DOI:
10.1039/D0NH00263A
(Communication)
Nanoscale Horiz., 2020,
5, 1332-1343
Effect of alkaline earth metal chloride additives BCl2 (B = Mg, Ca, Sr and Ba) on the photovoltaic performance of FAPbI3 based perovskite solar cells†
Received
5th May 2020
, Accepted 23rd July 2020
First published on 23rd July 2020
Abstract
Additive engineering is known to be an effective method for inducing a simultaneous effect of enlarging the grain size and surface passivation. As compared to the monovalent halides frequently used as additives, divalent halides are relatively less investigated in the role of additives. In this work, we report effects of alkaline earth metal halides BCl2 (B = Mg, Ca, Sr, Ba) as additives on the opto-electronic properties and photovoltaic performance of FAPbI3 based perovskite solar cells (PSCs). A significant improvement in power conversion efficiency (PCE) from 17.27% to 21.11% is observed by MgCl2 addition in the FAPbI3 precursor solution, while a marginal increment for CaCl2 or BaCl2 and a negative effect for SrCl2 is observed. The lattice constant of cubic FAPbI3 is hardly changed by additives, while the crystallinity is improved by MgCl2. The carrier lifetime increases from 40 ns to 287 ns and the trap density is reduced from 1.08 × 1016 cm−3 to 3.19 × 1015 cm−3 by addition of 5 mol% MgCl2, which is responsible for the enhancement in photovoltaic parameters. The steady-state PCE of the PSC with the MgCl2-additive-treated FAPbI3 measured under continuous illumination at the maximum power point remains unchanged for 1500 s.
New concepts
Formamidinium lead iodide (FAPbI3) perovskite is known to be an ideal material among the studied compositions in terms of optical bandgap. The precursor solution is suggested to include an additive – normally, the monovalent cationic chlorides, such as MACl or CsCl, are frequently used as additives – to improve the photovoltaic performance of FAPbI3 perovskite solar cells (PSCs). However, a change in bandgap is expected because MA or Cs cations can replace the FA cations. Thus, it is required to keep the bandgap of FAPbI3 even after modification by additive engineering. As compared to the monovalent halides, no systematic investigation on the role of divalent halides has been reported in FAPbI3 based PSCs. In this work, we first report the effects of alkaline earth metal halide BCl2 (B = Mg, Ca, Sr, Ba) additives on the opto-electronic properties and photovoltaic performance of FAPbI3 based PSCs. We have found that the addition of MgCl2 improves the power conversion efficiency significantly due to a large increase in carrier lifetime and a significant reduction in trap density. In addition, no change in bandgap is observed because of the higher oxidation state and much smaller ionic radius of Mg2+ than FA and Pb cations.
|
Introduction
Since the report of a 9.7% efficient and 500 h stable solid-state perovskite solar cell (PSC) in 2012,1 developed to solve the instability of methylammonium lead iodide (MAPbI3) in a liquid electrolyte,2,3 a swift surge in perovskite photovoltaics led to a certified power conversion efficiency (PCE) of 25.2% in 2019.4 The inherently excellent opto-electronic properties of organic lead halide perovskites5–7 are responsible for the superb photovoltaic performance, which might be better than the well-known thin film solar cell materials such as Cu(In1−xGax)Se2, multi-crystalline Si and CdTe because of higher PCE. Among the studied compositions for PSCs, FAPbI3 (FA = formamidnium) has been regarded as an ideal perovskite because of suitable bandgap (∼1.47 eV) and no structural phase transition at the operating temperature.8 However, the photoactive FAPbI3 is known to be in the high-temperature stabilized α phase, and tends to undergo phase transition from α to photo-inactive δ phase at ambient temperature.9 It was first reported that a substitution of a certain amount of FA cations with Cs cations can stabilize the α phase at ambient temperature.10 In general, substitution with smaller monovalent cations (Cs+, Rb+ or MA) and/or halide anion (Br−) was found to stabilize the α phase of FAPbI3 thermodynamically.11–14 Thus, these cations can be referred to as “stabilizers” in FAPbI3.12
The stabilizers can be incorporated compositionally by stoichiometrically mixing FA with a certain amount of stabilizer, which is called “compositional engineering”. The incorporation of stabilizer is also possible by “additive engineering” in which an adequate amount of additive is mixed with FAPbI3 precursor. The difference between compositional engineering and additive engineering is that additive engineering is a non stoichiometric approach and elements that may not be involved in the composition can be used as additives. Additive engineering has been found to be also beneficial for both surface passivation and growth of grain size.15 It is well-known that addition of MACl additive in a perovskite precursor solution shows a positive effect on the photovoltaic performance of FAPbI3 based PSCs.16–18 Although it has been argued whether or not chloride is incorporated in iodide sites, it is widely accepted that chloride plays an important role in enlarging the grain size and improving crystallinity.19 Since there is a positive effect of chloride in additives, we have been motivated to systematically investigate the effect of divalent alkaline earth metal chlorides, BCl2 (B = Mg, Ca, Sr and Ba), on the photovoltaic performance and stability of FAPbI3 based PSCs. Since the divalent cations in BCl2 are smaller in ionic radius (Mg2+ = 72 pm, Ca2+ = 100 pm, Sr2+ = 118 pm, and Ba2+ = 135 pm) and have higher formal charge than the FA cation, the surface passivation effect is expected rather than the substitution effect. In addition, the chloride content of BCl2 is twice that of monovalent chloride when the same quantity is dissolved in the precursor solution, which may also affect the opto-electronic properties of the PSCs. To the best of our knowledge, no systematic studies have been reported on the additive engineering of FAPbI3 with BCl2.
Here, we report on the effects of BCl2 additives on the photovoltaic parameters and opto-electronic properties of the FAPbI3 based PSCs. The additive BCl2 is mixed with a pre-synthesized FAPbI3 powder,20 where the concentration of BCl2, [BCl2], is varied with respect to [FAPbI3]. Among the studied additives, MgCl2 is found to improve the photovoltaic performance and stability. Crystal structure, film morphology, band alignment and carrier lifetime are comparatively investigated to understand the basis for the improvement. A pristine FAPbI3 device shows a PCE of 17.27% which is significantly improved to 21.11% by addition of MgCl2 in the FAPbI3 precursor solution.
Results and discussion
Effect of BCl2 (B = Mg, Ca, Sr and Ba) on the opto-electronic properties of FAPbI3 and photovoltaic performance
The perovskite precursor solutions are prepared by mixing the pre-synthesized FAPbI3 powder (yellow δ phase) with BCl2 with different molar ratios (see Experimental details). In order to investigate how the metal precursor additives change the reactivity of the perovskite precursors, UV-vis absorption spectroscopy of the precursor solution is studied. Fig. S1 (ESI†) shows that the absorption band around 310 nm is due to the iodoplumbate (probably PbI2(DMF)x(DMSO)4−x species), where peak intensity is higher for the MgCl2 additive than for other additives. A strong absorption is indicative of an increase in iodoplumbate concentration and thereby an improvement of photovoltaic performance.21 Another weak absorption peak at around 380 nm (inset in Fig. S1, ESI†) is related to PbI3−.22 This peak is also relatively intense for the MgCl2 additive compared to other additives. When considering that two characteristic peaks in the precursor solution affect the photovoltaic performance, MgCl2 additive is expected to be better in performance than other additives, which will be further evaluated. Fig. 1 shows a schematic illustration of perovskite deposition, where the precursor solution with and without additive is spin-coated on a SnO2 coated FTO (fluorine-doped tin oxide) substrate. To control the crystal growth, an intermediate is formed by dripping diethyl ether during the spin-coating procedure.23 The thickness of the 150 °C-annealed perovskite film is about 440 nm as confirmed from cross-sectional scanning electron microscopy (SEM) (images not shown).
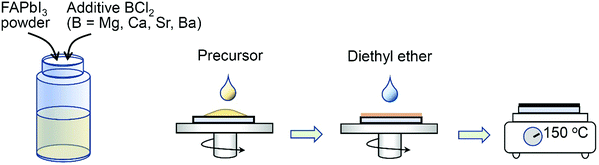 |
| Fig. 1 A schematic illustration of the perovskite deposition procedure. The precursor solution was prepared by mixing the pre-synthesized FAPbI3 powder with BCl2 additive (B = Mg, Ca, Sr and Ba), which was spin-coated. Diethyl ether was dipped for 20 s after rotating the substrate. The film was annealed at 150 °C in an air atmosphere. | |
The effects of the concentration of the additives on the photovoltaic parameters are investigated. As shown in Fig. S2 (ESI†), the photovoltaic parameters are strongly dependent on the additive concentration (note that the final concentration may be different because the solution was filtered prior to use), from which an optimal concentration is determined to be 5 mol% for MgCl2, 1 mol% for CaCl2 and 9 mol% for BaCl2. In contrast to the Mg, Ca and Ba cases, the addition of SrCl2 additive lowers the photovoltaic performance. Despite the negative effect of SrCl2, 10 mol% shows better performance among the tested concentrations from 5 mol% to 15 mol%. Fig. 2 shows the statistical short-circuit photocurrent density (Jsc), open-circuit voltage (Voc), fill factor (FF) and power conversion efficiency (PCE) for the optimal concentration of BCl2 additives. Unlike the BI2 additives doped in MAPbI3,24,25 5 mol% MgCl2 improves substantially Voc and FF, while a negligible or slight enhancement is observed for CaCl2 and BaCl2. Mean values are listed in Table 1, where the average PCE estimated from the reverse scanned parameters is improved from 16.39% to 19.66% by MgCl2 addition. A large improvement in Voc and FF implies that recombination might be reduced by the MgCl2 additive. In our study, bulk doping in FAPbI3 with SrCl2 shows a negative effect leading to deterioration of the photovoltaic performance, which is in contrast to the previous work reporting an improved PCE by the 10–30% doping with SrCl2 in MAPbI3 and even the 2% doping with SrI2 in CsPbI3.26,27
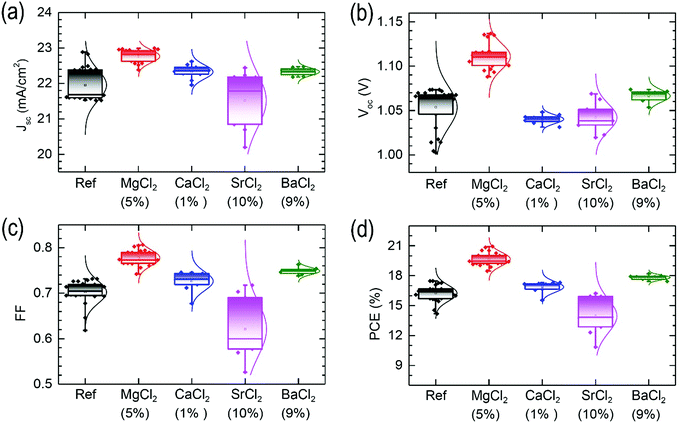 |
| Fig. 2 Statistical photovoltaic parameters of (a) Jsc, (b) Voc, (c) FF and (d) PCE measured at reverse scan for the BCl2 additives (B = Mg, Ca, Sr and Ba). Values in parentheses are the optimal mol% of the additive with respect to FAPbI3. | |
Table 1 Mean photovoltaic parameters of PSCs employing FAPbI3 perovskite films with and without (Ref) BCl2 additives (B = Mg, Ca, Sr and Ba). FS and RS stand for forward scan and reverse scan, respectively
Additive (mol%) |
J
sc (mA cm−2) |
V
oc (V) |
FF |
PCE (%) |
Ref (w/o) |
FS |
21.84 ± 0.42 |
1.07 ± 0.01 |
0.72 ± 0.03 |
16.66 ± 1.06 |
RS |
21.97 ± 0.68 |
1.05 ± 0.03 |
0.71 ± 0.03 |
16.38 ± 1.02 |
MgCl2 (5%) |
FS |
22.72 ± 0.41 |
1.11 ± 0.02 |
0.77 ± 0.02 |
19.36 ± 0.98 |
RS |
22.76 ± 0.31 |
1.11 ± 0.02 |
0.78 ± 0.03 |
19.67 ± 1.23 |
CaCl2 (1%) |
FS |
22.37 ± 0.33 |
1.05 ± 0.01 |
0.72 ± 0.04 |
16.86 ± 0.89 |
RS |
22.11 ± 0.33 |
1.04 ± 0.01 |
0.70 ± 0.03 |
16.09 ± 0.88 |
SrCl2 (10%) |
FS |
21.57 ± 1.12 |
1.04 ± 0.03 |
0.57 ± 0.13 |
12.75 ± 3.70 |
RS |
21.52 ± 1.12 |
1.04 ± 0.02 |
0.62 ± 0.10 |
13.95 ± 2.69 |
BaCl2 (9%) |
FS |
22.21 ± 0.43 |
1.07 ± 0.01 |
0.75 ± 0.01 |
17.66 ± 0.40 |
RS |
22.33 ± 0.15 |
1.07 ± 0.01 |
0.75 ± 0.01 |
17.82 ± 0.39 |
Surface morphology is not significantly altered by MgCl2, while CaCl2, SrCl2 and BaCl2 change the surface morphology of FAPbI3 (Fig. 3(a–e)). Cross-sectional SEM images confirm that MgCl2 results in a pinhole-free interface between SnO2 and perovskite, whereas large pinholes are formed at the interface for the pristine FAPbI3. This indicates that the MgCl2 additive supports an underlying conformal growth of perovskite on the substrate. The MgCl2 additive is expected to contribute to the enlargement of the grain size of FAPbI3 perovskite due to the higher coordination interaction between FA+ and Mg2+ than those of FA+ and Pb2+
28,29 and/or retarded crystallization kinetics.30 The higher Jsc obtained from the MgCl2 additive is thus probably due to better charge collection owing to the pinhole-free interface. In addition, the crystallinity of α phase FAPbI3 is improved in the presence of MgCl2 as confirmed from the X-ray diffraction (XRD) pattern in Fig. 3(f), which might be associated with higher Voc and FF because of the long-range ordering of FAPbI3 and thereby less defects. On the other hand, the addition of CaCl2, SrCl2 and BaCl2 cannot fully convert the δ phase to α phase because the δ phase is still present in the annealed film (Fig. 3(g)). Moreover, the CaCl2 additive increases the unreacted PbI2 peak, implying a strong interaction between CaCl2 and PbI2 in the precursor solution. Although the ionic radius of Sr2+ (118 pm) is similar to that of Pb2+ (119 pm), Sr2+ is not likely to replace Pb2+ because unreacted SrCl2 exists in the annealed film as shown in the XRD peak at around 12.8° in Fig. 3(g) and the lattice constant of cubic FAPbI331 is unchanged by SrCl2 due to no peak shift in the (100) reflection at 2θ = 13.94°.
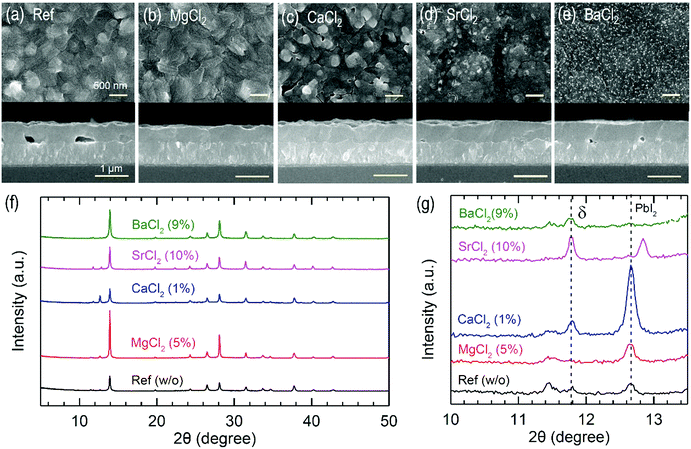 |
| Fig. 3 (a–e) Top-view and cross-sectional scanning electron microscope (SEM) images and (f, g) X-ray diffraction patterns of FAPbI3 films with and without (Ref) additives of MgCl2, CaCl2, SrCl2 and BaCl2. | |
Energy dispersive X-ray spectroscopy (EDS) is carried out to investigate the presence of BCl2 in the annealed FAPbI3 film. For the MgCl2 case in Fig. 4(a), neither Mg nor Cl is detected on the film surface. Similarly, Ca and Cl are not detected on the film surface for FAPbI3 with CaCl2 additive (Fig. 4(b)). However, for the SrCl2 and BaCl2 cases, Sr, Ba and Cl elements are detected with an atomic ratio of Sr
:
Cl or Ba
:
Cl = 1
:
2 (Fig. 4(c) and (d)). No detection of MgCl2 and CaCl2 is probably due to the relatively lower concentration as compared to SrCl2 and BaCl2. The ratio of I to Pb is found to be almost 3 (atomic ratio I
:
Pb = 18.86
:
6.16) for the MgCl2 case, which is indicative of stoichiometric formation of FAPbI3. On the other hand, iodide-rich (or Pb-deficient) phase is formed for CaCl2 and BaCl2 because of the ratio I/Pb = 3.22 (CaCl2) and 3.35 (BaCl2). Addition of SrCl2 results in iodide-deficient (or Pb-rich) phase in the annealed perovskite film. Although excess PbI2 or organic iodide was reported to passivate the perovskite grain boundary and thereby improve the photovoltaic performance,32,33 the stoichiometry of the perovskite was also reported to play an important role in photovoltaic performance and stability because defects can be minimized by stoichiometric composition.34 Thus, stoichiometric FAPbI3 formed by MgCl2 additive is in part responsible for the improved PCE. Since detection of MgCl2 and CaCl2 is unavailable by EDS, we further measure surface-sensitive X-ray photoelectron spectroscopy (XPS) to understand the chemical environment of FAPbI3 films depending on additives.
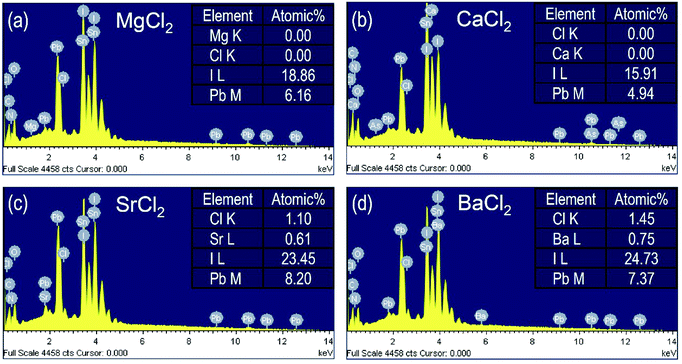 |
| Fig. 4 Energy dispersive X-ray spectroscopy (EDS) of FAPbI3 films with additive of (a) MgCl2 (5%), (b) CaCl2 (1%), (c) SrCl2 (10%) and (d) BaCl2 (9%). | |
Fig. 5(a–d) show Mg 1s, Ca 2p, Sr 3d and Ba 3d XPS spectra before and after addition of the additives. The film samples for XPS measurements were prepared on Si wafers. Mg, Ca, Sr and Ba elements are obviously detected. The detection of Cl from the MgCl2-treated FAPbI3 film confirms the existence of MgCl2 in the film (see Fig. 5(g)). Thus, the additives still exist in the annealed perovskite films. Since addition of MgCl2 significantly improved the photovoltaic performance among the studied BCl2 additives, we further investigate Pb 4f and I 3d XPS for the FAPbI3 films with and without MgCl2. In Fig. 5(e), the pristine FAPbI3 film shows two peaks at binding energies of 143.53 eV and 138.63 eV, which correspond to the Pb 4f5/2 and 4f7/2 core levels, respectively, originated from Pb2+ ions.35,36 The addition of MgCl2 in FAPbI3 shifts the Pb 4f peaks to lower binding energies of 142.93 eV for 4f5/2 and 138.08 eV for 4f7/2. Shift to lower binding energy after adding MgCl2 is also observed from I 3d XPS (Fig. 5(f)), where the 3d3/2 peak at 631.13 eV and the 3d5/2 peak at 619.68 eV shift to 630.63 eV and 619.13 eV. When considering no change in lattice constant by MgCl2, Mg or Cl element might not be directly involved in lattice construction. Thus, the shift in binding energy is indicative of a change in chemical environment around Pb and I at the surface. The decrease in binding energy of Pb2+ and I− is probably related to an increase in electron density around Pb2+ and I−.37–39 In the case that Mg2+ interacts with iodide at the surface, the (Pb–I) bond is more likely to be ionic due to an increased covalency of the adjacent (Mg–I) bond because of the smaller ionic radius of Mg2+ (72 pm) than Pb2+ (119 pm) according to Fajan's rule.40 Thus, the electron density of iodide in the (Mg–I–Pb) interaction is relatively increased as compared to the (Pb–I–Pb) configuration. In addition, the more covalent character of (Mg–I) will donate electrons to Pb, which increases the electron density of Pb. Chloride in MgCl2 can also affect the shift of the Pb 4f and the I 3d peaks by donating lone-pair elections to the perovskite surface. It was reported that post-treatment with SrCl2 was found to shift the Pb 4f and the I 3d core levels to lower binding energy.41
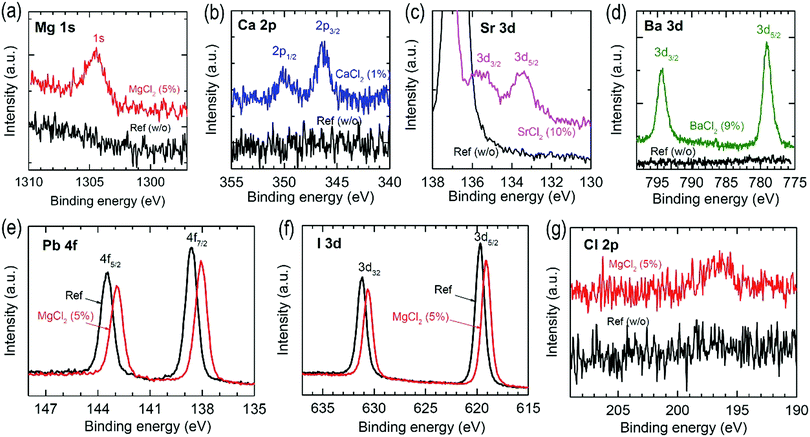 |
| Fig. 5 Mg 1s, Ca 2p, Sr 3d and Ba 3d X-ray photoelectron spectroscopy (XPS) spectra of the annealed FAPbI3 films with and without additives of (a) MgCl2, (b) CaCl2, (c) SrCl2 and (d) BaCl2. (e) Pb 4f, (f) I 3d and (g) Cl 2p XPS spectra of the FAPbI3 films before and after adding the MgCl2 additive. | |
Modification of the optical bandgap and band position is studied using UV-vis and ultraviolet photoelectron spectroscopy (UPS). Fig. 6(a) shows the UV-vis spectra of the annealed FAPbI3 with and without additives. The absorbance is slightly enhanced by the additives, which is attributed to a slight increase in Jsc. Despite better absorbance observed from the SrCl2 case, the lower Jsc than the reference device might be related to the presence of unconverted δ phase and/or poor SnO2/perovskite interface in the aforementioned Fig. 3. The Tauc plot obtained from the UV-vis spectral data is shown in Fig. 6(b), where a linear fit in the 1.54–1.57 eV range of photon energy reveals that the optical bandgap (Eg) is determined to be 1.50 eV for MgCl2 and CaCl2 and 1.51 eV for SrCl2 and BaCl2, which are almost unchanged as compared to the pristine FAPbI3 (Eg = 1.50 eV). Unaltered Eg is consistent with the invariable lattice constant as observed in XRD. Our result is different from the doping effect on Eg of MAPbI3 or CsPbI3, where Eg was influenced by doping due to the substitution effect.42–45 Valence band (EVB) and conduction band (ECB) positions are determined from UPS using He I photon energy (hν = 21.2 eV). In Fig. 6(c), UPS spectra are illustrated, showing the binding energy region of the cut-off energy (Ecutoff) and the valence band maximum (VBM). Work function (WF) is determined from WF = hν − Ecutoff (see Fig. S3 (ESI†) for determining Ecutoff) and EVB is determined using EVB = WF + VBM (see Fig. S3 (ESI†) for determining VBM).46 To determine VBM, we use a logarithmic scale method.47 The estimated values are listed in Table 2 and plotted in Fig. 6(d). EVB is determined to be 6.38 eV for the non-additive pristine FAPbI3, which is consistent with the reported value obtained from the combined experimental and theoretical data.48 Upon addition of additives, WF is almost unchanged, which indicates that the additive might not act as a dopant. Unlike the BI2 additive in MAPbI3 perovskite, resulting in up-shift of EVB and ECB,49EVB is down-shifted by the BCl2 additives in FAPbI3 perovskite films. The chloride seems to play a common effect on down-shifting EVB. Since the antibonding-nature EVB for the pristine FAPbI3 is formed by overlapping the Pb 6s orbital with the I 5p from the viewpoint of molecular orbital theory, the chloride at the surface is likely to lower the EVB because of overlap with a more electronegative Cl 3p orbital.50 It was reported that a low concentration of Mg doping into MAPbI3 led to down-shift of EVB and more n-type property.51 Thus, more n-type FAPbI3 might be induced by addition of MgCl2.
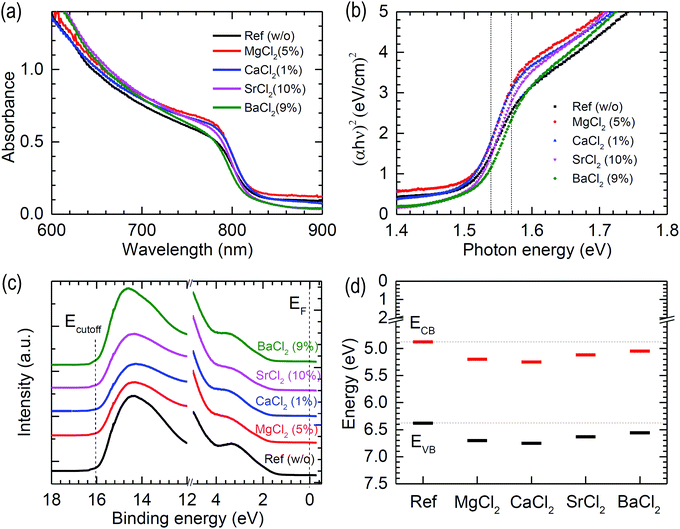 |
| Fig. 6 (a) UV-vis spectra, (b) Tauc plot, (c) ultraviolet photoelectron spectroscopy (UPS) and (d) band (conduction band (CB) and valence band (VB)) positions for the FAPbI3 films with and without additives. UPS spectra were measured with He I photon energy (21.2 eV). | |
Table 2 Work function (WF), valence band energy (EVB) and conduction band energy (ECB) estimated from the cut-off energy (Ecutoff), valence band maximum (VBM) and optical bandgap (Eg)
Sample |
E
cutoff (eV) |
WF (eV) |
VBM (eV) |
E
VB (eV) |
E
g (eV) |
E
CB (eV) |
Ref (w/o) |
16.05 |
5.15 |
1.23 |
6.38 |
1.50 |
4.88 |
MgCl2 (5%) |
16.05 |
5.15 |
1.55 |
6.70 |
1.50 |
5.20 |
CaCl2 (1%) |
16.00 |
5.20 |
1.55 |
6.75 |
1.50 |
5.25 |
SrCl2 (10%) |
16.02 |
5.18 |
1.45 |
6.63 |
1.51 |
5.12 |
BaCl2 (9%) |
16.11 |
5.09 |
1.47 |
6.56 |
1.51 |
5.05 |
Effect of MgCl2 additive on carrier mobility and trap density of FAPbI3
The improved Voc by MgCl2 additive in spite of the unchanged Eg underlines that carrier lifetime, associated with trap density, is probably improved. To verify this hypothesis, we measure time-resolved photoluminescence (TRPL) along with steady-state PL. In Fig. 7(a), the PL intensity of the bulk perovskite film is increased by MgCl2, which is indicative of reduction of non-radiative recombination. Upon contacting the perovskite film with SnO2, the PL intensity is similarly decreased by 90.4% (pristine) and 89.5% (MgCl2). Despite a similar degree of PL quenching, a lower Jsc for the pristine FAPbI3 is due to the presence of pinholes at the SnO2/perovskite interface (see Fig. 3). The TRPL spectrum in Fig. 7(b) is fit with the bi-exponential decay equation, f(t) = A1
exp(−t/τ1) + A2
exp(−t/τ2), where τ1 and τ2 are time constants and A1 and A2 are amplitude.33Table 3 lists the fit results of pristine perovskite and perovskite film with MgCl2 additive on the glass substrate. The addition of MgCl2 increases τ1 from 1.9 ns to 3.9 ns and τ2 from 201.2 ns to 376.5 ns. In addition, the amplitude ratio (A1/A2) decreases significantly from 447.9 to 30.3 after addition of MgCl2. This decrease in A1/A2 indicates that the contribution of the recombination pathway associated with τ1 decreases or in other words is mostly suppressed by the additive. Since τ1 is the one related to the surface defects, whereas τ2 is linked to bulk traps,52 MgCl2 appears to not only slow down the recombination process but suppress especially the presence of surface traps (i.e. the higher the number of surface traps, the larger the contribution (A1)). As a result, average carrier lifetime (τave) increases significantly from 40.1 ns to 287.6 ns after addition of MgCl2. Trap density (nt) is also evaluated from the space charge-limited current (SCLC) of a device with an electron-only configuration of FTO/SnO2/perovskite/PCBM/Au53 as shown in Fig. 7(c). nt is calculated based on the trap-filled-limit voltage (VTFL) obtained from dark J–V curves in Fig. 7(c).15VTFL is estimated to be 0.702 V for the pristine FAPbI3, which is significantly lowered to 0.186 V after the addition of MgCl2. As a result, nt decreases from 1.08 × 1016 cm−3, which is consistent with the reported value,54 to 3.19 × 1015 cm−3 after the addition of MgCl2. The prolonged τave is thus attributed to the decreased nt. The reverse saturation current density (J0) and the ideality factor (m) are also estimated from the full solar cell device structure in the dark. The dark J–V data shown in Fig. 7(d) result in J0 = 2.64 × 10−4 mA cm−2 for the PSC employing the FAPbI3 without additive, which is more than two orders of magnitude lowered after MgCl2 (J0 = 4.87 × 10−6 mA cm−2). In addition, the addition of MgCl2 lowers m from 1.82 to 1.42. The lower J0 supports that the MgCl2 additive in FAPbI3 decreases shallow trap states. The reduced m is indicative of a suppression of the trap-assisted (mono molecular) recombination by the presence of MgCl2 since the ideality factor correlates with the bimolecular band-to-band recombination (m = 1) and the Shockley–Read–Hall (SRH) recombination (m = 2) in photoelectric devices.55 The change in J0 and m is also responsible for the improved Voc and FF.56,57
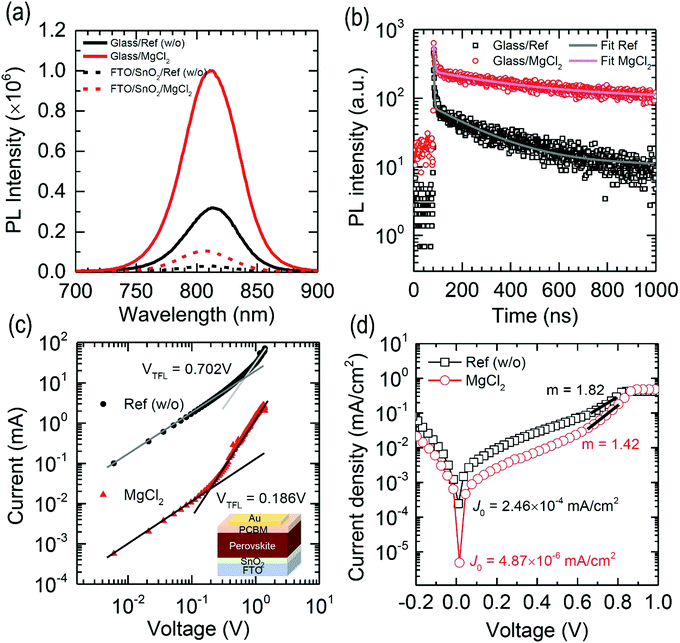 |
| Fig. 7 (a) Steady-state photoluminescence (PL) of the FAPbI3 film with and without MgCl2 deposited on a glass substrate and SnO2-coated FTO substrate. (b) Time-resolved PL (TRPL) for the FAPbI3 film with and without MgCl2 on a glass substrate. Dark current density (J)–voltage (V) curves for (c) the FTO/SnO2/FAPbI3 (with or without MgCl2)/PCBM/Au electron-only device and (d) the full solar cell device. | |
Table 3 Parameters for fitting TRPL data with a bi-exponential decay equation of f(t) = A1
exp(−t/τ1) + A2
exp(−t/τ2). Average lifetime (τave) was calculated by the weighted average of (A1τ12 + A2τ22)/(A1τ1 + A2τ2)
Samples |
A
1
|
τ
1 (ns) |
A
2
|
τ
2 (ns) |
τ
ave (ns) |
Glass/perovskite w/o MgCl2 |
29111.5 |
1.9 |
65.0 |
201.2 |
40.1 |
Glass/perovskite w/MgCl2 |
4545.8 |
3.9 |
150.2 |
376.5 |
287.6 |
Efficiency and stability of PSCs employing the FAPbI3 film with MgCl2 additive
Fig. 8(a) shows the J–V curves for the best performing PSCs employing the FAPbI3 films with and without 5 mol% MgCl2. The relevant photovoltaic parameters obtained from RS and FS are listed in Table 4. The PCE estimated from RS is enhanced from 17.27% to 21.11% after addition of 5 mol% MgCl2 in the FAPbI3 precursor solution due to the increment of Voc from 1.06 V to 1.15 V, FF from 0.73 to 0.79 and Jsc from 22.39 mA cm−2 to 23.33 mA cm−2. The integrated Jscs from the EQE data in Fig. 8(b) show a slight deviation from the measured Jsc values but the same tendency (20.07 mA cm−2 without additive vs. 22.32 mA cm−2 with MgCl2 additive). It is found from the steady-state Jsc and PCE measured at the maximum power point (MPP) that Jsc and PCE remain almost unchanged after 1500 s (Fig. 8(c)). This indicates that the MgCl2 additive improves not only the efficiency but also the stability due to reduced bulk and surface defects simultaneously.
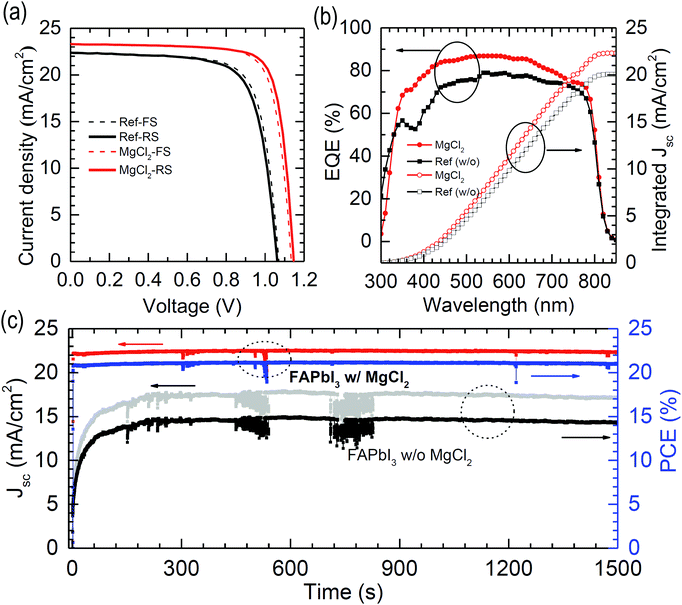 |
| Fig. 8 (a) J–V curves and (b) external quantum efficiency (EQE) of PSCs employing FAPbI3 with and without MgCl2 additive. (c) Steady-state Jsc and PCE for the PSCs employing FAPbI3 with and without the MgCl2 additive, measured at the maximum power point (MPP) for 1500 s under continuous 1 sun illumination. | |
Table 4 Photovoltaic parameters of the best performing PSCs employing FAPbI3 with and without 5 mol% MgCl2 additive. FS and RS stand for forward scan and reverse scan, respectively
|
J
sc (mA cm−2) |
V
oc (V) |
FF |
PCE (%) |
Ref (w/o) |
FS |
22.41 |
1.07 |
0.74 |
17.74 |
RS |
22.39 |
1.06 |
0.73 |
17.27 |
MgCl2 (5%) |
FS |
23.33 |
1.13 |
0.78 |
20.58 |
RS |
23.33 |
1.15 |
0.79 |
21.11 |
Conclusions
We have demonstrated that the addition of MgCl2 in the FAPbI3 precursor solution substantially improved the photovoltaic performance without altering the bandgap and lattice constant. It was also found that the concentration of the additive played an important role in determining the photovoltaic performance. CaCl2 and BaCl2 were found to increase the photovoltaic performance but the increment was marginal. For SrCl2, the addition of SrCl2 showed a negative effect due to poor morphology and incomplete δ → α phase transition. Using a nominal concentration of 5 mol% of MgCl2, a photo-stable and 21.11% efficient PSC was achieved.
Experimental details
Materials
N,N-Dimethylformamide (DMF, 99.8%), dimethyl sulfoxide (DMSO, 99.9%), diethyl ether (99.7%), chlorobenzene (CB, 99.8%), formamidinium acetate (FAAc, 99%), lithium bis(trifluoromethanesulfonyl)imide (LiTFSI, 99.8%), 4-tert-butylpyridine (tBP, 98%), acetonitrile (ACN, 99.999%), hydroiodic acid (HI, 57 wt% in water), magnesium chloride (MgCl2, 99.99%), calcium chloride (CaCl2, 99.9%), strontium chloride (SrCl2, 99.99%) and barium chloride (BaCl2, 99.999%) were purchased from Sigma-Aldrich. Aqueous colloidal tin(IV) oxide solution (SnO2, 15% in H2O) was purchased from Alfa Aesar. 2,2′,7,7′-tetrakis(N,N′-di-p-methoxyphenylamine)-9,9′-spirobifluorene (spiro-MeOTAD) was purchased from ShareChem. Lead(II) iodide (PbI2, 95%) was purchased from Kanto Chemical Co., Inc. All the chemicals were used as-received without further purification. Fluorine doped tin oxide (FTO) glass (Pilkington, TEC-8, 8 Ω sq−1) was used as a transparent electrode.
Synthesis of formamidinium iodide (FAI)
FAI was synthesized by reacting FAAc with HI. HI was slowly dropped into a round-bottom flask filled with FAAc, which was stirred for 2 h at 0 °C. The product was recovered by evaporating the solvent at 60 °C using a rotary evaporator, which was washed with diethyl ether and recrystallized from ethanol. Finally, the white precipitate was dried in a vacuum oven at 65 °C for 24 h and then stored in a glove box filled with argon prior to use.
Synthesis of FAPbI3 powder
FAPbI3 powder was synthesized by reacting FAI (2.236 g) with PbI2 (4.61 g) (molar ratio FAI
:
PbI2 = 1.3
:
1) in 60 ml ACN under stirring for 2 days at room temperature.20 The product was dried in a vacuum oven for 2 days at room-temperature and then stored in a glove box filled with argon.
Device fabrication
FTO-coated glass was cleaned with solvents (water and ethanol), and was further treated with ultraviolet-ozone (UVO) for 40 min. A SnO2 thin layer was prepared by spin-coating the diluted SnO2 solution on the cleaned FTO substrate at 4000 rpm for 20 s and then annealed at 185 °C for 30 min. The SnO2-coated FTO substrate was treated with UVO for 20 min prior to deposition of the perovskite precursor. The perovskite precursor solution was prepared by dissolving the pre-synthesized FAPbI3 powder (concentration was 1.4 M) in a mixed solvent of DMF and DMSO (DMF
:
DMSO = 8
:
1 v/v). To study the effect of the additives, a certain amount of additive (MgCl2 (5%): 0.0033 g, CaCl2 (1%): 0.0008 g, SrCl2 (10%): 0.0111 g, and CaCl2 (9%): 0.0131 g) was mixed with 0.4431 g of FAPbI3 powder in 0.5 ml of DMF/DMSO mixed solvent, which was stirred for over 12 h. It should be noted that MgCl2 itself was dissolved well in the mixed DMF/DMSO solvent but a precipitate was partially formed when it was mixed with FAPbI3. Thus filtration was required especially for the MgCl2 case. For the perovskite deposition, the spin-coating condition was as follows. The precursor solution was filtered and 25 μl of precursor solution was spread on a SnO2/FTO substrate, which was spun at 1000 rpm for 5 s and 5000 rpm for 20 s. Diethyl ether (1 ml) was dripped 20 s after spinning. The as-spun film was annealed at 150 °C for 30 min. A spiro-MeOTAD hole transporting layer was formed on the perovskite film by spin coating 20 μl of the spiro-MeOTAD solution (72.3 mg of spiro-MeOTAD, 28.8 μl of tBP, and 17.5 μl of LiTFSI solution (520 mg LiTSFI in 1 ml ACN in 1 mL of CB)) at 4000 rpm for 20 s. On top of the spiro-MeOTAD layer, a ca. 65 nm-thick Au electrode was formed by a thermal evaporation method at an evaporation rate of 0.3 Å s−1 under ca. 3.8 × 10−7 Torr.
Characterization
Current density–voltage (J–V) curves were obtained by a solar simulator with a 450 W xenon lamp (Newport 6279 NS) and a Keithley 2400 source meter. The AM 1.5G one sun (100 mW cm−2) was adjusted by the NREL-calibrated silicon reference solar cell with a KG-5 filter. The external quantum efficiency (EQE) was measured using an EQE system equipped with a 75 W xenon lamp (USHIO) as a white light source, where the monochromatic beam was generated by chopping the white light. Scanning electron microscopy (SEM) images were observed with field-emission scanning electron microscopy (JSM7000F). UV-vis spectra were recorded using a UV-vis spectrophotometer (Lambda 45, PerkinElmer). Steady-state photoluminescence (PL) and time-resolved photoluminescence (TRPL) spectra were measured using a fluorescence spectrometer (QuantaurusTau C11367-12, Hamamatsu) with excitation of a 464 nm laser (PLP-10, Humamatsu) pulsed at a frequency of 10 MHz for steady-state PL and 200 kHz for TRPL. X-ray diffraction (XRD) patterns were obtained by the diffractometer (Rigaku Smart lab SE) with monochromatic Cu Kα radiation (λ = 1.54056 Å) at a scan rate of 4° min−1. X-ray photoelectron spectroscopy (XPS) and ultraviolet photoelectron spectroscopy (UPS) measurements were carried out on an ESCALAB 250 XPS system (Thermo Fisher Scientific) with Al Kα X-ray radiation (1486.6 eV) for XPS and He I (21.2 eV) for UPS.
Conflicts of interest
The authors declare no conflict of interest.
Acknowledgements
This work was supported by the National Research Foundation of Korea (NRF) grants funded by the Ministry of Science and ICT (MSIT) of Korea under contracts NRF-2012M3A6A7054861 (Global Frontier R&D Program on Center for Multiscale Energy System), NRF-2016M3D1A1027663 and NRF-2016M3D1A1027664 (Future Materials Discovery Program) and NRF-2015M1A2A2053004 (Climate Change Management Program).
Notes and references
- H.-S. Kim, C.-R. Lee, J.-H. Im, K.-B. Lee, T. Moehl, A. Marchioro, S.-J. Moon, R. Humphry-Baker, J.-H. Yum, J. E. Moser, M. Grätzel and N.-G. Park, Lead Iodide Perovskite Sensitized All-Solid-State Submicron Thin Film Mesoscopic Solar Cell with Efficiency Exceeding 9%, Sci. Rep., 2012, 2, 591 CrossRef PubMed.
- A. Kojima, K. Teshima, Y. Shirai and T. Miyasaka, Organometal Halide Perovskites as Visible-Light Sensitizers for Photovoltaic Cells, J. Am. Chem. Soc., 2009, 131, 6050–6051 CrossRef CAS PubMed.
- J.-H. Im, C.-R. Lee, J.-W. Lee, S.-W. Park and N.-G. Park, 6.5% efficient perovskite quantum-dot-sensitized solar cell, Nanoscale, 2011, 3, 4088–4093 RSC.
-
https://www.nrel.gov/pv/cell-efficiency.html, accessed on April 29, 2020.
- W. A. Laban and L. Etgar, Depleted hole conductor-free lead halide iodide heterojunction solar cells, Energy Environ. Sci., 2013, 6, 3249–3253 RSC.
- G. C. Xing, N. Mathews, S. Y. Sun, S. S. Lim, Y. M. Lam, M. Grätzel, S. Mhaisalkar and T. C. Sum, Long-Range Balanced Electron- and Hole-Transport Lengths in Organic-Inorganic CH3NH3PbI3, Science, 2013, 342, 344–347 CrossRef CAS PubMed.
- M. A. Green, A. Ho-Baillie and H. J. Snaith, The emergence of perovskite solar cells, Nat. Photonics, 2014, 8, 506–514 CrossRef CAS.
- J.-W. Lee, D.-J. Seol, A.-N. Cho and N.-G. Park, High-Efficiency Perovskite Solar Cells Based on the Black Polymorph of HC(NH2)2PbI3, Adv. Mater., 2014, 26, 4991–4998 CrossRef CAS PubMed.
- X. J. Zheng, C. C. Wu, S. K. Jha, Z. Li, K. Zhu and S. Priya, Improved Phase Stability of Formamidinium Lead Triiodide Perovskite by Strain Relaxation, ACS Energy Lett., 2016, 1, 1014–1020 CrossRef CAS.
- J.-W. Lee, D.-H. Kim, H.-S. Kim, S.-W. Seo, S. M. Cho and N.-G. Park, Formamidinium and Cesium Hybridization for Photo- and Moisture-Stable Perovskite Solar Cell, Adv. Energy Mater., 2015, 5, 1501310 CrossRef.
- W. S. Yang, J. H. Noh, N. J. Jeon, Y. C. Kim, S. Ryu, J. Seo and S. I. Seok, High-performance photovoltaic perovskite layers fabricated through intramolecular exchange, Science, 2015, 348, 1234–1237 CrossRef CAS PubMed.
- C. Mu, J. L. Pan, S. Q. Feng, Q. Li and D. S. Xu, Quantitative Doping of Chlorine in Formamidinium Lead Trihalide (FAPbI(3−x)Cl(x)) for Planar Heterojunction Perovskite Solar Cells, Adv. Energy Mater., 2017, 7, 1601297 CrossRef.
- J.-W. Lee and N.-G. Park, Chemical Approaches for Stabilizing Perovskite Solar Cells, Adv. Energy Mater., 2020, 10, 1903249 CrossRef CAS.
- N.-G. Park and K. Zhu, Scalable fabrication and coating methods for perovskite solar cells and solar modules, Nat. Rev. Mater., 2020, 5, 333–350 CrossRef CAS.
- S.-G. Kim, J. Chen, J.-Y. Seo, D.-H. Kang and N.-G. Park, Rear-Surface Passivation by Melaminium Iodide Additive for Stable and Hysteresis-less Perovskite Solar Cells, ACS Appl. Mater. Interfaces, 2018, 10, 25372–25383 CrossRef CAS PubMed.
- M. Mateen, Z. Arain, Y. Yang, X. Liu, S. Ma, C. Liu, Y. Ding, X. Ding, M. Cai and S. Dai, MACl-Induced Intermediate Engineering for High-Performance Mixed-Cation Perovskite Solar Cells, ACS Appl. Mater. Interfaces, 2020, 12, 10535–10543 CrossRef PubMed.
- M. Kim, G.-H. Kim, T. K. Lee, I. W. Choi, H. W. Choi, Y. Jo, Y. J. Yoon, J. W. Kim, J. Lee, D. Huh, H. Lee, S. K. Kwak, J. Y. Kim and D. S. Kim, Methylammonium Chloride Induces Intermediate Phase Stabilization for Efficient Perovskite Solar Cells, Joule, 2019, 3, 2179–2192 CrossRef CAS.
- G. Yang, H. Zhang, G. Li and G. Fang, Stabilizer-assisted growth of formamdinium-based perovskites for highly efficient and stable planar solar cells with over 22% efficiency, Nano Energy, 2019, 63, 103835 CrossRef CAS.
- B. Lee, T. Hwang, S. Lee, B. Shin and B. Park, Microstructural Evolution of Hybrid Perovskites Promoted by Chlorine and its Impact on the Performance of Solar Cell, Sci. Rep., 2019, 9, 4803 CrossRef PubMed.
- Y. Zhang, S. Seo, S. Y. Lim, Y. Kim, S.-G. Kim, D.-K. Lee, S.-H. Lee, H. Shin, H. Cheong and N.-G. Park, Achieving Reproducible and High-Efficiency (>21%) Perovskite Solar Cells with a Presynthesized FAPbI3 Powder, ACS Energy Lett., 2020, 5, 360–366 CrossRef CAS.
- G. S. Shin, S.-G. Kim, Y. Zhang and N.-G. Park, A Correlation between Iodoplumbate and Photovoltaic Performance of Perovskite Solar Cells Observed by Precursor Solution Aging, Small Methods, 2020, 4, 1900398 CrossRef.
- Y. Deng, C. H. V. Brackle, X. Dai, J. Zhao, B. Chen and J. Huang, Tailoring solvent coordination for high-speed, room-temperature blading of perovskite photovoltaic films, Sci. Adv., 2019, 5, eaax7537 CrossRef CAS PubMed.
- N. Ahn, D.-Y. Son, I.-H. Jang, S. M. Kang, M. Choi and N.-G. Park, Highly Reproducible Perovskite Solar Cells with Average Efficiency of 18.3% and Best Efficiency of 19.7% Fabricated via Lewis Base Adduct of Lead(II) Iodide, J. Am. Chem. Soc., 2015, 137, 8696–8699 CrossRef CAS PubMed.
- S.-H. Chan, M.-C. Wu, K.-M. Lee, W.-C. Chen, T.-H. Lina and W.-F. Su, Enhancing perovskite solar cell performance and stability by doping barium in methylammonium lead halide, J. Mater. Chem. A, 2017, 5, 18044–18052 RSC.
- M.-C. Wu, T.-H. Lin, S.-H. Chan, Y.-H. Liao and Y.-H. Chang, Enhanced Photovoltaic Performance of Perovskite Solar Cells by Tuning Alkaline Earth Metal-Doped Perovskite-Structured Absorber and Metal-Doped TiO2 Hole Blocking Layer, ACS Appl. Energy Mater., 2018, 1, 4849–4859 CrossRef CAS.
- H. Zhang, H. Wang, S. T. Williams, D. Xiong, W. Zhang, C.-C. Chueh, W. Chen and A. K.-Y. Jen, SrCl2 Derived Perovskite Facilitating a High Efficiency of 16% in Hole-Conductor-Free Fully Printable Mesoscopic Perovskite Solar Cells, Adv. Mater., 2017, 29, 1606608 CrossRef PubMed.
- C. F. J. Lau, M. Zhang, X. Deng, J. Zheng, J. Bing, Q. Ma, J. Kim, L. Hu, M. A. Green, S. Huang and A. Ho-Baillie, Strontium-Doped Low-Temperature-Processed CsPbI2Br Perovskite Solar Cells, ACS Energy Lett., 2017, 2, 2319–2325 CrossRef CAS.
- F. Yang, M. A. Kamarudin, G. Kapil, D. Hirotani, P. Zhang, C. H. Ng, T. Ma and S. Hayase, Magnesium-Doped MAPbI3 Perovskite Layers for Enhanced Photovoltaic Performance in Humid Air Atmosphere, ACS Appl. Mater. Interfaces, 2018, 10, 24543–24548 CrossRef CAS PubMed.
- R. Chen, D. Hou, C. Lu, J. Zhang, P. Liu, H. Tian, Z. Zeng, Q. Xiong, Z. Hu, Y. Zhu and L. Han, Zinc ion as Effective Film Morphology Controller in Perovskite Solar Cells, Sustainable Energy Fuels, 2018, 2, 1093–1100 RSC.
- N. Guijarro, M. S. Prévot, X. Yu, X. A. Jeanbourquin, P. Bornoz, W. Bourée, M. Johnson, F.
L. Formal and K. Sivula, A Bottom-Up Approach toward All-Solution-Processed High-Efficiency Cu(In,Ga)S2 Photocathodes for Solar Water Splitting, Adv. Energy Mater., 2016, 6, 1501949 CrossRef.
- G. S. Shin, Y. Zhang and N.-G. Park, Stability of Precursor Solution for Perovskite Solar Cell: Mixture (FAI + PbI2) versus Synthetic FAPbI3 Crystal, ACS Appl. Mater. Interfaces, 2020, 12, 15167–15174 CrossRef CAS PubMed.
- H. Zhou, Q. Chen, G. Li, S. Luo, T.-B. Song, H.-S. Duan, Z. Hong, J. You, Y. Liu and Y. Yang, Interface engineering of highly efficient perovskite solar cells, Science, 2014, 345, 542–546 CrossRef CAS PubMed.
- D.-Y. Son, J.-W. Lee, Y. J. Choi, I.-H. Jang, S. Lee, P. J. Yoo, H. Shin, N. Ahn, M. Choi, D. Kim and N.-G. Park, Self-formed grain boundary healing layer for highly efficient CH3NH3PbI3 perovskite solar cells, Nat. Energy, 2016, 1, 1–8 Search PubMed.
- L. K. Ono, S. (Frank) Liu and Y. Qi, Reducing Detrimental Defects for High-Performance Metal Halide Perovskite Solar Cells, Angew. Chem. Int. Ed., 2020, 59, 6676–6698 CrossRef CAS PubMed.
- J. Chen, J.-Y. Seo and N.-G. Park, Simultaneous Improvement of Photovoltaic Performance and Stability by In Situ Formation of 2D Perovskite at (FAPbI3)0.88(CsPbBr3)0.12/CuSCN Interface, Adv. Energy Mater., 2018, 8, 1702714 CrossRef.
- M. Kot, M. Vorokhta, Z. Wang, H. J. Snaith, D. Schmeißer and J. I. Fleg, Thermal stability of CH3NH3PbIxCl3-x versus [HC(NH2)2]0.83Cs0.17PbI2.7Br0.3 perovskite films by X-ray photoelectron spectroscopy, Appl. Surf. Sci., 2020, 513, 145596 CrossRef CAS.
- J. Chen, S.-G. Kim, X. Ren, H. S. Jung and N.-G. Park, Effect of bidentate and tridentate additives on the photovoltaic performance and stability of perovskite solar cells, J. Mater. Chem. A, 2019, 7, 4977–4987 RSC.
- H.-L. Hsu, H.-T. Hsiao, T.-Y. Juang, B.-H. Jiang, S.-C. Chen, R.-J. Jeng and C.-P. Chen, Perovskite Solar Cells: Carbon Nanodot Additives Realize High-Performance Air-Stable p–i–n Perovskite Solar Cells Providing Efficiencies of up to 20.2%, Adv. Energy Mater., 2018, 8, 1802323 CrossRef.
- J. Li, T. Jiu, S. Chen, L. Liu, Q. Yao, F. Bi, C. Zhao, Z. Wang, M. Zhao and G. Zhang, Graphdiyne as a Host Active Material for Perovskite Solar Cell Application, Nano Lett., 2018, 18, 6941–6947 CrossRef CAS PubMed.
- K. Fajans, Struktur und Deformation der Elektronenhüllen in ihrer Bedeutung für die chemischen und optischen Eigenschaften anorganischer Verbindungen, Naturwissenschaften, 1923, 11, 165–172 CrossRef CAS.
- S. Wang, H. Cao, X. Liu, Y. Liu, T. Tao, J. Sun and M. Zhang, Strontium Chloride Passivated Perovskite Thin Films for Efficient Solar Cells with Power Conversion Efficiency over 21% and Superior Stability, ACS Appl. Mater. Interfaces, 2020, 12, 3661–3669 CrossRef CAS PubMed.
- M. Pazoki, T. J. Jacobsson, A. Hagfeldt, G. Boschloo and T. Edvinsson, Effect of metal cation replacement on the electronic structure of metalorganic halide perovskites: Replacement of lead with alkaline-earth metals, Phys. Rev. B, 2016, 93, 144105 CrossRef.
- J. Navas, A. Sánchez-Coronilla, J. J. Gallardo, N. C. Hernández, J. C. Piñero, R. Alcántara, C. Fernández-Lorenzo, D. M. D. l. Santos, T. Aguilar and J. Martín-Calleja, New insights into organic–inorganic hybrid perovskite CH3NH3PbI3 nanoparticles. An experimental and theoretical study of doping in Pb2+ sites with Sn2+, Sr2+, Cd2+ and Ca2+, Nanoscale, 2015, 7, 6216–6229 RSC.
- T. J. Jacobsson, M. Pazoki, A. Hagfeldt and T. Edvinsson, Goldschmidt's Rules and Strontium Replacement in Lead Halogen Perovskite Solar Cells: Theory and Preliminary Experiments on CH3NH3SrI3, J. Phys. Chem. C, 2015, 119, 25673–25683 CrossRef CAS.
- C. F. J. Lau, X. Deng, J. Zheng, J. Kim, Z. Zhang, M. Zhang, J. Bing, B. Wilkinson, L. Hu, R. Patterson, S. Huanga and A. Ho-Baillie, Enhanced performance via partial lead replacement with calcium for a CsPbI3 perovskite solar cell exceeding 13% power conversion efficiency, J. Mater. Chem. A, 2018, 6, 5580–5586 RSC.
- B. Philippe, T. J. Jacobsson, J.-P. Correa-Baena, N. K. Jena, A. Banerjee, S. Chakraborty, U. B. Cappel, R. Ahuja, A. Hagfeldt, M. Odelius and H. Rensmo, Valence Level Character in a Mixed Perovskite Material and Determination of the Valence Band Maximum from Photoelectron Spectroscopy: Variation with Photon Energy, J. Phys. Chem. C, 2017, 121, 26655–26666 CrossRef CAS.
- J. Endres, D. A. Egger, M. Kulbak, R. A. Kerner, L. Zhao, S. H. Silver, G. Hodes, B. P. Rand, D. Cahen, L. Kronik and A. Kahn, Valence and Conduction Band Densities of States of Metal Halide Perovskites: A Combined Experimental-Theoretical Study, J. Phys. Chem. Lett., 2016, 7, 2722–2729 CrossRef CAS PubMed.
- S. Tao, I. Schmidt, G. Brocks, J. Jiang, I. Tranca, K. Meerholz and S. Olthof, Absolute energy level positions in tinand lead-based halide perovskites, Nat. Commun., 2019, 10, 1–10 CrossRef CAS PubMed.
- C. Lu, J. Zhang, D. Hou, X. Gan, H. Sun, Z. Zeng, R. Chen, H. Tian, Q. Xiong, Y. Zhang, Y. Li and Y. Zhu, Calcium doped MAPbI3 with better energy state alignment in perovskite solar cells, Appl. Phys. Lett., 2018, 112, 193901 CrossRef.
- W.-J. Yin, H. Chen, T. Shi, S.-H. Wei and Y. Yan, Origin of High Electronic Quality in Structurally Disordered CH3NH3PbI3 and the Passivation Effect of Cl and O at Grain Boundaries, Adv. Electron. Mater., 2015, 1, 1500044 CrossRef.
- N. Phung, R. Félix, D. Meggiolaro, A. Al-Ashouri, G. S. E. Silva, C. Hartmann, J. Hidalgo, H. Köbler, E. Mosconi, B. Lai, R. Gunder, M. Li, K.-L. Wang, Z.-K. Wang, K. Nie, E. Handick, R. G. Wilks, J. A. Marquez, B. Rech, T. Unold, J.-P. Correa-Baena, S. Albrecht, F. D. Angelis, M. Bär and A. Abate, The Doping Mechanism of Halide Perovskite Unveiled by Alkaline Earth Metals, J. Am. Chem. Soc., 2020, 142, 2364–2374 CrossRef CAS PubMed.
- Y. Zhang, S.-G. Kim, D. Lee, H. Shin and N.-G. Park, Bifacial stamping for high efficiency perovskite solar cells, Energy Environ. Sci., 2019, 12, 308–321 RSC.
- D. Yang, X. Zhou, R. Yang, Z. Yang, W. Yu, X. Wang, C. Li, S. (Frank) Liu and R. P. H. Chang, Surface optimization to eliminate hysteresis for record efficiency planar perovskite solar cells, Energy Environ. Sci., 2016, 9, 3071–3078 RSC.
- D.-Y. Son, S.-G. Kim, J.-Y. Seo, S.-H. Lee, H. Shin, D. Lee and N.-G. Park, Universal approach toward hysteresis-free perovskite solar cell via defect engineering, J. Am. Chem. Soc., 2018, 140, 1358–1364 CrossRef CAS PubMed.
- T. He, S. Li, Y. Jiang, C. Qin, M. Cui, L. Qiao, H. Xu, J. Yang, R. Long, H. Wang and M. Yuan, Reduced-dimensional perovskite photovoltaics with homogeneous energy landscape, Nat. Commun., 2020, 11, 1672 CrossRef CAS PubMed.
- W. Tress, Perovskite Solar Cells on the Way to Their Radiative Efficiency Limit-Insights Into a Success Story of High Open-Circuit Voltage and Low Recombination, Adv. Energy Mater., 2017, 7, 1602358 CrossRef.
- N.-G. Park and H. Segawa, Research Direction toward Theoretical Efficiency in Perovskite Solar Cells, ACS Photonics, 2018, 5, 2970–2977 CrossRef.
Footnote |
† Electronic supplementary information (ESI) available. See DOI: 10.1039/d0nh00263a |
|
This journal is © The Royal Society of Chemistry 2020 |