DOI:
10.1039/C9NJ04755G
(Paper)
New J. Chem., 2020,
44, 102-109
Self-assembled star-shaped aza-BODIPY mesogen affords white-light emission†
Received
18th September 2019
, Accepted 18th November 2019
First published on 19th November 2019
Abstract
A novel multifunctional star-shaped aza-BODIPY mesogen was synthesized by a click reaction. This star-shaped aza-BODIPY mesogen undergoes self-assembly into a hexagonal columnar phase in its bulk state and spherical gels in organic solvents. Based on the investigation of the absorption and emission spectra and surface morphologies, J-aggregates are observed in their liquid crystalline (LC), gel and solid states, while H-aggregates are observed in n-hexane solution. Additionally, this star-shaped aza-BODIPY mesogen acts as a chemosensor toward CN− ions via a nucleophilic addition reaction, and the corresponding addition product can yield white light emission (WLE) upon doping with blue dye. This star-shaped aza-BODIPY mesogen is represented as the first example of an aza-BODIPY derivative with LC, organogel and white light emission properties.
1. Introduction
The self-assembly of organic dyes has provided a powerful tool to design novel nano-functional materials with distinct optical and electrochemical properties. By controlling the non-covalent interactions of photo- and redox-active dye molecules, extended superstructures up to mesophase materials and organogels1 with controlled exciton coupling and energy transport in π-stacked materials2,3 can be realized. Among the materials realized so far, J-aggregates4 have attracted considerable attention because of their excellent photophysical characteristics with strong and narrow J-bands, a long exciton lifetime and high fluorescence quantum yields.5 J-aggregates have been found in several π-conjugated systems, such as oligo(p-phenylenevinylenes) (OPVs),6 porphyrins,7 merocyanines,8 boradiazaindacenes (BODIPYs),9 perylene bisimides,10 and 8-aza-boradiazaindacenes (aza-BODIPY) dyes.11,12 A few examples of J-aggregates have also been observed in thermotropic liquid-crystalline (LC) phases of perylene bisimides,2b,13,14 porphyrins,15 and ionic cyanine dyes.16 Dye aggregates are capable of producing new optical or electronic functions that are not possible in a single dye molecule. Therefore, it is very important to study the aggregation states of dyes.
Aza-BODIPY dye is derived from BODIPY where the meso-carbon is replaced by nitrogen. Compared with BODIPY, aza-BODIPY shows ∼100 nm red shift in the absorption region of 600–800 nm.17 Thus, aza-BODIPY shows all the important features of BODIPY such as narrow absorption and emission bands with high molar absorption coefficients and fluorescence quantum yields in the visible-NIR region which are essential for their applications in photodynamic therapy (PDT), sensors and photosynthetic model systems.18 The relatively easy access to specific derivatives has now enabled aza-BODIPYs to be applied in diverse technologies extending from real-time fluorescence imaging to solar energy materials, and optoelectronic devices. To date, although a few BODIPY based liquid crystals and organogels with excellent photophysical performance have been reported,19–21 to the best of our knowledge, aza-BODIPY based liquid crystals and organogels have never been reported. Therefore it is significantly worthwhile to develop aza-BODIPY based LCs and organogels with the purpose of obtaining novel functional materials.
Click chemistry namely Cu(I) catalyzed azide–alkyne cycloaddition (CuAAC)22 provides an easy access to obtain novel aza-BODIPY NIR dyes for various applications. For example click reactions have been used for the attachment of multi-donor BODIPY chromophores to a central aza-BODIPY acceptor as a dendritic BODIPY antenna for light harvesting.23 A new triad (BDP-ADP-C60) in which an ADP (Aza-boron-dipyrrin) core is covalently linked to a BDP (a monostyryl boron-dipyrrin) moiety via a click reaction and a C60 unit using the Prato reaction in its two side arms can serve as an artificial photosynthetic model.24 The water-soluble aza-BODIPY derivatives are synthesized by attaching tetraethylene-glycerol groups to the central aza-BODIPY via a click reaction which could serve as near-infrared (NIR) emitting fluorescent probes.25
White-light emissive materials have drawn widespread attention owing to their potential in day-to-day lighting systems and display media.26–28 In general, white-light emission can be fabricated by mixing three primary colors (red, green and blue)29 or two complementary colors (yellow and blue)30via a dry or solution process. So far as we know, no aza-BODIPYs have been used to generate WLE materials.
Considering the great potential application of aza-BODIPYs, here the first example of a star-shaped aza-BODIPY columnar mesogen consisting of an aza-BODIPY core surrounded by four flexible 1,2,3-triazole dendritic paddles was synthesized efficiently via the CuAAC click reaction. This compound undergoes self-assembly into a thermotropic hexagonal columnar mesophase and spherical organogels. Optical and morphological investigation unveils that J-aggregates can be observed in its LC, solid and gel states, while H-aggregates can be observed in its hexane solution. Furthermore this compound can serve as a chemosensor toward the cyanide ion (CN−), which is the most toxic inorganic anion for living organisms, via nucleophilic addition.31 Most interestingly, the obtained addition product can yield white light emission upon doping with thiophene acetylene bolaamphiphile. Thus LC, organogel and WLE properties were observed simultaneously in this aza-BODIPY derivative.
2. Results and discussion
2.1. Materials and methods
Commercially available reagents are used as received. Solvents were dried and distilled prior to use based on previously reported procedures.321H NMR and 13C NMR spectra were recorded on Bruker-DRX-400 and Bruker-DRX-600 spectrometers. Elemental analysis was performed by using an Elementar VARIO EL elemental analyzer. Thin-layer chromatography (TLC) was carried out on aluminum plates pre-coated with 5735 silica gel 60 PF254 (Merck). Column chromatography was performed with Merck silica gel 60 (230–400 mesh). A Mettler heating stage (FP 82 HT) was used for polarizing optical microscopy (POM, Optiphot 2, Nikon) and DSC data were recorded with a DSC 200 F3 Maia calorimeter (NETZSCH) at 10 K min−1. Their optical properties (UV-vis and fluorescent emission) were studied on UNIC UV2600A and HITACHI F-7000 spectrophotometers.
SEM experiments were performed on a ZEISS SIGMA 300 scanning electron microscope (SEM, GER). All pictures were taken digitally. For sample preparation, the gel was placed on an aluminium foil for some time until the gel became dry, and then the sample was gold plated. Finally the sample was used for SEM analysis.33
The small-angle powder diffraction (SAXS) experiment was performed in transmission mode with synchrotron radiation at the 1W2A SAXS beamline and the beamline BL16B1 at the Shanghai Synchrotron Radiation Facility (SSRF).34 A modified Linkam hot stage with thermal stability within 0.2 °C was used, with a hole for the capillary drilled through the silver heating block and mica windows attached to it on each side. The samples were held in a poly(imide) (Kapton) film. A MarCCD 165 detector was used. The q calibration and linearization were verified using several orders of layer reflections from silver behenate. Positions and intensities of the diffraction peaks were measured using PeakSolve™ (Galactic).
For electron density reconstruction, Fourier reconstruction of the electron density was carried out using the general formula for 2D periodic systems:
E(xy) = Σhk sqrt[I(hk)] exp[i2π(hx + ky) + Φhk] |
For the centrosymmetric structures considered in this work, the phase angle Φ can take up the values of 0 or π. The choice of a phase combination was initially made depending on the merit of each reconstructed electron density map obtained using the most intense reflections, combined with the additional knowledge of the molecules (molecular shape, length, volume of each part and the distribution of electron density among the different moieties).
2.2. Synthesis
The target compound ADP/12 was synthesized by the Michael reaction and the click reaction23 as the key steps (Scheme 1). Benzaldehyde 1 and acetophenone 2 were obtained by etherification of 4-hydroxybenzaldehyde and 4-hydroxyacetophenone with propargyl bromide, respectively. Benzaldehyde 1 reacted with acetophenone 2 giving diaryl α,β-unsaturated ketone 3. Nitromethane was added to the α,β-unsaturated ketone 3via Michael addition. The obtained 1,3-diaryl-4-nitrobutanone 4 was condensed with ammonium acetate giving aza-dipyrrin 5. Complexation of aza-dipyrrin 5 with BF3·Et2O in the presence of diisopropylethylamine (DIPEA) gave terminal alkyne 6. The final product ADP/12 was obtained by a click reaction between the terminal alkyne 6 and azide 7.
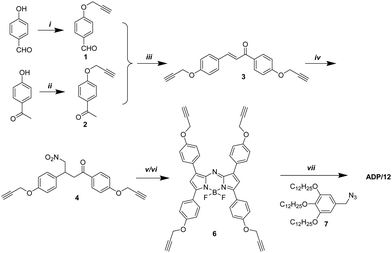 |
| Scheme 1 Synthesis of compounds ADP/12. Reagents and conditions: (i) 3-bromopropyne, acetone, K2CO3, 90 °C, 8 h, 93%; (ii) 3-bromopropyne, DMF, K2CO3, 90 °C, 8 h, 94%; (iii) C2H5OH, KOH, H2O, RT, 6 h, 89%; (iv) CH3NO2, (C2H5)2NH, CH3OH, reflux, 12 h, 76%; (v) NH4OAc, C2H5OH, 80 °C, 48 h; (vi) BF3·Et2O, DIPEA, CH2Cl2, RT, 10 h, 31%; (vii) tert-butanol, THF, H2O, sodium ascorbate, CuSO4·5H2O, RT, 12 h, 75%. | |
2.3. Mesomorphic properties
The mesomorphic behavior of ADP/12 was investigated by polarizing optical microscopy (POM), differential scanning calorimetry (DSC) and X-ray diffraction (XRD). The phase transition is summarized in Fig. 1. The color of the sample was deep blue, under POM, and no texture could be observed. The sample had high viscosity but could flow under shearing. Upon heating the sample to 99.4 °C, the viscosity of the sample was suddenly lost and the sample changed into flowing liquid. Upon cooling the sample to 75.4 °C, the sample showed high viscosity again. The DSC curve of ADP/12 is shown in Fig. S1 (ESI†). During the heating cycle, it showed a mesophase–isotropic transition with an enthalpy change of 0.39 kJ mol−1 at 99.4 °C, while during the cooling cycle, it showed an isotropic– liquid mesophase transition with an enthalpy change of 1.05 kJ mol−1 at 75.4 °C. This is in accordance with the POM observation, and therefore ADP/12 is an enantiotropic (thermodynamically stable) room temperature LC.
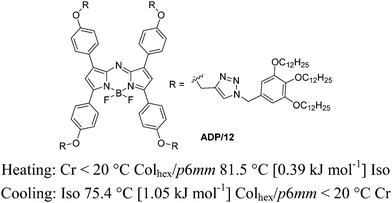 |
| Fig. 1 Structure of compound ADP/12 with transition temperatures (T/°C) and the associated enthalpy values as determined by DSC (peak temperatures, 10 K min−1); Cr = crystal; Colhex = hexagonal columnar phase; and Iso = isotropic liquid. | |
The mesomorphic phase was further investigated with SAXS. The XRD pattern of the columnar phase of the compound ADP/12 showed three small-angle reflections, and their reciprocal spacings in a ratio of 1
:
31/2
:
2, which are consistent with the 10, 11, 20 reflections of the hexagonal lattice with p6mm symmetry. The number of molecules (μ) in the slice of the columns with a height of h = 0.45 nm (the maximum of the measured diffuse wide-angle scattering ddiff) is 1 (Table S3, ESI†). This indicates that one molecule organizes into a one-dimensional (1D) disk-like stratum, and then the 1D disks stake into columns which further arrange into a 2D hexagonal columnar mesophase. It is worth noting that the intercolumnar distance (namely the lattice parameter a = 4.34 nm) of ADP/12 is much smaller than the fully extended molecular length (L = 6.8 nm, calculated by MM235). In this way, the discotic molecule should be tilted with respect to the cross-section of each disk-like stratum. From the longitudinal offset, the tilt superposition angle in the column was estimated to be θ = 42° in the Colhex phase with respect to the long axis of the column.3 Such a packing model (Fig. 2) is in correspondence with π-stacked aggregates with a J-type parallel stacking mode in the LC state based on the spectral studies (see Fig. 4 in the section of photophysical properties and aggregation in LC, gel and solvents).
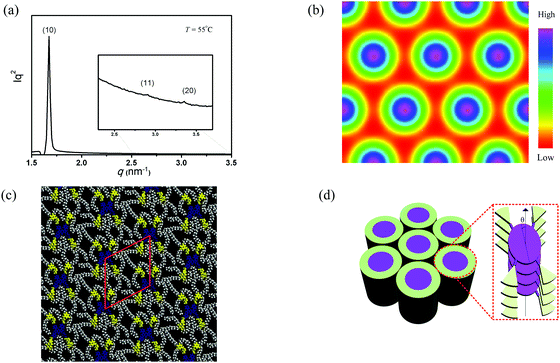 |
| Fig. 2 Colhex/p6mm phase of ADP/12 (a) XRD patterns at T = 55 °C; (b) reconstructed electron density map of the Colhex/p6mm phase; (c) snapshot of the Colhex/p6mm phase after MD annealing; and (d) the model for the Colhex phase. | |
2.4. Gel self-assembly
The gelation test of the compound ADP/12 was performed in several organic solvents as shown in Table 1. The gels were prepared by cooling the heated solution of ADP/12 (10 mg ml−1) quickly below 20 °C or slowly down to room temperature. And the method “stable to inversion of the container”36 was used to judge gel formation. The compound ADP/12 could form gels in organic solvents such as ethyl acetate and DMF. The morphologies of the xerogels were observed by SEM. The SEM image of the xerogel formed in EA showed irregular nanospheres with an average diameter of 6–20 μm (see Fig. 3), while the xerogel formed in DMF showed more regular nanospheres with an average diameter of 4 μm.
Table 1 Gelation test results of compounds ADP/12a
Solvent |
ADP/12
|
Solvent |
ADP/12
|
G = gel, S = soluble, and P = precipitation.
|
Ethyl acetate |
G |
Chloroform |
S |
Acetone |
P |
n-Hexane |
S |
DMF |
G |
Petroleum ether |
P |
Toluene |
S |
1,4-Dioxane |
S |
Acetonitrile |
P |
Methanol |
P |
Dichloromethane |
S |
Ethanol |
P |
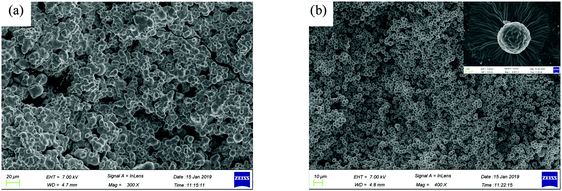 |
| Fig. 3 SEM images of the xerogels (a) ADP/12 in ethyl acetate; scale bar is 20 μm, (b) ADP/12 in DMF; scale bar is 10 μm, and the inset shows ADP/12 in DMF; scale bar is 1 μm. | |
2.5. Photophysical properties and aggregation in LC, gel and solvents
Dye aggregates with a narrow absorption band that is shifted to a longer wavelength (bathochromically shifted) compared with the monomer absorption band are usually referred to as Scheibe aggregates or J-aggregates (J denotes Jelley).37,38 Aggregates with absorption bands shifted to a shorter wavelength (hypsochromically shifted) compared with the monomer band are termed as H-aggregates39 (H denotes hypsochromic shift). For the compound ADP/12, the UV-vis absorption and fluorescence spectra in THF solution (1 × 10−5 M) are shown in Fig. S3 (ESI†). ADP/12 shows a Q-band between 600 and 750 nm with an absorption maximum at 694 nm, which coincides with that of other aza-BODIPY dyes.40 The emission spectrum is nearly a mirror image of the absorption spectrum, and the maximum emission in THF is at 735 nm.
To gain an insight into the solvatochromic behavior of ADP/12, the UV-vis absorption and fluorescence emission were investigated in different organic solvents (see Fig. 4), and the photophysical data are summarized in Table 2. As shown in Fig. 4a, the UV-vis absorption spectrum of ADP/12 is less influenced by the solvent polarity exhibiting 688–697 nm for solvents like CH2Cl2, THF, toluene and DMF,whereas the fluorescence spectra of ADP/12 exhibit a positive solvatochromism (red-shifted) with an increase of the polarities of the solvents from 731 nm (CH2Cl2) to 734 nm (toluene) to 735 nm (THF) and 744 nm (DMF) with Stokes shifts of 41–47 nm. ADP/12 shows fluorescence quantum yields (ΦFL) from 0.37 to 0.39 in different polarity organic solvents relative to rhodamine B in ethanol (ΦFL = 0.67). Interestingly, a broad spectrum with a blue-shifted absorption band of ADP/12 in n-hexane (1 × 10−6–5 × 10−5 M) was observed, while the luminescence was totally quenched, indicating that H-aggregates were formed in n-hexane. Furthermore, the SEM image (Fig. 4d) of ADP/12 in n-hexane reveals the presence of irregular spherical aggregates (an average diameter of 7–9 μm), which were different in both the shape and size as observed from the morphologies for the xerogels (Fig. 3). The UV-vis absorption spectrum of ADP/12 in the film, LC (40 °C) and gel states was also recorded. The film exhibits a broad and intensive absorption band in the range of 600–750 nm with an absorption maximum at 713 nm. The absorption peaks in the solid and LC states are red-shifted by 25 nm and 23 nm, respectively, compared to those in THF solution, which indicated the formation of π-stacked aggregates with a J-type parallel stacking mode in the solid and LC states. Combining all experimental evidence, different packing models of ADP/12 have been proposed in Scheme 2.
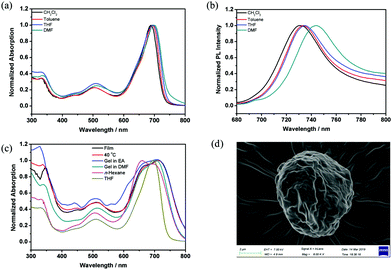 |
| Fig. 4 (a) The absorption spectra of ADP/12 in different solvents and (b) the emission spectra of ADP/12 in different solvents. (c) The absorption spectra of ADP/12 in different states and (d) the SEM images of ADP/12 solution in n-hexane. | |
Table 2 UV-vis absorption and fluorescence spectroscopy data of ADP/12 (1 × 10−5 M) at 20 °C
Comp. |
State |
λ
ex/nm |
λ
em/nm |
Φ
FL
|
Stokes shiftsb (nm) |
Relative to rhodamine B in ethanol (ΦFL = 0.65) as the standard.
Stokes shifts = λem − λex.
Not measured.
|
ADP/12
|
THF |
694 |
735 |
0.38 |
41 |
n-Hexane |
659 |
—c |
— |
— |
CH2Cl2 |
688 |
731 |
0.37 |
43 |
Toluene |
693 |
734 |
0.38 |
41 |
DMF |
697 |
744 |
0.39 |
47 |
Film |
713 |
— |
— |
— |
LC (40 °C) |
711 |
— |
— |
— |
Gel (EA) |
707 |
— |
— |
— |
Gel (DMF) |
704 |
— |
— |
— |
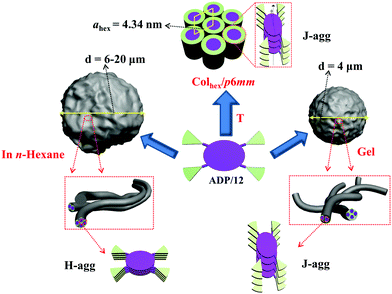 |
| Scheme 2 Proposed packing models for the self-assembly of ADP/12. | |
2.6. Chemosensor behavior
The active meso C
N bond of aza-BODIPY is prone to nucleophilic attack by anions. Therefore, aza-BODIPY can serve as an anion sensor based on a chemical reaction.31a Thus we tested the anion sensing behavior of aza-BODIPY with various anions. After ten equivalents of different anions, including Br−, Cl−, I−, HSO4−, NO2−, N3−, CO32−, NO3−, F−, H2PO4−, AcO− and CN−, were added to ADP/12 solution (1 × 10−5 M), respectively, a change in the color from blue to light yellow under daylight (Fig. S4b, ESI†) and yellow colour emission under UV light could be observed with the naked eye in the presence of CN−, while no visible color changes were noticed in the case of the other anions (Fig. 5c). This indicates that compound ADP/12 can be used as a specific sensor for CN−.
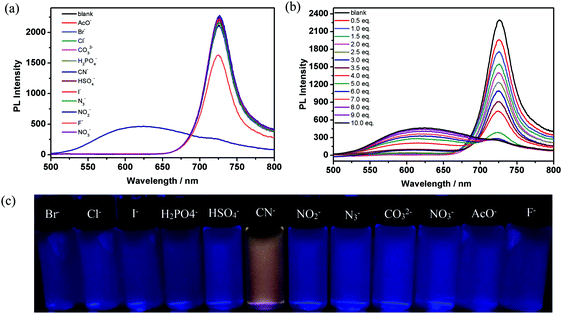 |
| Fig. 5 (a) The fluorescence response of ADP/12 upon addition of different anions. (b) Fluorescence titration spectra of ADP/12 in THF (1 × 10−5 M) upon addition of different amounts of CN− (TBACN) ion (0–10 equiv.) (λex = 430 nm), and (c) the samples with addition of different metal ions, under irradiation with 365 nm light. | |
The fluorescence profiles of ADP/12 in the presence of 10 equiv. of the selected anions in THF solution were investigated. Upon the addition of CN−, the absorption spectrum of ADP/12 was largely quenched at 694 nm and enhanced at 445 nm (Fig. S4a, ESI†). The fluorescence of ADP/12 was largely quenched at 726 nm and significantly enhanced at 630 nm (Fig. 5a). We also tested the fluorescence spectra of ADP/12 in the presence of other anions but there was no significant change in both the absorption and fluorescence spectra.
To assess the binding properties of ADP/12 to CN−, fluorescence titration curves of ADP/12 were obtained in THF solution. Upon increasing the addition amount of CN− (from 1 to 10 equiv.), the emission intensity of ADP/12 at 726 nm slowly decreased while the emission band at 630 nm increased (Fig. 5b). For a better understanding of the coordination of ADP/12 with CN−, fluorescence titration in the case of varying mole fractions of CN− ion in THF solution was carried out. The fluorescence Job's plot analysis showed 1
:
1 stoichiometry between ADP/12 and CN− (Fig. S5b, ESI†). The detection limit of ADP/12 to CN− was measured based on 3σ/k to be 3.69 × 10−5 mol L−1.41 It is well-known that some food contains cyanogenic glycosides, such as bitter almond,42 cassava43 and sprouting potato.44 The cyanide is generated by enzymatic hydrolysis of cyanogenic glycosides when their tissues are damaged. So we further explored the potential application of ADP/12 for determining cyanide in a food sample. Thus, direct detection of CN− was further studied on a sprouting potato. The sprouting potato was cut into fresh slices at room temperature. After being incubated with ADP/12 solution, it did not show any significant change in color under daylight (Fig. 6a and c). However, a remarkable yellow–green color was observed under UV light (365 nm) (Fig. 6b and d). According to a previous report, the C
N bond of aza-BODIPY can undergo a nucleophilic addition reaction with CN−.31a Therefore a nucleophilic addition product ADP-CN was supposed to be formed. However many efforts to run the 1H NMR experiment for this nucleophilic addition product failed. Only unclear and broader peaks were observed in the 1H NMR spectra for this nucleophilic addition product. Therefore we have turned to verify the nucleophilic addition product between intermediate 6 and CN−. Intermediate 6 contained the same C
N bond of aza-BODIPY but without a triazole group and a flexible alkyl chain. The results showed that compound 6 showed similar spectral changes as compound ADP/12 (Fig. S6, ESI†) upon addition of different anions. The 1H NMR spectra of 6 in the absence and presence of different equivalents of TBACN (tetrabutylammonium cyanide) in CDCl3 were recorded as shown in Fig. 7. The 1H NMR spectrum of compound 6 showed that the CH protons of the pyrrole rings appeared at 6.99 ppm. The aryl protons appeared as two sets of multiplets in the region of 8.03–8.08 ppm and 7.06–7.10 ppm, and the methylene of the OCH2 protons appeared as multiplets at 4.76–7.79 ppm and the alkyne protons appeared as two singlets at 2.60 ppm and 2.57 ppm. The intermediate 6 is a symmetrical compound. After addition of CN− ion to the intermediate 6, the resulting product 6-CN showed an asymmetric structure (Fig. S7, ESI†). Thus the CH protons of the pyrrole rings changed into two singlets at 6.54 ppm and 6.64 ppm, respectively. The aryl protons changed into eight sets of doublets in the region of 6.81–8.16 ppm (Fig. 7b–d). Upon increasing the amount of cyanide ions, the peak of the alkyne protons gradually reduced and the peak of the methylene protons of N–CH2 from TBACN gradually increased (Fig. 7a–d). When 1.0 equiv. of CN− was added into 6, the peak of the alkyne protons completely disappeared. The methylene protons of OCH2 appeared as four sets of singlets in the region of 4.61–4.75 ppm (Fig. 7d). With the disappearance of alkyne protons, the small coupling between alkyne protons and ArOCH2 methylene became much weaker. When 6 and TBACN formed a 1
:
1 mixture, the small coupling completely disappeared, and thus the methylene protons of OCH2 appeared as singlets instead of multiplets. Based on this experiment, it could be concluded that a nucleophilic addition reaction between the C
N bond of aza-BODIPY and CN− ion could be observed, and after the nucleophilic addition reaction with CN−, addition products 6-CN and ADP-CN could be obtained.
 |
| Fig. 6 Photographs taken under ambient light (a and c) and UV light (b and d): (a and b) a freshly cut potato slice; (c and d) after incubating the potato slice with ADP/12 solution. | |
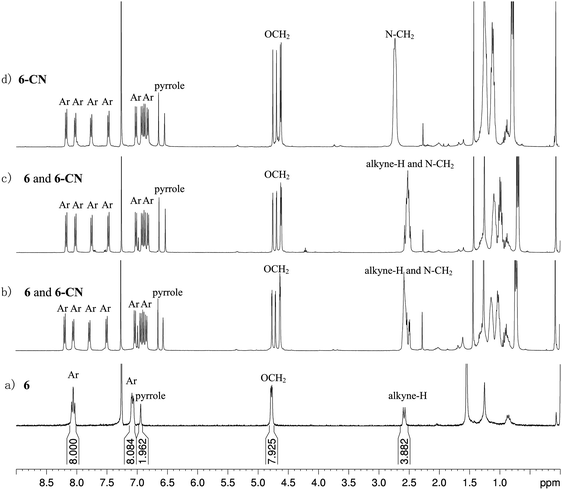 |
| Fig. 7
1H NMR (400 MHz, CDCl3) spectra of (a) free compound 6; (b) 0.4 equiv. of CN− added into 6; (c) 0.7 equiv. of CN− added into 6; and (d) 1.0 equiv. of CN− added into 6. | |
2.7. White light emission
Both the addition products 6-CN and ADP-CN obtained by the above mentioned nucleophilic addition reactions between CN− ion and the intermediate 6 or ADP/12 were separated and their optical properties were studied. Both 6-CN and ADP-CN emitted yellow colours in THF (500–800 nm). Their CIE coordinates were found to be (0.59, 0.41) (Fig. S9, ESI†) and (0.56, 0.43) (Fig. 8), respectively. Therefore, they could generate white-light emission upon blending with a dopant emitting complementary color (blue emission). We selected our previously reported blue emissive 5,5′-bis(phenylethynyl)-2,2′-bithiophene-based bolaamphiphile 2ET/1245 as a dopant. The CIE coordinates of 6-CN and 2ET/12 (0.14, 0.23) or ADP-CN and 2ET/12 can be connected in line with the coordinates of the white light area. The absorption spectrum of 6-CN showed a band around 394–568 nm and ADP-CN showed two bands around 403–597 nm and 697–800 nm, respectively. The emission spectra of 2ET/12 showed emission peaks centered at 465 nm. The partial overlap of the spectrum of 2ET/12 and 6-CN (or ADP-CN) indicated energy transfer from 2ET/12 to 6-CN (or ADP-CN) solutions (in THF) and also possibility to tune their emission colors (Fig. S8, ESI†). Two mixtures were prepared by doping 0.01 mL of 2ET/12 solution (1 × 10−4 mol L−1 in THF) into 3.5 mL 6-CN or ADP-CN solution (1 × 10−5 mol L−1 in THF). The emission spectrum of the two mixtures covered the spectral range of 400–750 nm (Fig. 8a). The CIE coordinates were found to be (0.33, 0.31) for 2ET/12 + 6-CN (Fig. S9, ESI†) or (0.33, 0.33) for 2ET/12 + ADP-CN, respectively (Fig. 8b). The CIE coordinates of 2ET/12 + 6-CN were quite close to those of the ideal white light emission (0.33, 0.33), while the CIE coordinates of 2ET/12 + ADP-CN were those of the ideal white light emission.46 Such anion tunable WLE should have great potential for detection of the most toxic inorganic anion CN− in living organisms and fabrication of stimuli responsive devices.
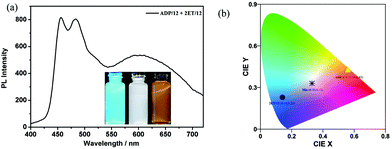 |
| Fig. 8 (a) Emission spectrum of the gel of ADP-CN (1 × 10−5 M) + 2ET/12 (1 × 10−5 M); the inset shows the photo of the 2ET/12, ADP-CN + 2ET/12, ADP-CN solutions in THF under 365 nm UV light and (b) the CIE coordinates of ADP-CN + 2ET/12. | |
3. Conclusion
In summary, a novel star-shaped aza-BODIPY mesogen has been prepared effectively by a click reaction. Such an aza-BODIPY mesogen undergoes self-assembly into a hexagonal columnar liquid crystalline phase and spherical organogels. Further J-aggregates are observed in its LC, gel and solid states, while H-aggregates are observed in n-hexane solution. Additionally, this compound and the intermediate 6 act as chemosensors toward CN− ions via nucleophilic addition reactions, and the corresponding addition products can yield white light emission upon doping with blue dye. The present investigation provided a new method to obtain a novel aza-BODIPY mesogen with white-light emission. The excellent self-assembly and photoelectric properties indicate that such a columnar aza-BODIPY liquid crystal shows great potential in many fruitful applications.
Conflicts of interest
There are no conflicts to declare.
Acknowledgements
This work was financially supported by the National Natural Science Foundation of China (No. 21865038 and 21664015) and the program for Innovative Research Team (in Science and Technology) in University of Yunnan Province (2018FY001(-012)). We thank beamline 1W2A at the Beijing Accelerator Laboratory and beamline BL16B1 at the Shanghai Synchrotron Radiation Facility (SSRF), China.
References
-
(a) L. H. Zeng, P. S. Liao, H. L. Liu, L. P. Liu, Z. W. Liang, J. Y. Zhang, L. P. Chen and C. Y. Su, J. Mater. Chem. A, 2016, 4, 8328–8336 RSC
;
(b) T. Ishi-i and S. Shinkai, Top. Curr. Chem., 2005, 258, 119–160 CrossRef CAS
;
(c) E. Arunkumar, C. C. Forbes and B. D. Smith, Eur. J. Org. Chem., 2005, 4051–4059 CrossRef CAS
.
-
(a) S. Yagai, Bull. Chem. Soc. Jpn., 2015, 88, 28–58 CrossRef
;
(b) T. Wöhrle, I. Wurzbach, J. Kirres, A. Kostidou, N. Kapernaum, J. Litterscheidt, J. C. Haenle, P. Staffeld, A. Baro, F. Giesselmann and S. Laschat, Chem. Rev., 2015, 116, 1139–1241 CrossRef PubMed
;
(c) A. Kaeser and A. P. H. J. Schenning, Adv. Mater., 2010, 22, 2985–2997 CrossRef CAS PubMed
;
(d) M. Lehmann and M. Hügel, Angew. Chem., Int. Ed., 2015, 54, 4110–4114 CrossRef CAS PubMed
.
- F. Würthner, C. Thalacker, S. Diele and C. Tschierske, Chem. – Eur. J., 2001, 7, 2245–2253 CrossRef
.
- F. Würthner, T. E. Kaiser and C. R. Saha-Möller, Angew. Chem., Int. Ed., 2011, 50, 3376–3410 (
Angew. Chem., Int. Ed.
, 2011
, 123
, 3436–3473
) CrossRef PubMed
.
-
(a) D. M. Eisele, J. Knoester, S. Kirstein, J. P. Rabe and D. A. Vanden Bout, Nat. Nanotechnol., 2009, 4, 658–663 CrossRef CAS PubMed
;
(b) H. Z. Lin, R. Camacho, Y. X. Tian, T. E. Kaiser, F. Würthner and I. G. Scheblykin, Nano Lett., 2010, 10, 620–626 CrossRef CAS PubMed
.
-
(a) S. Yagai, S. Okamura, Y. Nakano, M. Yamauchi, K. Kishikawa, T. Karatsu, A. Kitamura, A. Ueno, D. Kuzuhara, H. Yamada, T. Seki and H. Ito, Nat. Commun., 2014, 5, 4013 CrossRef CAS
;
(b) K. Sakakibara, P. Chithra, B. Das, T. Mori, M. Akada, J. Labuta, T. Tsuruoka, S. Maji, S. Furumi, L. K. Shrestha, J. P. Hill, S. Acharya, K. Ariga and A. Ajayaghosh, J. Am. Chem. Soc., 2014, 136, 8548–8551 CrossRef CAS PubMed
.
- S. Ogi, K. Sugiyasu, S. Manna, S. Samitsu and M. Takeuchi, Nat. Chem., 2014, 6, 188–195 CrossRef CAS PubMed
.
-
(a) A. Lohr, M. Lysetska and F. Würthner, Angew. Chem., Int. Ed., 2005, 44, 5071–5074 (
Angew. Chem., Int. Ed.
, 2005
, 117
, 5199–5202
) CrossRef CAS PubMed
;
(b) A. Lohr and F. Würthner, Angew. Chem., Int. Ed., 2008, 47, 1232–1236 (
Angew. Chem., Int. Ed.
, 2008
, 120
, 1252–1256
) CrossRef CAS PubMed
;
(c) A. Lohr and F. Würthner, Chem. Commun., 2008, 2227–2229 RSC
.
-
(a) A. Nagai, K. Kokado, J. Miyake and Y. Chujo, Polym. J., 2010, 42, 37–42 CrossRef CAS
;
(b) A. Nagai, K. Kokado, J. Miyake and Y. Chujo, Macromolecules, 2009, 42, 5446–5452 CrossRef CAS
.
-
(a) S. Ogi, V. Stepanenko, K. Sugiyasu, M. Takeuchi and F. Würthner, J. Am. Chem. Soc., 2015, 137, 3300–3307 CrossRef CAS
;
(b) X. J. Ma, Y. B. Zhang, Y. F. Zhang, C. Peng, Y. K. Che and J. C. Zhao, Adv. Mater., 2015, 27, 7746–7751 CrossRef CAS PubMed
;
(c) S. Ogi, V. Stepanenko, J. Theirs and F. Würthner, J. Am. Chem. Soc., 2016, 138, 670–678 CrossRef CAS PubMed
.
- Z. J. Chen, Y. Liu, W. Wagner, V. Stepanenko, X. K. Ren, S. Ogi and F. Würthner, Angew. Chem., Int. Ed., 2017, 56, 5729–5733 CrossRef CAS PubMed
.
- Y. Liu, Y. J. Zhang, F. Fennel, W. Wagner, F. Würthner, Y. F. Chen and Z. J. Chen, Chem. – Eur. J., 2018, 24, 16388–16394 CrossRef CAS
.
-
(a)
Handbook of Liquid Crystals, ed. J. W. Goodby, P. J. Collings, T. Kato, C. Tschierske, H. Gleeson and P. Raynes, Wiley-VCH, Weinheim, 2nd edn, 2014, 7, 602 Search PubMed
;
(b) T. Kato, N. Mizoshita and K. Kishimoto, Angew. Chem., Int. Ed., 2006, 45, 38–68 (
Angew. Chem., Int. Ed.
, 2006
, 118
, 44–74
) CrossRef CAS PubMed
;
(c) C. Tschierske, Angew. Chem., Int. Ed., 2013, 52, 8828–8878 (
Angew. Chem., Int. Ed.
, 2013
, 125
, 8992–9047
) CrossRef CAS PubMed
.
- S. Herbst, B. Soberats, P. Leowanawat, M. Lehmann and F. Würthner, Angew. Chem., Int. Ed., 2017, 56, 2162–2165 CrossRef CAS PubMed
.
- D. Gao, J. A. Edzang, A. K. Diallo, T. Dutronc, T. S. Balaban, C. Videlot-Ackermann, E. Terazzi and G. Canard, New J. Chem., 2015, 39, 7140–7146 RSC
.
- W. J. Harrison, D. L. Mateer and G. J. T. Tiddy, J. Phys. Chem., 1996, 100, 2310–2321 CrossRef CAS
.
-
(a) D. K. Prusty, M. Kwak, J. Wildeman and A. Herrmann, Angew. Chem., Int. Ed., 2012, 51, 11894–11898 CrossRef CAS PubMed
;
(b) J. A. Hendricks, E. J. Keliher, D. Wan, S. A. Hilderbrand, R. Weissleder and R. Mazitschek, Angew. Chem., Int. Ed., 2012, 51, 4603–4606 CrossRef CAS PubMed
;
(c) O. A. Bozdemir, S. E. Cakmak, O. O. Ekiz, A. Dana and E. U. Akkaya, Angew. Chem., Int. Ed., 2011, 50, 10907–10912 CrossRef PubMed
;
(d) T. Bura, P. Retailleau and R. Ziessel, Angew. Chem., Int. Ed., 2010, 49, 6659–6663 CrossRef CAS PubMed
;
(e) L. Bonardi, H. Kanaan, F. Camerel, P. Jolinat, P. Retailleau and R. Ziessel, Adv. Funct. Mater., 2008, 18, 401–413 CrossRef CAS
.
-
(a) N. Adarsh, M. Shanmugasundaram, R. R. Avirah and D. Ramaiah, Chem. – Eur. J., 2012, 18, 12655–12662 CrossRef CAS PubMed
;
(b) N. Adarsh, R. R. Avirah and D. Ramaiah, Org. Lett., 2010, 12, 5720–5723 CrossRef CAS PubMed
;
(c) N. Adarsh, M. Shanmugasundaram and D. Ramaiah, Anal. Chem., 2013, 85, 10008–10012 CrossRef CAS PubMed
;
(d) S. O. McDonnell and D. F. O’Shea, Org. Lett., 2006, 8, 3493–3496 CrossRef CAS PubMed
;
(e) A. Kamkaew, S. H. Lim, H. B. Lee, L. V. Kiew, L. Y. Chung and K. Burgess, Chem. Soc.
Rev., 2013, 42, 77–88 RSC
.
- S. Mula, S. Frein, V. Russo, G. Ulrich, R. Ziessel, J. Barbera and R. Deschenaux, Chem. Mater., 2015, 27, 2332–2342 CrossRef CAS
.
- A. Florian, M. J. Mayoral, V. Stepanenko and G. Fernández, Chem. – Eur. J., 2012, 18, 14957–14961 CrossRef CAS PubMed
.
- F. Camerel, L. Bonardi, M. Schmutz and R. Ziessel, J. Am. Chem. Soc., 2006, 128, 4548–4549 CrossRef CAS PubMed
.
-
(a) M. Meldal and C. W. Tornøe, Chem. Rev., 2008, 108, 2952–3015 CrossRef CAS PubMed
;
(b) H. C. Kolb and K. B. Sharpless, Drug Discovery Today, 2003, 8, 1128–1137 CrossRef CAS PubMed
.
- Z. C. Zuo, H. B. Liu and Y. L. Li, Chem. – Asian J., 2009, 4, 707–713 CrossRef PubMed
.
- S. Fukuzumi and D. K. P. Ng, Chem. – Eur. J., 2013, 19, 11332–11341 CrossRef PubMed
.
- D. Collado, Y. Vida, Fr. Najera and E. Perez-Inestrosa, RSC Adv., 2014, 4, 2306–2309 RSC
.
- J. Kido, M. Kimura and K. Nagai, Science, 1995, 267, 1332–1334 CrossRef CAS PubMed
.
- M. Chen, Y. Zhao, L. Yan, S. Yang, Y. Zhu, I. Murtaza, G. He, H. Meng and W. Huang, Angew. Chem., Int. Ed., 2017, 56, 722–727 CrossRef CAS PubMed
.
- G. M. Farinola and R. Ragni, Chem. Soc. Rev., 2011, 40, 3467–3482 RSC
.
-
(a) Z. Zhang, D. Liu, D. Li, K. Huang, Y. Zhang, Z. Shi, R. Xie, M.-Y. Han, Y. Wang and W. Yang, Chem. Mater., 2015, 27, 1405–1411 CrossRef CAS
;
(b) X. Li, Y. Liu, X. Song, H. Wang, H. Gu and H. Zeng, Angew. Chem., 2015, 127, 1779–1784 CrossRef
;
(c) E. Aharon, M. Kalina and G. L. Frey, J. Am. Chem. Soc., 2006, 128, 15968–15969 CrossRef CAS PubMed
.
- J. Liu, X. Guo, L. J. Bu, Z. Y. Xie, Y. X. Cheng, Y. H. Geng, L. X. Wang, X. B. Jing and F. S. Wang, Adv. Funct. Mater., 2007, 17, 1917–1925 CrossRef CAS
.
-
(a) A. Dvivedi, S. Kumar and M. Ravikanth, Sens. Actuators, B, 2016, 224, 364–371 CrossRef CAS
;
(b) S. Dini and H. Khanmohammadi, Spectrochim. Acta, 2019, 222, 117157 CrossRef CAS PubMed
.
-
W. L. F. Armarego and C. L. L. Chai, Common physical techniques used in purification, in Purification of laboratory chemicals, ed. W. L. F. Armarego and C. L. L. Chai, Elsevier Academic Press, Cornwall, UK, 5th edn, 2003, pp. 1–52 Search PubMed
.
- Y. Li, B. Li, Y. Fu, S. Lin and Y. Yang, Langmuir, 2013, 29, 9721–9726 CrossRef CAS PubMed
.
- Z. Li, Z. Wu, G. Mo, X. Xing and P. Liu, Instrum. Sci. Technol., 2014, 42, 128–141 CrossRef CAS
.
-
CAChe 3.2, Oxford Molecular Ltd, Oxford, UK, 1999 Search PubMed
.
- F. M. Menger and K. L. Caran, J. Am. Chem. Soc., 2000, 122, 11679–11691 CrossRef CAS
.
- E. E. Jelley, Nature, 1936, 138, 1009–1010 CrossRef CAS
.
- G. Scheibe, Angew. Chem., 1936, 49, 563 CAS
.
- M. Wang, G. L. Silva and B. A. Armitage, J. Am. Chem. Soc., 2000, 122, 9977–9986 CrossRef CAS
.
-
(a) A. Loudet, R. Bandichhor, L. Wu and K. Burgess, Tetrahedron, 2008, 64, 3642–3654 CrossRef CAS PubMed
;
(b) D. O. Frimannsson, M. Grossi, J. Murtagh, F. Paradisi and D. F. O’Shea, J. Med. Chem., 2010, 53, 7337–7343 CrossRef CAS PubMed
.
- I. J. Kim, R. Manivannan and Y. A. Son, Sens. Actuators, B, 2017, 246, 319–326 CrossRef CAS
.
- P.-X. Pei, J.-H. Hu, P.-W. Ni, C. Long, J.-X. Su and Y. Sun, RSC Adv., 2017, 7, 46832–46838 RSC
.
- W.-C. Lin, J.-W. Hu and K.-Y. Chen, Anal. Chim. Acta, 2015, 893, 91–100 CrossRef CAS PubMed
.
- P. S. Kumar, P. R. Lakshmi and K. P. Elango, New J. Chem., 2019, 43, 675–680 RSC
.
- H. f. Gao, H. F. Cheng, Z. H. Yang, M. Prehm, X. H. Cheng and C. Tschierske, J. Mater. Chem. C, 2015, 3, 1301–1308 RSC
.
- L. Ying, C. L. Ho, H. B. Wu, Y. Cao and W. Y. Wong, Adv. Mater., 2014, 26, 2459–2473 CrossRef CAS PubMed
.
Footnotes |
† Electronic supplementary information (ESI) available. See DOI: 10.1039/c9nj04755g |
‡ Both authors contributed equally to this work. |
|
This journal is © The Royal Society of Chemistry and the Centre National de la Recherche Scientifique 2020 |