DOI:
10.1039/C9NJ05100G
(Paper)
New J. Chem., 2020,
44, 258-264
Hierarchically porous BiOCl@NiCo2O4 nanoplates as low-cost and highly efficient catalysts for the discoloration of organic contaminants in aqueous media†
Received
10th October 2019
, Accepted 20th November 2019
First published on 20th November 2019
Abstract
The construction of noble metal-free materials with remarkable activity and high stability via a simple, green, efficient, and low-cost method remains a crucial task for addressing the problem of water contamination. Herein, ultrathin NiCo2O4 nanosheets were in situ assembled on the surface of BiOCl single-crystal nanosheets with a vertical orientation to form a well-defined hierarchically porous structure. Notably, the obtained BiOCl@NiCo2O4 nanoplates displayed outstanding catalytic activity in the reductive conversion of 4-nitrophenol (4-NP) to 4-aminophenol (4-AP). On account of their special morphology, plentiful porosity, and good stability, the as-prepared composite could finish the reduction reaction within 280 s with a large rate constant (kapp) of 7.18 × 10−3 s−1. The BiOCl@NiCo2O4 nanoplates possessed excellent recyclability, and the original activity could be maintained even after seven consecutive cycles. Furthermore, the resultant BiOCl@NiCo2O4 could also act as a hyperactive catalyst for the reductive discoloration of organic dyes (rhodamine B, rhodamine 6G, Congo red, and methyl orange). Our strategy, therefore, promotes the development of low-cost and high-performance noble metal-free catalysts to treat water pollution.
Introduction
With the rapid economic growth, the process of urbanization and industrialization is accelerating. This is followed by the excess discharge of industrial effluents and sanitary sewage into freshwater streams, which results in a scarcity of water resources and greatly threatens the security of national water resources.1–3 Wastewater that includes various kinds of organic contaminants such as nitro aromatics and fertilizers, dye wastes, and microbial pathogens can cause a serious risk to the aquatic ecosystem and human health.4–8 In the factory wastewater, the most common and stubborn contaminant is 4-nitrophenol (4-NP), which is quite hazardous to human beings and animals even at low concentrations.9,10 However, the reduction product of 4-NP, namely, 4-aminophenol (4-AP) can be used as a crucial intermediate product in the synthesis of antipyretic, analgesic and clofibrate drugs. In addition, as a photographic developer, antioxidant and anticorrosion lubricant, 4-AP has been extensively applied in various fields.11,12 Hence, the catalytic conversion of 4-NP into 4-AP has garnered vast attention from academia nowadays. Other kinds of stubborn contaminants in wastewater are organic dyes, which are commonly used in cosmetic, textile, food, leather, paper and plastic industries due to their bright colors and easy applications.13–15 The continuous enrichment of organic dyes in water can change the color significantly and hinder the penetration of sunlight into water, thus influencing the normal growth of aquatic organisms. Moreover, a gigantic majority of dyes are acknowledged as potential carcinogens and are harmful to human health.16–19 Therefore, it is urgent to search for efficient and environmentally friendly methods to deal with water contaminated with these organic dyes.
Currently, multifarious techniques have been used for removing organic contaminants, such as adsorption, electrochemical degradation, photocatalytic degradation, oxidation/reduction, coagulation/flocculation or biological degradation.20–22 Among these different techniques, the catalytic reductive discoloration of dyes assisted by NaBH4 has aroused wide public attention due to its high efficiency, quick operating protocol, cost effectivity, and mild reaction conditions.23,24
So far, a wide variety of catalysts with different morphologies based on noble metals (Au, Ag, Pd and Pt) have been constructed for the reduction and discoloration of 4-NP and organic dyes.25–27 Despite the considerable catalytic activity of these noble metal catalysts for the degradation reaction, they are not without their problems, such as high cost, low abundance, and complicated multi-step synthesis. All of these deficiencies extremely limit the wide range of applications of precious metal catalysts in practice. Thus, the exploration of inexpensive and stable earth-abundant metal-based catalysts with outstanding catalytic activity is urgently desired for both fundamental research and practical applications.
In recent years, mixed transition-metal oxides have attracted considerable attention because of their low cost, tunable valence states, easy fabrication, abundant reactive sites, and environmental friendliness.28–31 As one of the typical mixed transition-metal oxides, NiCo2O4 possesses outstanding electrocatalytic activity and can be applied in the fields of supercapacitors,32,33 optoelectronic devices,34 electrocatalysts35,36 and lithium-ion batteries.37,38 Bismuth oxychloride (BiOCl), a star material in the field of photocatalysis, has attracted worldwide attention due to its striking photocatalytic activity, corrosion resistance, and unique layered structure.39–41 Nowadays, BiOCl has been widely applied in various areas, such as photocatalysis, energy storage, and pollutant decomposition.42–44 Unfortunately, till now, the catalytic reduction performance of these materials has rarely been reported. Hence, it is necessary to further explore the catalytic reduction functions of NiCo2O4 and BiOCl.
Based on these above-mentioned considerations, in this paper, we disclosed a facile and efficient approach for the construction of hierarchically porous BiOCl@NiCo2O4 nanoplates. By using the hydrothermal method, BiOCl single-crystal nanosheets could be obtained. Then, the as-prepared BiOCl nanosheets were used as the precursor to reflux with Ni(NO3)2·6H2O and Co(NO3)2·6H2O to fabricate intermediate products. Finally, the BiOCl@NiCo2O4 nanoplates were prepared by calcinating the intermediate products at 350 °C in air for 120 min. The obtained BiOCl@NiCo2O4 displayed a coarse surface structure, and the surface of the BiOCl nanosheets was uniformly coated with a large amount of vertically oriented NiCo2O4 nanosheets to form a well-defined hierarchically porous structure. Surprisingly, this nanocomposite exhibited outstanding catalytic activity and remarkable reusability for the reduction of 4-NP and the discoloration of commercial organic dyes without using noble metals. Moreover, BiOCl@NiCo2O4 could be further applied for the discoloration of the organic contaminants in the Yellow River water.
Results and discussion
Preparation and characterization of BiOCl@NiCo2O4 nanoplates
The BiOCl@NiCo2O4 nanoplates were fabricated by a convenient method involving three steps, namely, hydrothermal, reflux and thermal treatments. As depicted in Scheme 1, using Bi(NO3)3·5H2O and KCl as the precursors, BiOCl single-crystal nanosheets can be synthesized by the hydrothermal method according to a previously reported method.45 Then, BiOCl@NiCo2O4 was obtained via refluxing the as-prepared BiOCl nanosheets with a certain amount of Ni(NO3)2·6H2O and Co(NO3)2·6H2O, followed by calcination at 350 °C in air for 120 min.
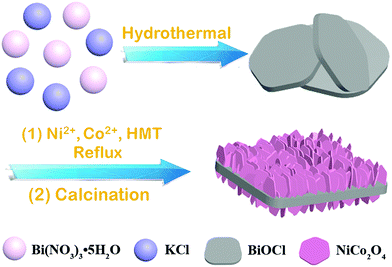 |
| Scheme 1 Schematic illustration for the preparation of BiOCl@NiCo2O4 nanoplates. | |
The surface morphology and structure of the as-obtained BiOCl, NiCo2O4 nanosheets and BiOCl@NiCo2O4 were investigated via scanning electron microscopy (SEM). As shown in Fig. 1A, pure BiOCl comprises large-scale sheet-like nanostructures, and the smooth surface texture can also be observed in the amplified image (Fig. 1B). Moreover, the morphology of BiOCl barely changed after calcination at 350 °C in air for 120 min (Fig. S1, ESI†). In comparison to the glossy surface of pure BiOCl, after the NiCo2O4 growing process, the surface of the BiOCl nanosheets was uniformly coated with a large amount of vertically oriented NiCo2O4 nanosheets, which displayed a coarse surface structure (Fig. 1C). In addition, BiOCl@NiCo2O4 in the high-resolution FE-SEM images further illustrated that these vertically oriented nanosheets could be interconnected with each other to form a well-defined hierarchically porous structure on the BiOCl substrate (Fig. 1D). For comparison, the morphology of the pristine NiCo2O4 nanosheets was also measured. As shown in Fig. S2 (ESI†), pristine NiCo2O4 is composed of hexagonal nanosheets with large-scale aggregation. The above-mentioned phenomenon revealed that the BiOCl nanosheets played a substantial role in the formation of vertically oriented NiCo2O4 nanosheets. Moreover, the successful synthesis of BiOCl@NiCo2O4 was further confirmed by energy dispersive X-ray (EDX) analysis, which indicated the existence of Bi, O, Cl, Ni, and Co in the catalysts (Fig. S3, ESI†). The homogeneous dispersion of Bi, O, Cl, Ni, and Co in BiOCl@NiCo2O4 was further verified by the corresponding elemental mapping (Fig. 1a–f).
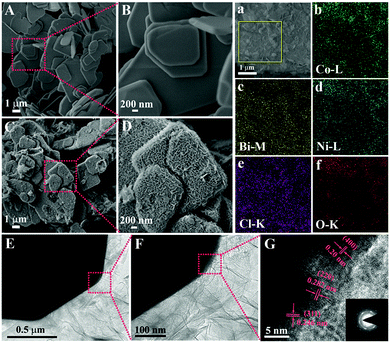 |
| Fig. 1 Different magnification SEM images of BiOCl nanosheets (A and B) and BiOCl@NiCo2O4 nanoplates (C and D). (a) SEM images of the selected area. (b–f) Element mapping images of BiOCl@NiCo2O4 nanoplates. (E and F) TEM images of BiOCl@NiCo2O4. (G) HRTEM image of the BiOCl@NiCo2O4. Inset: Selected area electron diffraction of BiOCl@NiCo2O4. | |
In order to comprehend more details of the microstructure of BiOCl@NiCo2O4, transmission electron microscopy (TEM) and high-resolution TEM (HRTEM) were carried out. As presented in Fig. 1E, numerous vertically oriented NiCo2O4 nanosheets (lighter color area) are evenly assembled on the surface of the BiOCl nanosheets (black color area). The high-resolution TEM (HRTEM) image marked in the noted area, as presented in Fig. 1G, reveals visible lattice fringes, which can be well indexed to the (311), (400) and (220) planes of spinel NiCo2O4.46–48 The above results were also in good agreement with the XRD pattern (Fig. 2A).
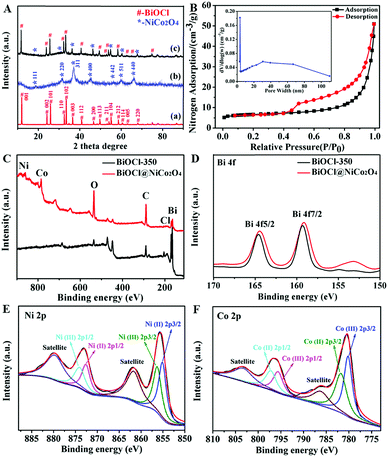 |
| Fig. 2 (A) XRD pattern of BiOCl-350 (a), NiCo2O4 nanosheets (b) and BiOCl@NiCo2O4 (c). (B) Nitrogen adsorption/desorption isotherms of BiOCl@NiCo2O4. Inset: Pore size distribution of BiOCl@NiCo2O4. XPS survey spectrum (C) and high-resolution scans for Bi 4f electron (D) of BiOCl@NiCo2O4 and BiOCl-350. High-resolution scans for the Ni 2p electron (E) and Co 2p electron (F) of BiOCl@NiCo2O4. | |
To better understand the structural properties of different samples, XRD analysis was conducted. The corresponding XRD result displayed in Fig. 2A clearly reveals that BiOCl@NiCo2O4 exhibits two groups of diffraction peaks, which are attributed to the tetragonal structure of BiOCl (JCPDS no. 06-0249)49,50 and the spinel NiCo2O4 phase (JCPDS Card no. 20-0781).51–53 Moreover, no other characteristic peaks for impurities, such as Co3O4 and NiO, could be detected, indicating that NiCo2O4 assembled on the surface of the BiOCl nanosheets was pure.
Moreover, nitrogen adsorption–desorption isotherms and pore size distribution curves were measured to explore the meso- and macroporous features of BiOCl@NiCo2O4. As shown in Fig. 2B, the isotherms of BiOCl@NiCo2O4 exhibit curves similar to the type IV sorption model. The surface area of BiOCl@NiCo2O4 was certified to be 20.88 m2 g−1, which was 17 times greater than that of the NiCo2O4 nanosheets (1.25 m2 g−1). The BJH analysis of the desorption branch of the isotherm, as displayed in the inset of Fig. 2B, indicates that there is a large portion of pores in the range of 3–4 nm. These results verified the existence of mesopores in BiOCl@NiCo2O4.
For further insights into the oxidation states and chemical composition of the as-prepared BiOCl@NiCo2O4, X-ray photoelectron spectroscopy (XPS) was performed. From the typical XPS survey spectrum in Fig. 2C, it can be seen that only Bi, O and Cl exist in BiOCl-350. However, the XPS survey spectrum of BiOCl@NiCo2O4 revealed the existence of Bi, O, Cl, Ni and Co in BiOCl@NiCo2O4. The high-resolution spectrum of Bi 4f is shown in Fig. 2D. Compared to the observation for BiOCl-350, the Bi 4f peaks for BiOCl@NiCo2O4 shift from 159.9 eV (Bi 4f7/2) and 165.1 eV (Bi 4f5/2) to 159.6 eV (Bi 4f7/2) and 164.8 eV (Bi 4f5/2), respectively. The downshift in the Bi 4f peaks indicates the successful synthesis of the BiOCl@NiCo2O4 composite. The O 1s spectrum was divided into three symmetric peaks (Fig. S5, ESI†). The peaks at the binding energies of 530.4 eV, 531.6 eV and 532.6 eV were attributed to oxygen in M–O–M (M = Ni/Co/Bi) in the spinel of BiOCl@NiCo2O4, defect sites, and adsorbed water, respectively.54,55 The high-resolution Ni 2p spectrum depicted in Fig. 2E exhibits two spin–orbit doublets of Ni2+ and Ni3+ and two shake-up satellites. The peaks at the binding energies of 855.1 eV and 872.6 eV were indexed to Ni2+ 2p3/2 and Ni2+ 2p1/2, whereas the peaks at the binding energies of 856.3 eV and 874.2 eV belonged to Ni3+ 2p3/2 and Ni3+ 2p1/2, respectively. Furthermore, two types of chemical valence states of the Co species can also be obtained in the Co 2p spectrum (Fig. 2F). The peaks at the binding energies of 781.8 eV and 797.3 eV correspond to Co2+ 2p3/2 and Co2+ 2p1/2, while the peaks at the binding energies of 781.9 eV and 797.2. eV are assigned to Co3+ 2p3/2 and Co3+ 2p1/2, respectively.56–58 The above-mentioned results revealed that the NiCo2O4 nanosheets had successfully assembled on the surface of the BiOCl nanosheets.
Catalytic performance of samples in the reduction of 4-NP and reductive degradation of organic dyes
As typical examples of hazardous and persistent organic contaminants in industrial water, 4-nitrophenol (4-NP) and organic dyes such as rhodamine B (RhB), rhodamine 6G (R6G), Congo red (CR) and methyl orange (MO) are harmful to human beings and have a negative impact on aquatic organisms. Hence, the reduction of 4-nitrophenol and the reductive discoloration of the above-mentioned organic dyes were chosen as the model reactions to evaluate the catalytic activity of BiOCl@NiCo2O4 under normal temperature and pressure conditions. The reduction reactions were conducted in the presence of NaBH4 and the reaction processes were monitored by UV-vis spectroscopy. As displayed in Fig. S7 (ESI†), the 4-NP solution immediately changes from light yellow to bright yellow after the addition of a freshly prepared NaBH4 solution, and the maximum absorption wavelength shifts from 319 nm to 400 nm. This can be attributed to the formation of the 4-nitrophenolate ions under alkaline conditions upon the addition of a freshly prepared NaBH4 solution. Without the presence of the catalyst, the color of the solution was basically invariable after a long time, which indicated that the reduction reaction was a failure (Fig. S8, ESI†). Nevertheless, after the addition of a small amount of BiOCl@NiCo2O4 to the reaction mixture, the bright yellow solution immediately transformed to transparent within 280 s (Fig. 3A inset), which indicated the completion of the reduction reaction. Meanwhile, the characteristic absorption peak of 4-NP around 400 nm quickly decreased, and a new peak located at 300 nm related to 4-AP began to appear (Fig. 3A). Furthermore, the catalytic performances of BiOCl-350 (Fig. 3B) and pure NiCo2O4 (Fig. 3C) were also evaluated in the same conditions by the reduction reaction of 4-NP. The results proved that the order of the catalytic performance for the prepared products is BiOCl@NiCo2O4 > BiOCl-350 > NiCo2O4. These results suggest that there are synergistic effects between the BiOCl nanosheets and the NiCo2O4 nanosheets in BiOCl@NiCo2O4 during the catalytic process. It is noteworthy that the amount of NaBH4 was in excess compared to 4-NP; thus, the reduction reaction should follow the pseudo first-order kinetic equation (ln(Ct/C0) = −kappt). As depicted in Fig. 3D, in order to give a better comparison, the catalytic activities of the BiOCl@NiCo2O4, BiOCl-350, and NiCo2O4 nanosheets were also measured. The apparent rate constants, kapp, of the prepared BiOCl@NiCo2O4, BiOCl-350 and NiCo2O4 catalysts were found to be 7.18 × 10−3 s−1, 3 × 10−3 s−1 and 0.928 × 10−3 s−1, respectively, according to the slope of the fitted line (Fig. 3D and Table S1, ESI†). The catalytic efficiency of the BiOCl@NiCo2O4 nanocatalyst was obviously superior to that of the reported noble metal catalysts (Table S2, ESI†).
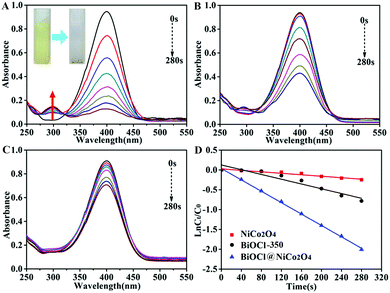 |
| Fig. 3 Time-dependent UV-visible spectrum for the reduction of 4-NP to 4-AP in water after adding BiOCl@NiCo2O4 (A), BiOCl-350 (B), NiCo2O4 nanosheets (C). (D) Plots of ln(Ct/C0) of 4-NP against time using BiOCl@NiCo2O4, BiOCl-350 and NiCo2O4 nanosheets. Inset: Color change of the reaction solution. | |
Meanwhile, the reductive discoloration reactions of different organic dyes (RhB, R6G, CR and MO) were evaluated in the presence of NaBH4 and the processes were recorded by UV-vis absorption spectroscopy. The BiOCl@NiCo2O4 catalyst displayed excellent efficiency when compared with BiOCl-350 and raw NiCo2O4 (Fig. 4 and Fig. S9, S10, ESI†). The apparent rate constants (kapp) of the prepared samples are shown in Fig. S11 and Table S1 (ESI†). The results revealed that BiOCl@NiCo2O4 possessed the highest catalytic activity, which was favourable for the reduction of 4-NP to 4-AP (Fig. S11 and Table S1, ESI†). The catalytic properties of the BiOCl@NiCo2O4 nanocatalyst are evidently more outstanding than those reported for other precious metal catalysts (Table S3, ESI†).
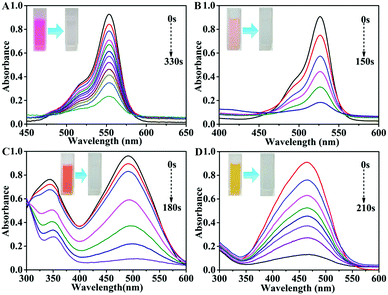 |
| Fig. 4 Time-dependent UV-visible spectra and photographs for the reduction of different organic dyes after adding BiOCl@NiCo2O4. (A) RhB, (B) R6G, (C) CR, (D) MO. | |
Stability and reusability of the BiOCl@NiCo2O4 catalyst
For green catalysis, catalysts with outstanding stability and reusability are surely the most ideal candidates. Therefore, the recyclability of the as-prepared BiOCl@NiCo2O4 was also evaluated by the reduction reaction of 4-NP. After the reaction finished, BiOCl@NiCo2O4 could be easily collected by centrifugation and reused for the next round of cycles. As exhibited in Fig. S12 (ESI†), the reused BiOCl@NiCo2O4 presents excellent activity even for seven consecutive cycles, with nearly one hundred percent conversion within a time period of 332 s. Moreover, the SEM results of the recycled catalyst show no obvious change in the morphology (Fig. S13, ESI†). All of these results indicate that BiOCl@NiCo2O4 possesses excellent stability and reusability.
Catalytic mechanism
A plausible mechanism of the reduction reaction was elucidated (Fig. 5). Actually, the remarkable catalytic activity of BiOCl@NiCo2O4 for the reduction of 4-nitrophenol and organic dyes was due to the following reasons. First, BiOCl@NiCo2O4 possessed a large specific surface area and a structure with abundant pores, which provided many active sites for the adsorption of organic dyes and 4-NP. Furthermore, the BiOCl nanosheets could act as a support for NiCo2O4, which effectively prevented the aggregation of the NiCo2O4 nanosheets and provided accessible surface-active sites for an improved catalytic rate. Moreover, the excellent electron-transport properties of the NiCo2O4 nanosheets could accelerate the electron transfer and further boost the catalytic efficiency. Furthermore, NiCo2O4 supported on the surfaces of BiOCl generally revealed strong support-determined features of size and morphology, which are the essential factors that give rise to enhanced catalytic activity.27,59 Therefore, during the catalytic process, BiOCl@NiCo2O4 served as an electronic intermediate system by transferring electrons from the donor BH4− anions to dye molecules. As shown in Fig. 5, in the initial stage, BH4− adsorbed on the surface of the catalyst can generate reactive nascent hydrogen, which can reduce the H2O molecules to produce H2 that finally acts as a reducing agent for the dye molecules. During this process, the BiOCl@NiCo2O4 nanocomposite accepts electrons from the BH4− ions and transports them to the dye molecules adsorbed on the surface of the nanocomposite. Meanwhile, the generated hydrogen can contribute to the convection of the catalyst and water and simultaneously remove the reaction products to reduce their local concentrations, hence keeping the surface clean for the succeeding catalytic cycles and retaining excellent activity of the catalyst.23,60
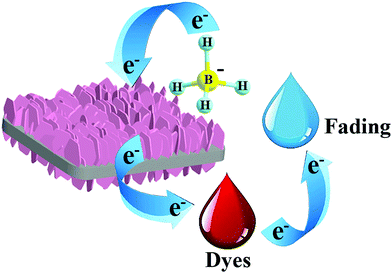 |
| Fig. 5 Schematic mechanism of the reductive discoloration of organic dyes over the BiOCl@NiCo2O4 nanoplates. | |
Environmental application of BiOCl@NiCo2O4
In order to evaluate the practical applications of the as-prepared BiOCl@NiCo2O4 in the treatment of river water contaminated with dyes, the Yellow River water, which was gathered in the Lanzhou section, served as a practical sample. First, the collected Yellow River water was filtered to obtain a clear solution. Then, a series of solutions containing different organic pollutants (4-NP, MO, CR, RhB and R6G) were added to the above water to obtain dye-polluted Yellow River water samples. As depicted in Fig. 6, the polluted Yellow River water is colorful, while the introduction of a certain amount of NaBH4 and BiOCl@NiCo2O4 nanocatalysts to the polluted water can make the mixture became colorless quickly, which reveals that the reductive discoloration of organic pollutants is complete. Hence, the as-prepared BiOCl@NiCo2O4 nanocatalyst can serve as a promising candidate to address the environmental waste water purification.
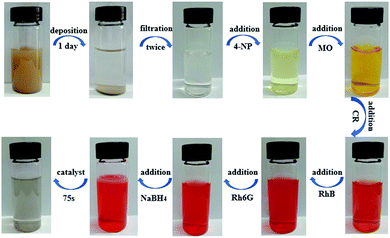 |
| Fig. 6 Schematic diagram of the reductive degradation of 4-NP and four different dyes in the Yellow River water in the presence of BiOCl@NiCo2O4. Reaction conditions: H2O (15 mL), 750 μL 4-NP (1 mM), 750 μL MO (1 mM), 750 μL CR (0.7 mM), 750 μL RhB (0.02 mM), 750 μL R6G (0.16 mM), NaBH4 (536 μL, 2 M) and 2.5 mL BiOCl@NiCo2O4 (1 mg mL−1). | |
Experimental
Chemicals
Ni(NO3)2·6H2O, Co(NO3)2·6H2O, hexamethylenetetramine (HMT), Bi(NO3)3·5H2O, KCl, 4-nitrophenol, sodium borohydride (NaBH4), 4-nitrophenol, rhodamine 6G (R6G), Congo red (CR), methyl orange (MO), and rhodamine B (RhB) were commercially obtained without further purification and treatment. Distilled water was used in all experiments.
Instrumentation
A Sirion 200 (FEI) field-emission scanning electron microscope and Tecnai-G2-F30 (FEI) transmission electron microscope at an acceleration voltage of 300 kV were used to investigate the structure and morphology of the materials. X-ray powder diffraction (XRD) patterns were obtained using a Bruker AXS D8-Advance diffractometer with Cu Kα radiation (λ = 1.5418 Å). X-ray photoelectron spectroscopy (XPS) was performed on a PHI-5702 multifunctional spectrometer. The specific surface areas (SBET) were calculated by using the Brunauer–Emmett–Teller (BET) method with a Micromeritics TriStar II 3020 analyzer. UV-visible absorption spectra (UV-vis) were measured on a UV 1750 spectrometer.
Preparation of BiOCl@NiCo2O4 nanoplates
BiOCl single-crystal nanosheets were prepared according to the reported method.45 Then, the synthesized BiOCl (50 mg) was dispersed into distilled water (50 mL) to form a dispersion by ultrasonication for 60 min. Ni(NO3)2·6H2O (0.05 mmol) and Co(NO3)2·6H2O (0.10 mmol) were dissolved in 13.4 mL deionized water to form solution 1. The dispersion of 65.4 mg HMT in 13.4 mL deionized water was solution 2. Subsequently, the formed solution 1 and solution 2 were gradually added into the BiOCl suspension and refluxed at 120 °C for 5 h. After the reaction was completed, the powder was collected by centrifugation and washed several times by ethanol and deionized water and dried at 60 °C. Finally, the BiOCl@NiCo2O4 nanoplates were obtained by calcinating at 350 °C in air for 120 min with a ramp rate of 5 °C min−1.
Synthesis of NiCo2O4 nanosheets and BiOCl-350
The pristine NiCo2O4 nanosheets were prepared under the same conditions stated above in the absence of BiOCl. BiOCl-350 was prepared by calcining the BiOCl single-crystal nanosheets at 350 °C in air for 120 min with a ramp rate of 5 °C min−1.
Catalysis evaluation
The catalytic reaction of 4-NP to 4-AP was conducted inside a standard quartz cell with a path length of 1 cm at room temperature. Typically, 150 μL of 4-NP (1 mM) and 375 μL of freshly prepared NaBH4 (60 mM) solution were mixed in a quartz cuvette with 2.7 mL deionized water. Afterward, the catalysts (2 mg) were added to the above solution. The catalytic reaction was monitored by using the UV-vis absorption spectra collected at regular time intervals. Moreover, the reductive degradation reactions of different organic dyes [CR (7.0 × 10−4 M), RhB (2.0 × 10−5 M), R6G (1.6 × 10−4 M), MO (6.7 × 10−4 M)] were investigated using a similar method. The following procedure was applied: 150 μL of aqueous solution of the dye [CR (7.0 × 10−4 M), RhB (2.0 × 10−5 M), R6G (1.6 × 10−4 M), MO (6.7 × 10−4 M)], 480 μL NaBH4 (0.1 M) and 2.7 mL deionized water were mixed together into a standard quartz cell. In addition, a fresh catalyst solution (1 mg mL−1, 50 μL) was added to the above-mentioned solution, and the catalytic process was recorded using a UV-vis absorption spectrometer. For comparison, the as-prepared NiCo2O4 and BiOCl-350 were also tested in the above reactions under similar conditions.
Conclusions
In short, a simple, low-cost, facile and efficient strategy was developed to in situ assemble NiCo2O4 nanosheets on the surface of BiOCl single-crystal nanosheets with a well-defined hierarchically porous structure. The resulting BiOCl@NiCo2O4 nanocomposite displayed excellent catalytic activity in the reduction of 4-NP to 4-AP and the discoloration of various organic dyes in the presence of NaBH4. Moreover, the nanocatalyst exhibited remarkable stability and could be recovered and reused for several consecutive cycles. Hence, the as-prepared BiOCl@NiCo2O4 has a great potential to be a hyperactive catalyst for applications in the fields of environmental and green chemistry.
Conflicts of interest
There are no conflicts to declare.
Acknowledgements
This work was supported by grants from the National Natural Science Foundation of China (No. 21864023 and 21761031), the Gansu Provincial Natural Science Foundation of China (No. 18JR3RA087) and the Young Teacher Research Foundation of Northwest Normal University (NWNU-LKQN-16-9).
Notes and references
- E. Cserhati, E. Frogacs and G. Oros, Environ. Int., 2004, 30, 953–971 CrossRef PubMed.
- M. C. M. van Loosdrecht and D. Brdjanovic, Science, 2014, 344, 1452–1453 CrossRef CAS PubMed.
- N. Wang, W. J. Ma, Y. C. Du, Z. Q. Ren, B. H. Han, L. J. Zhang, B. J. Sun, P. Xu and X. J. Han, ACS Appl. Mater. Interfaces, 2018, 11, 1174–1184 CrossRef PubMed.
- T. Cui, Y. H. Zhang, W. Q. Han, J. S. Li, X. Y. Sun, J. Y. Shen and L. J. Wang, Chem. Eng. J., 2017, 315, 335–344 CrossRef CAS.
- S. X. Yi, Y. S. Zou, S. Sun, F. Y. Dai, Y. Si and G. Sun, ACS Sustainable Chem. Eng., 2019, 7, 986–993 CrossRef CAS.
- M. Mon, R. Bruno, J. Ferrando-Soria, D. Armentano and E. Pardo, J. Mater. Chem. A, 2018, 6, 4912–4947 RSC.
- M. Ahmad, M. Yousaf, A. Nasir, I. A. Bhatti, A. Mahmood, X. C. Fang, X. Jian, K. Kalantar-Zadeh and N. Mahmood, Environ. Sci. Technol., 2019, 53, 2161–2170 CrossRef CAS PubMed.
- J. S. Cai, J. Y. Huang, S. Wang, J. Iocozzia, Z. T. Sun, J. Y. Sun, Y. K. Yang, Y. K. Lai and Z. Q. Lin, Adv. Mater., 2019, 31, 1806314 CrossRef PubMed.
- G. Q. Wu, X. Y. Liang, L. J. Zhang, Z. Y. Tang Al-Mamun, H. J. Zhao and X. T. Su, ACS Appl. Mater. Interfaces, 2017, 9, 18207–18214 CrossRef CAS PubMed.
- N. Bingwa, R. Patala, J. H. Noh, J. H. Ndolomingo, M. J. S. Tetyana, S. Bewana and R. Meijboom, Langmuir, 2017, 33, 7086–7095 CrossRef CAS PubMed.
- J. J. Lv, A. J. Wang, X. H. Ma, R. Y. Xiang, J. R. Chen and J. J. Feng, J. Mater. Chem. A, 2015, 3, 290–296 RSC.
- L. H. Zhi, H. Liu, Y. Y. Xu, D. C. Hu, X. Q. Yao and J. C. Liu, Dalton Trans., 2018, 33, 15458–15464 RSC.
- Y. Zhang, P. L. Zhu, L. Chen, G. Li, F. G. Zhou, D. Q. Lu, R. Sun, H. Zhou and C. P. Wong, J. Mater. Chem. A, 2014, 2, 11966–11973 RSC.
- B. F. Sun, H. L. Li, X. Y. Li, X. W. Liu, C. H. Zhang, H. Y. Xu and X. S. Zhao, Ind. Eng. Chem. Res., 2018, 57, 14011–14021 CrossRef CAS.
- Z. X. Liu, L. J. Zhang, F. H. Dong, J. Dang, K. Wang, D. Wu, J. Zhang and J. Fang, ACS Appl. Nano. Mater., 2018, 1, 4170–4178 CrossRef CAS.
- G. Salehi, R. Abazari and A. R. Mahjoub, Inorg. Chem., 2018, 57, 8681–8691 CrossRef CAS PubMed.
- M. A. Rauf and S. S. Ashraf, Chem. Eng. J., 2012, 209, 520–530 CrossRef CAS.
- G. Z. Kyzas, N. K. Lazaridis and M. Kostoglou, Chem. Eng. J., 2014, 248, 327–336 CrossRef CAS.
- S. Kundu, I. H. Chowdhury and M. K. Naskar, J. Chem. Eng. Data, 2018, 63, 559–573 CrossRef CAS.
- Y. X. Zhang, Y. J. Ye, X. B. Zhou, Z. L. Liu, G. P. Zhu, D. C. Li and X. H. Li, J. Mater. Chem. A, 2016, 4, 838–846 RSC.
- B. M. Pirzada, Pushpendra, R. K. Kunchala and B. S. Naidu, ACS Omega, 2019, 4, 2618–2629 CrossRef CAS PubMed.
- L. Y. Wang, G. W. Hu, Z. Y. Wang, B. D. Wang, Y. Song and H. A. Tang, RSC Adv., 2015, 5, 73327–73332 RSC.
- K. Iqbal, A. Iqbal, A. M. Kirillov, C. F. Shan, W. S. Liu and Y. Tang, J. Mater. Chem. A, 2018, 6, 4515–4524 RSC.
- K. Iqbal, A. Iqbal, A. M. Kirillov, W. S. Liu and Y. Tang, Inorg. Chem., 2018, 57, 13270–13278 CrossRef CAS PubMed.
- X. Wang, D. P. Liu, S. Y. Song and H. J. Zhang, J. Am. Chem. Soc., 2013, 135, 15864–15872 CrossRef CAS PubMed.
- J. Liu, J. F. Hao, C. C. Hu, B. J. He, J. B. Xi, J. W. Xiao, S. Wang and Z. W. Bai, J. Phys. Chem. C, 2018, 122, 2696–2703 CrossRef CAS.
- K. Iqbal, A. Iqbal, A. M. Kirillov, B. K. Wang, W. S. Liu and Y. Tang, J. Mater. Chem. A, 2017, 5, 6716–6724 RSC.
- Q. Zhao, Z. H. Yan, C. C. Chen and J. Chen, Chem. Rev., 2017, 117, 10121–10211 CrossRef CAS PubMed.
- T. W. Zhang, Z. F. Li, Z. X. Zhang, L. K. Wang, P. Sun and S. W. Wang, J. Phys. Chem. C, 2018, 122, 27469–27476 CrossRef CAS.
- T. T. Zhou, X. P. Liu, R. Zhang, L. L. Wang and T. Zhang, Adv. Mater. Interfaces, 2018, 5, 1800115 CrossRef.
- T. T. Zhou, X. P. Liu, R. Zhang, Y. B. Wang and T. Zhang, ACS Appl. Mater. Interfaces, 2018, 10, 37242–37250 CrossRef CAS PubMed.
- P. Wu, S. Cheng, M. H. Yao, L. F. Yang, Y. Y. Zhu, P. P. Liu, Q. Xing, J. Zhou, M. K. Wang, H. Luo and M. L. Liu, Adv. Funct. Mater., 2017, 27, 1702160 CrossRef.
- F. Lu, M. Zhou, K. Su, T. Ye, T. J. Yang, T. D. Lam, Y. Bando and X. Wang, ACS Appl. Mater. Interfaces, 2019, 11, 2082–2092 CrossRef CAS PubMed.
- Y. Y. Wang, Z. Y. Zhang, X. Liu, F. Ding, P. Zou, X. X. Wang, Q. B. Zhao and H. B. Rao, ACS Sustainable Chem. Eng., 2018, 6, 12511–12521 CrossRef CAS.
- Y. L. Dai, V. P. Kumar, C. J. Zhu, H. Y. Wang, K. J. Smith, M. O. Wolf and M. J. MacLachlan, Adv. Funct. Mater., 2019, 29, 1807519 CrossRef.
- X. H. Gao, H. X. Zhang, Q. G. Li, X. Yu, Z. Hong, X. W. Zhang, C. D. Liang and Z. Lin, Angew. Chem., Int. Ed., 2016, 55, 6290–6294 CrossRef CAS PubMed.
- L. Y. Hu, C. L. Dai, H. Liu, Y. Li, B. L. Shen, Y. M. Chen, S. J. Bao and M. W. Xu, Adv. Energy Mater., 2018, 1800709 CrossRef.
- J. M. Son, S. Oh, S. H. Bae, S. Nam and I. K. Oh, Adv. Energy Mater., 2019, 1900477 CrossRef.
- H. Li, J. Li, Z. H. Ai, F. L. Jia and L. Z. Zhang, Angew. Chem., Int. Ed., 2018, 57, 122–138 CrossRef CAS PubMed.
- R. J. Zeng, Z. B. Luo, L. S. Su, L. J. Zhang, D. P. Tang, R. Niessner and D. Knopp, Anal. Chem., 2019, 91, 2447–2454 CrossRef CAS PubMed.
- Y. J. Wang, J. R. Jin, W. G. Chu, D. Cahen and T. He, ACS Appl. Mater. Interfaces, 2018, 10, 15304–15313 CrossRef CAS PubMed.
- L. Wang, D. D. Lv, F. Dong, X. L. Wu, N. Y. Cheng, J. Scott, X. Xu, W. C. Hao and Y. Du, ACS Sustainable Chem. Eng., 2019, 7, 3010–3017 CrossRef CAS.
- J. Di, C. Zhu, M. X. Ji, M. L. Duan, R. Long, C. Yan, K. Z. Gu, J. Xiong, Y. B. She, J. X. Xia and H. M. Li, Angew. Chem., Int. Ed., 2018, 57, 14847–14851 CrossRef CAS PubMed.
- H. Li, J. Shang, H. J. Zhu, Z. P. Yang, Z. H. Ai and L. Z. Zhang, ACS Catal., 2016, 6, 8276–8285 CrossRef CAS.
- J. Jiang, K. Zhao, X. Y. Xiao and L. Z. Zhang, J. Am. Chem. Soc., 2012, 134, 4473–4476 CrossRef CAS PubMed.
- J. Yang, C. Yu, S. X. Liang, S. F. Li, H. W. Huang, X. T. Han, C. T. Zhao, X. D. Song, C. Hao, P. M. Ajayan and J. S. Qiu, Chem. Mater., 2016, 28, 5855–5863 CrossRef CAS.
- C. Mahala and M. Basu, ACS Omega, 2017, 2, 7559–7567 CrossRef CAS PubMed.
- S. Chakrabarty, A. Mukherjee and S. Basu, ACS Sustainable Chem. Eng., 2018, 6, 5238–5247 CrossRef CAS.
- H. G. Yu, C. Cao, X. F. Wang and J. G. Yu, J. Phys. Chem. C, 2017, 121, 13191–13201 CrossRef CAS.
- C. J. Huang, J. L. Hu, S. Cong, Z. Z. Zhao and X. Q. Qiu, Appl. Catal., B, 2015, 174, 105–112 CrossRef.
- D. Yan, W. Wang, X. Luo, C. Chen, Y. Zeng and Z. H. Zhu, Chem. Eng. J., 2018, 334, 864–872 CrossRef CAS.
- H. Tong, S. H. Yue, L. Lu, F. Q. Jin, Q. W. Han, X. G. Zhang and J. Liu, Nanoscale, 2017, 43, 16826–16835 RSC.
- Y. X. You, M. J. Zheng, D. K. Jiang, F. G. Li, H. Yuan, Z. H. Zhai, L. Ma and W. Z. Shen, J. Mater. Chem. A, 2018, 18, 8742–8749 RSC.
- Q. X. Zhang, P. Chen, M. H. Zhuo, F. L. Wang, Y. H. Su, T. S. Chen, K. Yao, Z. W. Cai, W. W. Lv and G. G. Liu, Appl. Catal., B, 2018, 221, 129–139 CrossRef CAS.
- J. X. Xia, J. Di, H. T. Li, H. Xu, H. M. Li and S. J. Guo, Appl. Catal., B, 2016, 181, 260–269 CrossRef CAS.
- Y. X. Liu, Y. Bai, Y. Han, Z. Yu, S. M. Zhang, G. H. Wang, J. H. Wei, Q. B. Wu and K. N. Sun, ACS Appl. Mater. Interfaces, 2017, 9, 36917–36926 CrossRef CAS PubMed.
- C. Guan, X. M. Liu, W. N. Li, X. Ren, C. W. Cheng and J. Wang, Adv. Energy Mater., 2017, 12, 1602391 CrossRef.
- F. X. Ma, L. Yu, C. Y. Xu and X. W. Lou, Energy Environ. Sci., 2016, 9, 862–866 RSC.
- Z. Yang, W. W. Tjiu, W. Fan and T. X. Liu, Electrochim. Acta, 2013, 90, 400–407 CrossRef CAS.
- Y. Zheng and A. Q. Wang, J. Mater. Chem., 2012, 22, 16552–16559 RSC.
Footnote |
† Electronic supplementary information (ESI) available: EDX, XPS and UV-vis spectra. See DOI: 10.1039/c9nj05100g |
|
This journal is © The Royal Society of Chemistry and the Centre National de la Recherche Scientifique 2020 |
Click here to see how this site uses Cookies. View our privacy policy here.