DOI:
10.1039/C9RA09256K
(Paper)
RSC Adv., 2020,
10, 2740-2746
Synthesis and dielectric properties of the eco-friendly insulating gas thiazyl trifluoride†
Received
7th November 2019
, Accepted 8th January 2020
First published on 15th January 2020
Abstract
Sulfur hexafluoride (SF6), which is known as a superior electrically insulating and arc-quenching medium, plays a decisive role in the modern transmission and distribution network of electric energy, especially in high-voltage power networks. However, the ever-increasing usage of SF6 also leads to the continuous escalation of atmospheric SF6 levels, which is considered to be the main cause of the greenhouse effect. To decrease this environmental impact, eco-friendly alternatives to SF6 have been researched for decades. To date, no significant success has been made regarding replacement gases for transmission networks. Some potential alternatives have comparatively lower global warming potential (GWP) but involve technical trade-offs. Thiazyl trifluoride, which has some excellent chemical and electric properties, is a novel substitution candidate for SF6. In this article, an efficient synthetic route starting from sulfur monochloride and followed by ammonization and fluorination was proposed. The structures of the intermediates and the target products were determined by X-ray diffraction (XRD), infrared spectroscopy (IR), and gas chromatography-mass spectrometry (GC-MS). The effects of some determining factors on the yield and purity, including the molar ratio of the reactants, recrystallization conditions and condensation temperature, were also investigated. The results showed that the overall yield of thiazyl trifluoride was approximately 25%, while the purity could be up to 90.6% under optimal conditions.
Introduction
SF6 is instrumental in achieving space-saving electrical energy transmission and distribution equipment, such as gas-insulated switchgears (GISs) and gas-insulated lines (GILs), due to its remarkable dielectric insulation and arc-quenching performance.1,2 According to the report of The Intergovernmental Panel on Climate Change (IPCC) in 2013, the global warming potential (GWP) of SF6 is approximately 23
000 times higher than that of CO2, and SF6 has a cumulative effect on global warming owing to its extreme chemical stability.3 The Kyoto protocol has identified SF6 as one of the six emission-limiting gases. Therefore, there is an urgent need to find a new environmentally friendly gas to replace SF6.4
In recent years, researchers have been committed to finding environmentally friendly alternatives to replace SF6; these alternatives should have low GWP, high dielectric strength and low boiling point.5,6 Significant efforts have been made to study insulation gases, and many researchers have made great progress using substitutes such as perfluorocarbons (e.g. c-C4F8, C3F8 (ref. 7)), hydrofluorocarbons, CF3I,8 C5F10O,9,10 C6F12O and C4F7N.11,12 These alternatives have higher insulation strength and lower GWP than SF6, but they also have different shortcomings in terms of boiling point, toxicity, stability, and other aspects. For example, although the insulation strength of c-C4F8 is approximately 1.25 times higher than that of SF6 and the GWP is far below that of SF6, the liquidus temperature of c-C4F8 is high (−8 °C), and carbon deposition will occur after discharge, which will affect equipment safety and insulation strength.13,14 Similarly, C4F7N has to be mixed with CO2 due to its high boiling point, which limits its insulation and arc-extinguishing performance.15
Thiazyl trifluoride (NSF3) is commonly known as a chemically stable, colorless gas that is used as an important precursor for the preparation of various sulfur–nitrogen–fluorine compounds. Recently, the results of a quantum chemical calculation based on a structure-activity relationship model show that NSF3 is most likely to be an insulating gas with a low greenhouse effect. Baoshan Wang et al.16 suggested that NSF3 has excellent performance parameters: the insulation strength is 1.35 times that of SF6 at the same pressure, and the GWP value is 916, which is approximately one-twentieth that of SF6, while the boiling point is −27 °C. Therefore, NSF3 has been theoretically proven to be a promising alternative gas considering its liquidus temperature, insulation performance, and greenhouse effect. Research on the synthetic route of this gas is a necessary precondition for testing the comprehensive performance of NSF3 in actual electrical equipment.
Few reports have described the synthesis of NSF3, and these studies have seldom focused on its electrical performance. William H. Kirchhoff17 reported the general protocol for the synthesis of NSF3 by using S4N4 and AgF2. However, Oskar Glemser18 argued that the numerous side products were difficult to separate; he also reported a synthesis method involving sulfur, ammonia and silver difluoride, with carbon tetrachloride as a solvent. Moreover, he suggested that the molar ratio between the reaction materials was 1
:
1
:
6 (NH3
:
S
:
AgF2). However, the byproducts of the reaction contained hydrogen fluoride gas, which is extremely corrosive and harmful to the environment.19 Clifford20 proposed a method in which NSF3 was prepared by SF2NCOF oxidation with silver difluoride. Generally, most related studies are more suitable for minor or trace synthesis rather than large-scale production.
To verify the insulation properties of NSF3 and obtain further insight for commercial production, a comparatively economical and efficient two-step route (Fig. 1) was proposed. Ammonia and sulfur monochloride (S2Cl2) were used as raw materials and were fluorinated by AgF2 to prepare NSF3. In addition, some decisive factors were studied to maximize the yield and purity.
 |
| Fig. 1 Two-step synthesis of NSF3 starting from sulfur monochloride. | |
Experimental methods
General remarks
Some of the chemicals used in this work are potentially dangerous due to their toxicity. Thus, the detailed information on the MSDS of the materials used in this experiment were determined according to the Chemical Book, and all toxic properties are described in Table S1 in the ESI.†
Material and methods
All solvents used were anhydrous and analytical grade. Sulfur monochloride (greater than or equal to 98%) was purchased from Shanghai Aladdin Bio-Chem Technology Company. Silver(II) fluoride was obtained from Sinopharm Chemical Reagent Co. Ltd. NH3 and nearly 99.99% pure nitrogen gas were obtained from Wuhan Xiangyun Industry and Trade Co. Ltd. The copper reactor and condenser were obtained from Shanghai Yanzheng Experimental Instrument Co., Ltd.
IR spectra were recorded on a Thermo FT-IR 5700 IR spectrometer (KBr), and Thermo Fisher DXR was used to record Raman spectra. GC-MS was carried out on a Varian-450 gas chromatograph coupled to a Varian-320 m selective detector equipped with EI detectors. The gas chromatograph was equipped with a 30 m and 0.250 mm, 0.25 mm df, VF-5 column. Photoelectron spectra and X-ray diffraction patterns were recorded on an X-ray photoelectron spectrometer (ESCALAB250Xi, Thermo Fisher Scientific, United States) and an X-ray diffractometer (XRD-6100, Shimadzu Corporation, Japan) with Cu-Kα radiation (λ = 0.154 nm).
Synthesis of tetrasulfur tetranitride (S4N4)
CCl4 (250 mL) and S2Cl2 (40 mL, 0.5 mol) were added to a 500 mL three-necked flask at 0 °C with stirring. Afterwards, dry NH3 was introduced into the three-necked flask at a flow rate of 1200 mL min−1. The whole reaction was carried out in an ice bath for 1 hour, resulting in the appearance of an orange precipitate in a red solution. The precipitate was washed with pure water with stirring for approximately 3 hours, isolated by Soxhlet extraction with dry dioxane, and then recrystallized from benzene to obtain pure S4N4 crystals. To generate S4N4 crystals, the red solution was transferred to a vacuum after evaporation treatment with dichloromethane and recrystallized with benzene.
The yield was 43%, and the crystal phase of S4N4 was further confirmed by XRD (as shown in Fig. 2). According to XPS, the percentage of S4N4 in the sample was above 99%. (N: 30.15%; S: 69.50%; C: 0.13%; O: 0.22%).
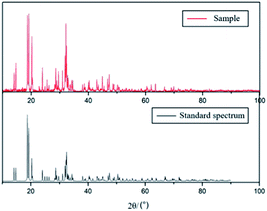 |
| Fig. 2 X-ray diffraction spectrum of S4N4. | |
Synthesis of thiazyl trifluoride
S4N4 (3.7 g, 0.02 mol) and CCl4 (80 mL) were added to a copper reactor equipped with a copper reflux condenser under N2 protection. The mixture was dissolved under magnetic stirring and heated to stable reflux. Then, AgF2 (45.7 g, 0.32 mol) was quickly added to the reflux. The whole reaction was carried out at 78 °C for 2 hours. The outlet of the condenser was connected by a conduit, and the gas product was successively dried by potassium permanganate solution and anhydrous calcium chloride and finally condensed to obtain crude NSF3 as the product. The purity of NSF3 was 90.6%, as determined by GC, while the structure was confirmed by IR and GC-MS.
GC/EI/MS (70 eV) m/z: 102.8 [M]−, 32 [S]+, 46.8 [NS]+, 65 [NSF]+, 69.9 [SF2]+, 83.9 [NSF2]+, 88.9 [SF3]+.
IR: 815 cm−1 (S–F), 945 cm−1 (N
S).
The insulation performance of thiazyl trifluoride
A high-voltage analysis platform was applied to evaluate the insulation performance of NSF3.21 The configuration of the circuit is shown in Fig. 3, and the parameters are shown in Table 1. The measurement accuracy was within ±1%.
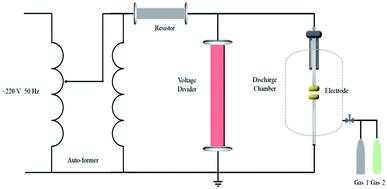 |
| Fig. 3 Configuration of the high-voltage analysis platform. | |
Table 1 The parameters of the high-voltage test device
Rated voltage |
Rated capacity |
Max output voltage |
Protective resistor |
Voltage divider |
220 V |
10 kVA |
100 kV |
5 kΩ |
1000 : 1 |
Two tungsten–copper alloy electrodes with diameters of 10 mm were employed as the test electrodes and positioned with a 2.5 mm-long interelectrode gap. The cavity was composed of a stainless-steel base, a sealed quartz-glass body, a charging/extracting valve and an electrode spacing adjustment screw. The unit can withstand a maximum pressure of 0.7 MPa.
The gas chamber was evacuated to vacuum and then filled with inert buffer gas after stabilization for 20 min. The procedure above was repeated three times to eliminate the effects of other impurities in the test chamber. The experimental gas was injected into the chamber until the pressure was equal to the saturated vapor pressure (absolute pressure at 20 °C). An increasing voltage method was applied to the discharge chamber through a power frequency voltage to determine the instantaneous breakdown voltage. The tests were conducted at least 5 times with a gap of 4 min between every two breakdowns.
The breakdown voltages of NSF3 and SF6 under the same conditions are shown in Table 2, which shows that the insulation strength of NSF3 (whose purity was approximately 87%) was 1.28 times that of SF6.
Table 2 The dielectric strength of NSF3 and SF6
Gas |
Pressurea/MPa |
Purityb/% |
Breakdown voltage/kV |
Isolated pressure. The impurities of NSF3 were SF6, CCl3F, and SO2, as determined by GC-MS. |
SF6 |
0.3 |
100 |
35.23 |
NSF3 |
0.3 |
87 |
45.08 |
Results and discussion
Effects of the molar ratio of reactants on the yield of tetrasulfur tetranitride
According to previous studies, the yield was greatest when ammonia was passed through at a rate of 900–1000 mL min−1.22 However, the effect of ammonia on the reaction was related to not only its injection speed but also its total amount by affecting the concentration of the reactants. Therefore, the reaction of ammonia to sulfur monochloride in a molar ratio from 6
:
1 to 17
:
1 was studied, and the synthetic route and results are displayed in Table 3. The yield of 2 increased from 30.3% to 73.8% as the molar ratio increased and then remained constant when the amount of ammonia reached over 16 equivalents of sulfur monochloride. Thus, the optimal molar ratio of sulfur monochloride to ammonia should be 1
:
16.
Table 3 Effects of the various molar ratios of sulfur monochloride to ammonia on the yield of 2a
Effects of recrystallization conditions on the yield of tetrasulfur tetranitride
Sulfur was found to be the main impurity after removal of ammonium chloride by distilled water. Recrystallization was one of the most important steps in the preparation of S4N4. In addition to temperature, diverse types of solvent could also directly affect the process of crystallization since the solubility varies by material. Nevertheless, the solubilities of S4N4 and sulfur were extremely similar; thus, choosing an appropriate solvent became key to improving the yield. Accordingly, benzene, 1,4-dioxane, and cyclohexane were selected as alternative solvents for recrystallization after taking polarity and boiling point into account; therefore, the dissolution differences in various temperature phases were tested. As shown in Fig. 4, the solubilities of S4N4 and sulfur in cyclohexane were relatively low, and the temperature sensitivities showed similar trends, which indicated that cyclohexane cannot be used as a solvent during the recrystallization process. By contrast, the solubilities of sulfur and S4N4 in 1,4-dioxane were higher than those of the other compounds. However, the solubility of sulfur was too sensitive to temperature, which resulted in the formation of a turbid liquid and eutectoid. Both S4N4 and sulfur can be well dissolved by benzene, which has a good thermosensitivity to S4N4. In general, benzene was treated as the optimal solvent for recrystallization.
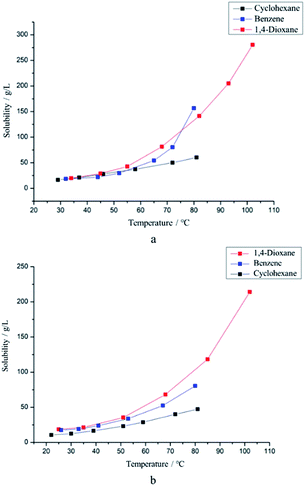 |
| Fig. 4 (a) The solubility of S4N4 varies with temperature in three solvents. (b) The solubility of sulfur varies with temperature in three solvents. | |
Furthermore, the cooling temperature of crystallization was also a decisive factor; thus, reactions were carried out over a temperature gradient to verify the optimal temperature. Table 4 provides the relationship between recrystallization yield and cooling temperature. The results showed that the solution cooled rapidly, and the two substances were simultaneously precipitated by supersaturation at a low temperature (lower than 10 °C, entries 1–2). The yield of S4N4 increased obviously from 27.1% to 52.2% with the increase in temperature from 15 °C to 30 °C (entries 3–6). S4N4 precipitated first and separated from sulfur when the temperature ranged from 10–20 °C. However, sulfur crystallized after some time, resulting in a low yield. As shown in Table 4, the optimum cooling temperature was 30 °C, and several orange needle-shaped crystals of good crystal shape were obtained at this time, while the yield was up to 52.2%. At this point, the cooling rate was moderate, which contributed to the formation of the S4N4 crystal nucleus, thus making it easier to avoid the precipitation of sulfur. Further heating accelerated the dissolution of S4N4, leading to a decrease in yield (entry 7).
Table 4 Effect of cooling temperature on recrystallization yielda
Entry |
Temperature/°C |
Yield (%) |
Reaction conditions: impurity-containing S4N4 was dissolved by stirring with benzene, and the saturated solution was heated to 80 °C before cooling. No expected product was obtained. |
1 |
5 |
—b |
2 |
10 |
—b |
3 |
15 |
27.1 |
4 |
20 |
38.4 |
5 |
25 |
50.4 |
6 |
30 |
52.2 |
7 |
35 |
48.1 |
Such efforts were made to promote the industrialization of the route. For instance, unpurified S4N4 was reacted with AgF2, which resulted in a substantial amount of NSF3 and effectively avoided environmental pollution by carcinogenic benzene. However, the purification process of gases remains to be further explored.
Effects of fluidizer on the yield of thiazyl trifluoride
This reaction was a combination of a S–N compound with a double-wedge cage structure and an active fluoride ion provided by silver difluoride. The reaction procedure is shown in Fig. 5. Apparently, the reactivity and mass of the active fluoride ion played key roles in the yield of NSF3. We had already tried different kinds of fluorinating agents, such as KF (with 18-crown-6 as the phase transfer catalyst), CsF and AgF2. The target product NSF3 could only be obtained by AgF2. It was worth noting that AgF2 was highly susceptible to deterioration during the loading process, which would apparently result in the loss of a considerable amount of raw material, resulting in a low yield. Therefore, we investigated the effects of AgF2 on the yield. As shown in Table 5, the yield of NSF3 was found to increase from 21.32% to 57.38% (entries 1–5) when the AgF2
:
S4N4 molar ratio increased from 8
:
1 to 16
:
1, owing to the increase in the concentration of F−. After that, the yield of NSF3 approached a steady state at approximately 58% upon further addition of AgF2 (entry 6). Based on our findings, it could be concluded that the optimal molar ratio of AgF2 to S4N4 was 16
:
1, considering economic factors and reaction efficiency.
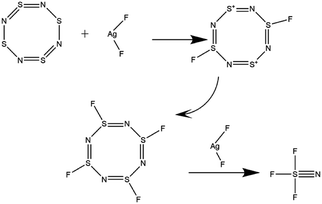 |
| Fig. 5 Pathway for the synthesis of thiazyl trifluoride from tetrasulfur tetranitride. | |
Table 5 Effects of the AgF2
:
S4N4 molar ratio on the yield of NSF3a
Entry |
Molar rate (AgF2/S4N4) |
Reaction time/h |
Temperature/°C |
Yieldb/(%) |
Reaction conditions: S4N4 (3 mmol), CCl4 (waterless, 30 mL), and AgF2 were added in the specified proportions under stirring. Isolated yield. |
1 |
8 : 1 |
2 |
78 |
21.32 |
2 |
10 : 1 |
2 |
78 |
32.32 |
3 |
12 : 1 |
2 |
78 |
44.79 |
4 |
14 : 1 |
2 |
78 |
53.44 |
5 |
16 : 1 |
2 |
78 |
57.38 |
6 |
18 : 1 |
2 |
78 |
58.27 |
Effects of reaction conditions on the preparation of thiazyl trifluoride
NSF3 was synthesized from S4N4 and AgF2 by ring-opening fluorination; the three S–F single bonds were formed in a stepwise manner, which meant insufficient reaction time would result in a low crude yield of the product. Consequently, it was necessary to keep the reaction stable at a constant temperature for a period of time. William H. Kirchhoff reported that the whole process was approximately 3 hours. However, it was found that the yield increased from 28.12% to 57.15% as the reaction time was extended to 2 hours (as shown in Table 6). Further extension of the reaction time had no significant effect on yield; thus, the time should be controlled for approximately 2 hours to obtain the highest yield and maximum economic benefit.
Table 6 Effects of reaction time on the yield of NSF3a
Entry |
Timeb/h |
Yieldc/(%) |
Reaction conditions: S4N4 (3 mmol), AgF2 (48 mmol), and CCl4 (waterless, 30 mL). The reaction was carried out under stirring at 78 °C. Timing began after AgF2 was added. Isolated yield, measured by GC-MS. |
1 |
0.5 |
28.12 |
2 |
1 |
35.35 |
3 |
1.5 |
43.03 |
4 |
2 |
57.15 |
5 |
2.5 |
57.54 |
6 |
3 |
57.68 |
To purify the target gas, GC-MS was used to identify the impurities in the crude product, and SF6, CCl3F and SO2 were confirmed to be the main impurities. The multistage condensation method was used for further purification, in conjunction with a potassium permanganate solution and anhydrous calcium chloride. In consideration of the boiling point of NSF3 and other impurity gases, ice-salt baths with different temperature gradients for condensation were set up, and the impacts on the purity are displayed below. By comparing the results shown in Table 7, it was found that the purity increased first and then decreased as the temperature decreased. Moreover, the purity of NSF3 reached 90% when the condensing temperature was −15 °C. It was speculated that this result was because impurities whose boiling points were higher than that of NSF3 were easier to liquefy and separate during the temperature reduction process. However, the target gas was also liquefied, and the gas flow rate decreased as the condensing temperature gradually approached the boiling point of NSF3, owing to the absence of an inert carrier gas, thereby leading to a decrease in the overall purity of the product. Therefore, it was suggested that the first-stage condensation temperature should be −15 °C to maximize the purity of NSF3.
Table 7 Effects of condensing temperature on the purity of NSF3a
Entry |
Condensing temperature/°C |
Purityb/% |
Reaction conditions: S4N4 (3 mmol), AgF2 (48 mmol), and CCl4 (waterless, 30 mL). The reaction was carried out under stirring at 78 °C for 2 hours, and the gas mixture was condensed at different temperatures. GC purity. |
1 |
−5 |
49 |
2 |
−10 |
60 |
3 |
−15 |
90 |
4 |
−20 |
82 |
5 |
−25 |
75 |
Hence, NSF3 was successfully synthesized via a two-step reaction using sulfur monochloride, ammonia and silver(II) fluoride as raw materials. The total yield of NSF3 was 25% under the optimal conditions, and the purity was over 90%.
Assessment of the thermal stability of thiazyl trifluoride
To evaluate the thermal stability of thiazyl trifluoride, normal vibration mode analysis was performed, and the vibration free energy at 298 K was estimated to predict the reaction free energy of the radical decomposition. The simulation of the gas phase radical decomposition reaction was performed with Gaussian 09 software. The geometries of all molecules were optimized by B3LYP/6-31G(d,p). The convergence threshold for geometry optimization was tighter than the default value (opt = tight), and tight grid integration was applied to perform DFT simulation (int = ultrafine).
The schematic illustration of radical decomposition is shown in Fig. 6, and we consider two types of bond cleavage between N–S and S–F to assess all the decomposition patterns.
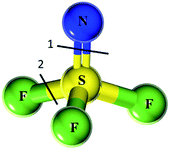 |
| Fig. 6 Schematic illustration of the radical decomposition of a molecule. | |
All the structures of molecules after geometry optimization are listed in Fig. 7. For the first step of radical decomposition, one of the F or N atoms is deleted, forming a single radical molecule, which results in the
and
as shown in Fig. 7B and C.
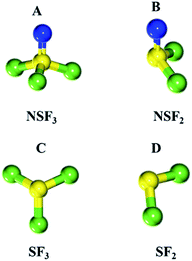 |
| Fig. 7 Geometry of molecules after optimization with B3LYP/631G(d,p). (A) Geometry of NSF3 molecules. (B) Geometry of NSF2 after the first step of decomposition (losing a fluorine atom). (C) Geometry of SF3 after the first step of decomposition (losing a nitrogen atom). (D) Geometry of SF2 resulting from the decomposition of SF3. | |
The reaction free energy for the radical decomposition reaction of the NSF3 molecule is shown in Table S2.† The bond cleavage between F and S produces the lowest reaction energy (58.22 kcal mol−1) and decomposition into molecules B. The other pathway produces molecules C and nitrogen radical, resulting in a reaction energy higher than 58.22 kcal mol−1. Thus, it is difficult to decompose (especially radical nitrogen atom). The decomposition from
to
(as shown in Fig. 7D) and F radical has the lowest reaction energy in this system. All the simulation results listed above suggest the formation of
radical. Furthermore, it has been confirmed that the thermal unimolecular decomposition or isomerization of NSF3 is negligible even at extremely high temperatures (e.g., ∼3000 K whenever partial discharge or arc occurs).16
Comparison with other insulating gases
A comparison of the physical and environmental properties of NSF3 and other mainstream potential substitute gases is shown in Table S3.† Although c-C4F8 has desirable insulating properties, the GWP remains high at 8700. Besides, c-C4F8 discharges at high energy may precipitate carbon, which may affect the insulation capacity. C5F10O and C4F7N have disadvantages of their high boiling point (26.5 °C and −4.7 °C, respectively), so they need to be mixed with buffer gases to avoid the obstacle of high boiling point to a certain extent. The gas CF3I exhibits high dielectric strength, low boiling point and GWP. However, it has been proven that iodine precipitation can be produced with high voltage and severe discharge, which may lead to a decline of dielectric strength. It can be concluded from the comparison that the performance of NSF3 in various aspects is relatively excellent and has certain application prospects.
Conclusions
In this paper, an efficient and convenient synthesis route of thiazyl trifluoride from sulfur monochloride was developed. It is worth noting that this compound has the potential to be a novel eco-friendly insulating gas to replace SF6 in high-voltage transmission systems. Sulfur monochloride reacted with ammonia in an ice bath to obtain tetrasulfur tetranitride. Afterwards, tetrasulfur tetranitride was fluorinated by AgF2 and treated with multistage condensation to produce thiazyl trifluoride. The conditions of the relevant reactions were also optimized to maximize the yield and purity. The overall yield of NSF3 was approximately 25%, and the purity was up to 90.6%, which has not been mentioned in previous research. The dielectric strength of NSF3 was confirmed to be 1.28 times that of SF6 through electrical experiments, indicating that NSF3 might be a potential alternative to SF6.
Conflicts of interest
There are no conflicts to declare.
Acknowledgements
The work was supported by the National Key R&D Program of China (2017YFB0902500) and State Grid Science & Technology Project (The Key Technology of Environmentally-Friendly Gas-Insulated Transmission Lines). In addition, we are thankful for the technical assistance by Wuhan University Testing Center and School of Electrical Engineering.
Notes and references
- M. Maiss and C. A. M. Brenninkmeijer, Atmospheric SF6: trends, sources, and prospects, Environ. Sci. Technol., 1998, 32, 3077–3086 CrossRef CAS.
- Y. Kieffel and F. Biquez, SF6 alternative development for high voltage switchgears, IEEE Electrical Insulation Conf. Seattle, 7–10 June 2015, pp. 379–383 Search PubMed.
- E. A. Ray, F. L. Moore and J. W. Elkins, et al., Quantification of the SF6 lifetime based on mesospheric loss measured in the stratospheric polar vortex, J. Geophys. Res.: Atmos., 2017, 122, 4626–4638 CAS.
- D. Fench, Kyoto Protocol to the United Nations Framework Convention on Climate Change, Rev. Eur. Community Int. Environ. Law, 1998, 7, 214–217 CrossRef.
- M. Rabie and C. M. Franck, Assessment of eco-friendly gases for electrical insulation to replace the most potent industrial greenhouse gas SF6, Environ. Sci. Technol., 2018, 52, 369–380 CrossRef CAS.
- A. Beroual and A. Haddad, Recent advances in the quest for a new insulation gas with a low impact on the environment to replace sulfur hexafluoride (SF6) gas in high-voltage power network applications, Energies, 2017, 10, 1216 CrossRef.
- Y. Deng, B. Li and D. Xiao, Analysis of the insulation characteristics of C3F8 gas mixtures with N2 and CO2 using boltzmann equation method, IEEE Trans. Dielectr. Electr. Insul., 2015, 22, 3253–3259 CAS.
- H. Katagiri, H. Kasuya and H. Mizoguchi, Investigation of the performance of CF3I gas as a possible substitute for SF6, IEEE Trans. Dielectr. Electr. Insul., 2008, 15, 224–299 Search PubMed.
- X. Zhang, Y. Li, S. Xiao, L. Huang, J. Tang, Z. Deng and S. Tian. Study on the discharge characteristics of an environmental-friendly insulating medium C5F10O, Proc. CSEE, 2018, vol. 38, pp. 4298–4306 Search PubMed.
- X. Zhang, Y. Li, S. Tian, S. Xiao, D. Chen, J. Tang and R. Zhuo, Decomposition mechanism of the C5-PFK/CO2 gas mixture as an alternative gas for SF6, Chem. Eng. J., 2018, 336, 38–46 CrossRef CAS.
- Y. Li, X. Zhang, S. Tian, S. Xiao, Y. Li and D. Chen, Insight into the decomposition mechanism of C6F12O-CO2 gas mixture, Chem. Eng. J., 2019, 360, 929–940 CrossRef CAS.
- Z. Gao, M. Wang, S. Wang, Y. Wang, R. Peng, P. Yu and Y. Luo, Novel and efficient synthesis of insulating gas-heptafluoroisobutyronitrile from hexafluoropropylene, R. Soc. Open Sci., 2019, 6, 181751 CrossRef PubMed.
- O. Yamamoto, T. Takuma, S. Hamada and Y. Yamakawa, Applying a gas mixture containing c-C4F8 as an insulation medium, IEEE Trans. Dielectr. Electr. Insul., 2001, 8, 1075–1081 CrossRef CAS.
- X. Liu, J. Wang, Y. Wang, Z. Zhang and D. Xiao, Analysis of the insulation characteristics of c-C4F8/CO2 gas mixtures by the Monte Carlo method, J. Phys. D: Appl. Phys., 2008, 41, 015206 CrossRef.
- Y. Wang, M. Sun and Z. Gao, et al., A novel and efficient synthetic route to perfluoroisobutyronitrile from perfluoroisobutyryl acid, RSC Adv., 2018, 8, 37159 RSC.
- X. Yu, H. Hou and B. Wang, A Priori Theoretical Model for Discovery of Environmentally Sustainable Perfluorinated Compounds, J. Phys. Chem. A, 2018, 122, 3462–3469 CrossRef CAS PubMed.
- W. H. Kirchhoff and E. B. Wilson, The Microwave Spectrum and Structure of NSF3, J. Am. Chem. Soc., 1961, 85, 1726–1729 CrossRef.
- O. Glemser, H. Meyer and A. Haas, Schwefel-Stickstoff-Fluorverbindungen, XIII. Weitere Mitteilung über NSF und NSF3, Eur. J. Inorg. Chem., 1964, 97, 1704–1709 CAS.
- O. Glemser and R. Mews, Chemistry of thiazyl fluoride (NSF) and thiazyl trifluoride (NSF3): a quarter century of sulfur-nitrogen-fluorine chemistry, Angew. Chem., Int. Ed. Engl., 1980, 19, 883–899 CrossRef.
- A. F. Clifford and C. S. Kobayashi, The Preparation and Properties of N-Fluoroformyliminosulfur Difluoride, SF2=NCOF, Inorg. Chem., 1964, 4, 571–574 CrossRef.
- Y. Zheng, W. Zhou, H. Li, X. Yan, Z. Li, W. Chen and K. Bian, Influence of conductor surface roughness on insulation performance of C4F7N/CO2 mixed gas, IEEE Trans. Dielectr. Electr. Insul., 2019, 26, 922–929 CAS.
- H. B. V. Valkenburgh and J. C. Bailar, Nitrogen tetrasulfide and nitrogen tetraselenide, J. Am. Chem. Soc., 1925, 47, 2134–2137 CrossRef.
Footnote |
† Electronic supplementary information (ESI) available. See DOI: 10.1039/c9ra09256k |
|
This journal is © The Royal Society of Chemistry 2020 |