoflavones content and antioxidant activity. Furthermore, 15 day fermented soybeans exhibited great different distribution with respect to the other soybean samples and having high positive score of PC1, which indicated that these soybean samples could be best depicted as having great bioactive compounds and antioxidant activity. The trends of this change explanation could also further support by the results illustrated in Table 1, Fig. 1 and 6. Thus, these results confirmed that fermentation time played an important role on the phenolic compounds, isoflavones composition and antioxidant activity variation of the soybean samples, which was in accordance with the previous studies published earlier.44,60 Hence, this finding implied that PCA could be useful to provide crucial insight into the discrimination and classification of the soybean samples investigated, along with the relationships between TPC, isoflavone and antioxidant activity.
4. Conclusions
The current study demonstrated that E. cristatum used for SSF was very effective for the enhancement of TPC, aglycone isoflavone, scavenging activity of DPPH radical and ABTS˙+, FRAP and RP of soybeans studied. Carbohydrate cleaving enzymes (i.e., α-amylase, β-glucosidase and CMCase) and protease were produced by E. cristatum during soybean fermentation. β-Glucosidase activity was very high whereas α-amylase as well as protease activity was at moderate levels, and cellulase (CMCase) was low during the fermentation periods. Carbohydrate cleaving enzymes, TPC, aglycone isoflavone as well as antioxidant activity remarkably increased with the proceeding of the fermentation times. There was a significant correlation between the improvement of TPC and the activities of α-amylase, β-glucosidase, cellulase and protease during SSF process. More importantly, β-glucosidase produced by E. cristatum was strongly involved in the biotransformation of isoflavone glucosides of soybeans into their corresponding aglycone forms. Both PCA and Pearson's correlation analysis verified that the augment of antioxidant activities of soybean samples during SSF were mainly attributed to the improvement of TPC and isoflavones aglycone. Thus, we demonstrated for the first time E. cristatum mycelia bioprocessed soybeans significantly improved its TPC, aglycone isoflavone and antioxidant activity, which might be utilized in the production of novel functional foods or nutraceuticals compared to the non-fermented soybean. Further work is needed to estimation their potential human health promotion effects in E. cristatum bioprocessed soybeans with regards to the development of nutraceuticals or functional foods.
Abbreviations
SSF | Solid-state fermentation |
TPC | Total phenolics content |
HPLC | High-performance liquid chromatography |
TAI | Total aglycone isoflavones |
TGI | Total glucoside isoflavones |
ABTS | 2,2-Azinobis(3-ethylbenzothiazoline-6-sulphonic acid)diammonium salt |
DPPH | 2,2-Diphenyl-1-picrylhydrazyl |
Vitamin C | Ascorbic acid |
RP | Reducing power |
FRAP | Ferric reducing antioxidant power |
CMCase | Carboxymethylcellulase |
PCA | Principal component analysis |
Conflicts of interest
The authors declare there are no conflicts of interest.
Acknowledgements
This work was financial supported by Natural Science Youth Foundation of Hunan Agricultural University (No. 19QN22) and Hunan Agricultural University Research Startup Fund. The authors also thank the Hunan Agricultural University Undergraduate Students Innovation Training Project (No. xcx19103), Hainan Province Science and Technology Major Project (No. ZDKJ2016003) and Hunan Province Science and Technology Innovation Plan Project (No. 2017SK2430). This work was also supported by Excellent Youth Foundation of Education Department of Hunan Province (No. 19B269).
References
- T. Aruna, Innovative Food Sci. Emerging Technol., 2019, 57, 102193 CrossRef CAS.
- O. A. Adebo, P. B. Njobeh, J. A. Adebiyi, S. Gbashi, J. Z. Phoku and E. Kayitesi, Functional Food—Improve Health through Adequate Food, ed. M. C. Hueda, 2017, pp. 77–109 Search PubMed.
- H.-Y. Shin, S.-M. Kim, J. H. Lee and S.-T. Lim, Food Chem., 2019, 272, 235–241 CrossRef CAS PubMed.
- S. N. Bhowmik and R. T. Patil, in Crop Improvement Through Microbial Biotechnology, Elsevier, 2018, pp. 73–106 Search PubMed.
- R. D. Divate, C.-C. Wang, S.-T. Chou, C.-T. Chang, P.-M. Wang and Y.-C. Chung, LWT--Food Sci. Technol., 2017, 81, 18–25 CrossRef CAS.
- T. B. Dey, S. Chakraborty, K. K. Jain, A. Sharma and R. C. Kuhad, Trends Food Sci. Technol., 2016, 53, 60–74 CrossRef.
- E. Rosales, M. Pazos and M. Á. Sanromán, in Current Developments in Biotechnology and Bioengineering, Elsevier, 2018, pp. 319–355 Search PubMed.
- C. Torres-León, N. Ramirez-Guzman, J. Ascacio-Valdes, L. Serna-Cock, M. T. dos Santos Correia, J. C. Contreras-Esquivel and C. N. Aguilar, LWT, 2019, 112, 108236 CrossRef.
- W. C. Vong, X. Y. Hua and S.-Q. Liu, LWT, 2018, 90, 316–322 CrossRef CAS.
- S. Martins, S. I. Mussatto, G. Martínez-Avila, J. Montañez-Saenz, C. N. Aguilar and J. A. Teixeira, Biotechnol. Adv., 2011, 29, 365–373 CrossRef CAS.
- R. Y. Gan, H. B. Li, A. Gunaratne, Z. Q. Sui and H. Corke, Compr. Rev. Food Sci. Food Saf., 2017, 16, 489–531 CrossRef CAS.
- K. S. Sandhu, S. Punia and M. Kaur, LWT--Food Sci. Technol., 2016, 71, 323–328 CrossRef CAS.
- R. K. Salar, S. S. Purewal and K. S. Sandhu, Food Res. Int., 2017, 100, 204–210 CrossRef CAS PubMed.
- T. Bhanja, A. Kumari and R. Banerjee, Bioresour. Technol., 2009, 100, 2861–2866 CrossRef CAS PubMed.
- J. H. Lee, C. E. Hwang, K. S. Son and K. M. Cho, Food Chem., 2019, 272, 362–371 CrossRef CAS.
- T. D. Oluwajuyitan and O. S. Ijarotimi, Heliyon, 2019, 5, e01504 CrossRef PubMed.
- I.-M. Chung, S.-H. Seo, J.-K. Ahn and S.-H. Kim, Food Chem., 2011, 127, 960–967 CrossRef CAS PubMed.
- S. Natarajan, D. Luthria, H. Bae, D. Lakshman and A. Mitra, J. Agric. Food Chem., 2013, 61, 11736–11743 CrossRef CAS PubMed.
- K. Zaheer and M. Humayoun Akhtar, Crit. Rev. Food Sci. Nutr., 2017, 57, 1280–1293 CrossRef CAS PubMed.
- W. Szeja, G. Grynkiewicz and A. Rusin, Curr. Org. Chem., 2017, 21, 218–235 CrossRef CAS PubMed.
- L. Chen, H. Teng and J. Xiao, Food Sci. Human Wellness, 2019 Search PubMed.
- T. Izumi, M. K. Piskula, S. Osawa, A. Obata, K. Tobe, M. Saito, S. Kataoka, Y. Kubota and M. Kikuchi, J. Nutr., 2000, 130, 1695–1699 CrossRef CAS PubMed.
- H. Zhang and H. Yu, J. Food Biochem., 2019, 43, e12804 CrossRef PubMed.
- A. Ahmad, K. Ramasamy, A. B. A. Majeed and V. Mani, Pharm. Biol., 2015, 53, 758–766 CrossRef CAS PubMed.
- Z. Liu, L. Gao, Z. Chen, X. Zeng, J. Huang, Y. Gong, Q. Li, S. Liu, Y. Lin and S. Cai, Nature, 2019, 566 Search PubMed.
- Q. Li, J. Huang, Y. Li, Y. Zhang, Y. Luo, Y. Chen, H. Lin, K. Wang and Z. Liu, Sci. Rep., 2017, 7, 6947 CrossRef PubMed.
- Q. Li, Y. Li, Y. Luo, Y. Zhang, Y. Chen, H. Lin, K. Wang, J. Huang and Z. Liu, Food Microbiol., 2019, 80, 70–76 CrossRef CAS PubMed.
- Y. Yao, M. Wu, Y. Huang, C. Li, X. Pan, W. Zhu and Y. Huang, LWT--Food Sci. Technol., 2017, 82, 248–254 CrossRef CAS.
- L. Cao, X. Guo, G. Liu, Y. Song, C.-T. Ho, R. Hou, L. Zhang and X. Wan, J. Food Drug Anal., 2018, 26, 112–123 CrossRef CAS PubMed.
- Q. Gu, G. Duan and X. Yu, Microorganisms, 2019, 7, 122 CrossRef CAS PubMed.
- C. Jiang, Z. Zeng, Y. Huang and X. Zhang, LWT, 2018, 98, 204–211 CrossRef CAS.
- S.-D. Zhou, X. Xu, Y.-F. Lin, H.-Y. Xia, L. Huang and M.-S. Dong, Food Chem., 2019, 272, 670–678 CrossRef CAS PubMed.
- R. K. Salar and S. S. Purewal, Biocatal. Agric. Biotechnol., 2016, 8, 221–227 CrossRef.
- S. Ang, E. Shaza, Y. Adibah, A. Suraini and M. Madihah, Process Biochem., 2013, 48, 1293–1302 CrossRef CAS.
- G. Chen, Y. Liu, J. Zeng, X. Tian, Q. Bei and Z. Wu, J. Cereal Sci., 2020, 102940 CrossRef CAS.
- Y. Xiao, X. Rui, G. Xing, H. Wu, W. Li, X. Chen, M. Jiang and M. Dong, J. Funct. Foods, 2015, 16, 58–73 CrossRef CAS.
- V. A. Q. Santos, C. G. Nascimento, C. A. Schmidt, D. Mantovani, R. F. Dekker and M. A. A. da Cunha, LWT, 2018, 92, 509–515 CrossRef.
- Y. Xiao, G. Xing, X. Rui, W. Li, X. Chen, M. Jiang and M. Dong, J. Funct. Foods, 2014, 10, 210–222 CrossRef CAS.
- T. M. Đorđević, S. S. Šiler-Marinković and S. I. Dimitrijević-Branković, Food Chem., 2010, 119, 957–963 CrossRef.
- B. A. Acosta-Estrada, J. Villela-Castrejón, E. Perez-Carrillo, C. E. Gómez-Sánchez and J. A. Gutiérrez-Uribe, J. Cereal Sci., 2019, 90, 102837 CrossRef CAS.
- Y. Ma, Y. Wu, J. Yang, H. Liang and F. Shang, Bangladesh J. Bot., 2017, 46, 907–915 Search PubMed.
- H. B. Singh, B. N. Singh, S. P. Singh and C. S. Nautiyal, Bioresour. Technol., 2010, 101, 6444–6453 CrossRef CAS PubMed.
- M. Kang, F.-H. Zhai, X.-X. Li, J.-L. Cao and J.-R. Han, J. Cereal Sci., 2017, 73, 138–142 CrossRef CAS.
- L. Wang, Y. Luo, Y. Wu and Z. Wu, J. Funct. Foods, 2018, 41, 183–190 CrossRef CAS.
- Q. Bei, G. Chen, F. Lu, S. Wu and Z. Wu, Food Chem., 2018, 245, 297–304 CrossRef CAS PubMed.
- B. A. Acosta-Estrada, J. A. Gutiérrez-Uribe and S. O. Serna-Saldívar, Food Chem., 2014, 152, 46–55 CrossRef CAS.
- S. Yeo and J. Ewe, in Advances in fermented foods and beverages, Elsevier, 2015, pp. 107–122 Search PubMed.
- S. J. Hur, S. Y. Lee, Y.-C. Kim, I. Choi and G.-B. Kim, Food Chem., 2014, 160, 346–356 CrossRef CAS.
- F. Shahidi and J. Yeo, Molecules, 2016, 21, 1216 CrossRef.
- L. Wang, J. Zhang, W. Zhang, X. Lin, C. Li and Z. Wu, Process Biochem., 2019, 82, 51–58 CrossRef CAS.
- K.-C. Cheng, J.-Y. Wu, J.-T. Lin and W.-H. Liu, Eur. Food Res. Technol., 2013, 236, 1107–1113 CrossRef CAS.
- J.-E. Sarry and Z. Günata, Food Chem., 2004, 87, 509–521 CrossRef CAS.
- C. Zambrano, A. Kotogán, O. Bencsik, T. Papp, C. Vágvölgyi, K. C. Mondal, J. Krisch and M. Takó, LWT, 2018, 89, 457–465 CrossRef CAS.
- F. Uyar and Z. Baysal, Process Biochem., 2004, 39, 1893–1898 CrossRef CAS.
- G. Oboh, A. Ademiluyi and A. Akindahunsi, Food Sci. Technol. Int., 2009, 15, 41–46 CrossRef CAS.
- J.-K. Kim, C.-H. Cui, Q. Liu, M.-H. Yoon, S.-C. Kim and W.-T. Im, Food Chem., 2013, 141, 1369–1377 CrossRef CAS PubMed.
- H. Zhao, J. Dong, J. Lu, J. Chen, Y. Li, L. Shan, Y. Lin, W. Fan and G. Gu, J. Agric. Food Chem., 2006, 54, 7277–7286 CrossRef CAS PubMed.
- D. L. A. Razak, N. Y. A. Rashid, A. Jamaluddin, S. A. Sharifudin and K. Long, Biocatal. Agric. Biotechnol., 2015, 4, 33–38 CrossRef.
- F.-H. Zhai, Q. Wang and J.-R. Han, J. Cereal Sci., 2015, 65, 202–208 CrossRef CAS.
- X.-Y. Zhang, J. Chen, X.-L. Li, K. Yi, Y. Ye, G. Liu, S.-F. Wang, H.-L. Hu, L. Zou and Z.-G. Wang, J. Funct. Foods, 2017, 32, 375–381 CrossRef CAS.
Footnote |
† These authors contributed equally to this work. |
|
This journal is © The Royal Society of Chemistry 2020 |