DOI:
10.1039/D0NH00098A
(Communication)
Nanoscale Horiz., 2020,
5, 934-943
Nanostrip flexible microwave enzymatic biosensor for noninvasive epidermal glucose sensing†
Received
14th February 2020
, Accepted 3rd April 2020
First published on 3rd April 2020
Abstract
Microwave sensors based on microstrip antennas are promising as wearable devices because of their flexibility and wireless communication compatibility. However, their sensitivity is limited due to the reduced sensor size and the potential of biochemical monitoring needs to be explored. In this work, we present a new concept to enhance the microwave signals using nanostrip-based metamaterials. The introduction of the nanostrip structures was achieved by theory and simulations. Experiments prove their enhancement of the electric field and sensing response in the characteristic gigahertz (GHz) wave band. Ordered nanostrips were fabricated on a plastic substrate through a simple nanoscale printing approach. Glucose oxidase is directly doped into the nanostrips, which enables a flexible wearable enzymatic biosensor for glucose sensing. Sensing experiments demonstrated that the nanostrip biosensor gives excellent performance for glucose detection, including high sensitivity, fast response, low detection limit, high affinity, and low power consumption. The applicability of the nanostrip-based sensor as a wearable epidermal device for real-time noninvasive monitoring of glucose in sweat is verified as well.
New concepts
Microwave sensors based on microstrip antennas are good candidates for wearable devices due to their far field wireless communication feature without battery requirements. However, current microstrip technology cannot meet the demand for epidermal testing, which requires higher sensitivity. In this work, for the first time, we proposed and demonstrated nanostrip antenna based microwave sensors. Following the metamaterial design, the ordered nanostrip array, composed of conductive polymers, which are fabricated by a low cost nanoscale printing approach, shows a strong enhancement of microwave signals. We further constructed a novel microwave enzymatic biosensor based on nanostrips doped with glucose oxidase. The proposed sensor shows high performance for glucose sensing with a low detection limit, wide dynamic range, and fast response. By integrating the nanostrips on a flexible substrate, it was demonstrated as a wearable epidermal biosensor for sweat glucose sensing. The proposed new type of nanoscale microwave biosensor could be applied as a general platform for the ultra-sensitive detection of various biomarkers.
|
Introduction
Developing epidermal electronic devices based on skin-friendly soft elastic materials has been of great interest over the past several decades. Due to their body compliance, when attached to the human skin, these flexible devices can be applied as wearable sensors to provide useful human health and medical information on individuals.1,2 Early efforts in developing these flexible and wearable sensors were focused on monitoring body physical signals such as steps,3 pulse waves,4 voice,5 motion,6 breath,7,8 heart rate9 and skin temperature.10 Different types of transducers including force,11 pressure,12,13 temperature,14 and humidity15,16 sensors have been reported. Recently, flexible and wearable biosensors have been proposed as well.17 Compared to physical sensors, these devices require integration of specific bioreceptors into sensing elements which are capable of recognizing target analytes in complex samples at physiologically relevant concentrations.18 Due to their conformal contact with the skin, such flexible epidermal biosensors can achieve in situ analysis of biomarkers in body fluids such as sweat and interstitial fluid. This provides clear advantages compared with conventional blood tests, where invasive blood collection is inevitable. Though many biomarkers could be detected by such wearable biosensors, current research focuses largely on minimally invasive glucose monitoring, which is driven by the huge glucose sensing market for therapeutic diabetes applications. To enable continuous glucose monitoring, entrapment of the enzyme glucose oxidase within a flexible device is generally required. Different types of transducers including electrochemical,19 optical20 and piezo-electrical21 sensing devices have been reported. Though the sensing element of these flexible biosensors has been largely optimized, one of the challenges for real-life application of these wearable epidermal electronics is the need for complex data processing circuits and wireless transmission modules, which are difficult to make flexible. Finding an alternative sensing method which could facilitate data processing and transmission and be compatible with flexible device design may provide a new avenue.
In recent years, microwave biochemical sensors which are based on the change of the electromagnetic properties of the sensing materials at ultrahigh frequencies (ca. >1 GHz) have emerged.22 In the presence of electromagnetic waves, microwave sensors exhibit a strong resonance behaviour with the samples under test.23 The resonant frequency and magnitude will be shifted according to the content and concentration of the sample. Owing to the features of high frequency and passive conduction, microwave sensing technology is very suitable for matching with high frequency wireless transmission circuits, or as a part of antenna systems, so that the required processing and data transmission circuit could be greatly simplified.24,25 In particular, microstrip-type microwave sensors can be designed as planar electrodes, which are compact and mass-produced and can be fabricated on various substrates using the low cost screen printing technique.26
Such sensors have been demonstrated for wireless monitoring of temperature27 and humidity.28 Biochemical sensing using microstrip antennas such as for water–ethanol mixtures,29 milk adulteration,30 cells31 and cancer biomarker32 detection has been reported as well. Very recently, a flexible microwave sensor has been developed to enable conformal contact with the target.33 Mason and Korostynska et al. presented the very first example using a flexible microwave sensor for lactic acid monitoring as a functional wearable system.34 However, the detection limit due to the sensor size reduction hinders the performance of current microstrip based wearable sensing systems. The response signal and trace detection ability of microwave sensors for biochemical indices need to be improved.
According to the electromagnetic enhancement mechanism, roughening of a metal surface can enhance the resonance caused by excitation of electromagnetic waves, which will greatly improve the electric field intensity. A typical representative is a metamaterial, which is defined as an electromagnetic material arranged periodically with its size smaller than the wavelength of the incident electromagnetic wave.35 A metamaterial can manipulate electromagnetic waves by dedicated design of its structure, which has been demonstrated for improvement of the sensing performance of electromagnetic wave sensing.36 Studies on metamaterials have expanded to a fairly broad electromagnetic spectrum,37 including infrared chemical analysis,38 terahertz imaging39 and microwave nondestructive evaluation.40 Inspired by these studies, in this work we proposed a nanostrip type of metamaterial to enhance microwave biosensing based on a sub-100 nm ordered conductive polymer nanowire array (Fig. 1). The design of the nanostrips is analyzed by theory and simulations to enable a strong localization and enhancement of the electromagnetic field. The nanostrips were then fabricated on a flexible plastic substrate by a nanoscale soft printing approach featuring facile fabrication/manufacture with high throughput.41 The GOx enzyme was directly doped into the nanostrips with biotin–streptavidin conjugation to enable specific glucose sensing. Such a flexible epidermal biosensor was tested for glucose detection after attachment to human skin under different bending conditions. Though this work only demonstrates glucose sensing, the proposed new type of nanoscale microwave wearable biosensors can be applied as a general platform for different biomarker detection when integrated with other bio-probes. It has potential application prospects in biomedical testing where a low detection limit is required.42
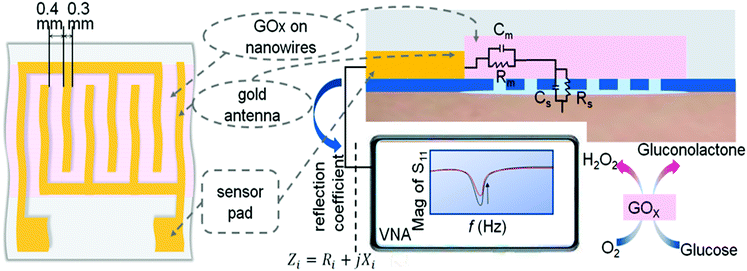 |
| Fig. 1 Schematic illustration of the sensing mechanism and measurement setup. | |
Results and discussion
Mechanism and the enhancement effect of the nanostrip
As shown in Fig. 1, the nanostrips are composed of continuously conductive polymer nanowires, which contacted with the interdigital microwave antenna. The nanostrip integrated microwave sensor is connected to a miniaturized vector network analyzer (VNA) through a subminiature version A interface to extract the microwave spectrum and the reflection coefficient, S11, can be tested in real time.26 The measured value of the input impedance, Zi, at the target frequency is extracted from S11 |  | (1) |
| 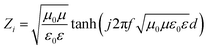 | (2) |
where f and d are the frequency of the microwave and the thickness of the nanostrips, respectively. j represents the imaginary part. μ and ε are the relative complex permeability and the relative complex permittivity of the nanostrips.43 As described in the literature,44 the complex permeability is mainly related to the properties of the material itself, and thus the changes caused by the configuration of the antenna are not significant. However, the equivalent complex dielectric constant could be changed by a different configuration of the antenna, which indicates that the introduction of 1D nanoscale structures may greatly affect the equivalent impedance and the microwave signal.
Next, we discussed the performance of the nanostrip-based sensor, and analyzed the expected enhancement effect of the nanostrips compared with the conventional microstrip sensor by simulations. Fig. 2a and b show the electric field distribution of the nanostrip and microstrip antennas (the local electric field distribution is shown in Fig. S1, ESI†). According to the results, the electric field distribution of the nanostrip antenna is significantly stronger than that of the microstrips, which is ultimately reflected in an increase in the overall electric field strength. This is due to the fact that the nanoscale line width of the nanostrip increases the local current density and ultimately leads to an increase in the electric field strength. This result is consistent with published reports, where they demonstrated improving the electric field strength by using one dimensional nanostructures.45,46 The stronger the electric field, the greater the change in the degree of polarization of the measured object, and thus it is more easily reflected in the change of the microwave signal.
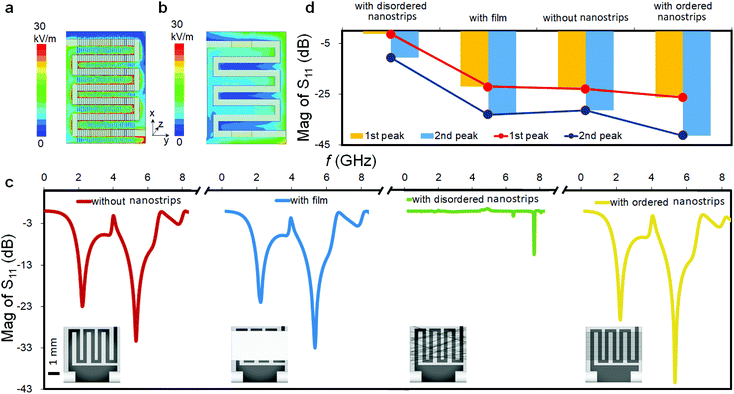 |
| Fig. 2 Electric field distribution of the proposed sensor with microstrips (a) and nanostrips (b) at the characteristic frequency; simulated reflection coefficient of the microwave biosensor without nanostrips, with a thin film, with disordered nanostrips and with ordered nanostrips (c); and corresponding peak value of the S11 magnitude spectrum of the four microwave sensors (d). | |
The spatial distribution of the nanostrips and its effects on the microwave response signal were further analyzed. The reflection coefficient magnitudes of microstrip antennas without nanostrips, with a conductive polymer thin film (same height as the nanostrips), with disordered nanostrips, and with ordered nanostrips were simulated, separately (Fig. 2c). The simulation results show that the four kinds of antennas all have characteristic peaks at 2.2 GHz and 5.3 GHz. The ordered nanostrip antenna obtained the maximum response (Fig. 2d) at the two frequencies. The thin film coated microwave sensor, though having the same height as the nanostrips, has almost no enhancement effect. It is also interesting to note that the reflection coefficient magnitude of the microstrip antennas with the same nanostrips but disordered shows the smallest signal. This indicates that the introduction of nanomaterials needs to be in an orderly arrangement to present the enhancement effect. This result agrees with the published report that the microwave peak will be higher with parallel nanowires due to the array layout shape.44 Such an effect is also consistent with the properties of metamaterials for electromagnetic wave manipulation, where the periodic 1D nano-structure with sub-wavelength size has the strongest enhancement effect on the local electric field.47 For a one-dimensional line structure with a parallel arrangement, κ can be used to represent the resonance in the same direction as the applied electromagnetic wave. It can be expressed as:48,49
|  | (3) |
| 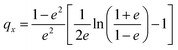 | (4) |
|  | (5) |
|  | (6) |
where
D is the width of the nanowire,
L is the length of the nanowire, and
q and
e are factors which depend on the length of the nanowire. It can be seen from
eqn (3)–(5) that
D and
L are the two key factors; the larger the length/width ratio (
L/
D) of the nanowires, the higher the resonance factor
κx, and it will result in a greater resonance.
κy =
κz corresponding to the resonance in the direction perpendicular to the electromagnetic wave, where it shows little effect on the transmission direction of the microstrip line. In this paper, parallel nanowires are aligned in a large area, with a length larger than 0.4 mm and a linewidth less than 100 nm, and thus
L/
D > 4000. By comparison, the thin film and disordered nanowire structures both have relatively low
L/
D ratios. On account of these reasons, the scale of the nanowires is much smaller than the wavelength and with a high
L/
D ratio; thus, the nanostrip sensor with ordered nanowires exhibits a stronger resonance and yields a higher magnitude response compared to the microstrips without nanowires or with disordered nanowires. These results agree with the simulated electric field (
Fig. 2a and b and Fig. S1, ESI
†), which proves that the introduction of the ordered nanostrip based metamaterial can produce a higher microwave response signal.
To enable specific glucose sensing, the GOx enzyme was immobilized into the nanowires of the proposed microwave sensor. When exposed to a glucose sample, the reaction of the GOx enzyme and glucose produces gluconolactone (lactone of gluconic acid) and hydrogen peroxide (H2O2) according to the following reactions.
| Glucose + GOx(oxd) → Gluconolactone + GOx(red) | (7) |
| GOx(red) + O2 → GOx(oxd) + H2O2 | (8) |
As an enzyme, GOx can be reused as the reaction reaches equilibrium, and the product concentrations change as a result. The incorporation of GOx ensures that any concentration change in the environment is specifically related to the presence and amount of glucose in a given sample. The concentration changes will result in a variation in the dielectric constant, which can be detected by the microwave sensor. The equivalent dielectric constant50 is equal to
|  | (9) |
where
d and
w are the height and width of the substrate.
εm and
εs are the dielectric constants for the substrate and the glucose sample, respectively. Under a microwave field, changes in the dielectric constant of the measured sample are directly related to the changes in the impedance, which can be detected by the microwave sensor.
Fabrication and characterization of the nanostrip microwave biosensor
The fabrication and functionalization of the nanostrip flexible microwave biosensor are shown in Fig. 3. The nanostrips were first patterned by nanoscale soft-printing (NSP) directly on polyethylene terephthalate (PET) substrates. The NSP approach relies on the conformal contact between the soft mode (PDMS) containing nanogroove structures and the bottom substrate. The formed nano-channels ensure a rather large Laplace pressure, which enables the facile filling of the channels with aqueous inks through simple capillary filling. NSP can be applied to many different substrates, featuring simple operation and no need for cleanroom facilities. According to theory analysis, narrower nanowires and a higher density of the nanowires are preferred to achieve the maximum microwave enhancement effect (see the ESI,† S1); we still need to consider the limitation of the materials and fabrication cost of the nanowires. The typical size of the PEDOT:PSS nanoparticle domain is about 60 nm;51 the line width of 100 nm ensures that the PEDOT:PSS can form continuous 1D nanowires without clogging. Regarding the distance between the neighboring nanowires, a higher density of the nanowires will increase the cost of the nanomold. Besides, if the space between the neighboring nanowires is too short, one cannot achieve good conformal contact between the PDMS mold and the substrate, which will result in leaking of the PEDOT:PSS solution during the capillary filling and significantly reduce the quantity of the nanowires. Considering that the intensity of the absorption peak did not change too much in the range of a sub-10 micrometer space between the neighboring nanowires (Fig. S1e and f, ESI†), we used an average 4 μm spacing in this work. Overall, the designed template contains parallel nanogrooves with a cross section of 100 nm (width) × 60 nm (depth) and the spacing between the neighboring nanogrooves is 3 μm and 5 μm. To enable bio-functionalization, here, a mixed conductive polymer aqueous ink is designed by mixing a positively charged polymer (PLL-g-OEG4-biotin) with the negatively charged PEDOT
:
PSS at a typical mixing ratio of 1
:
9.41,52
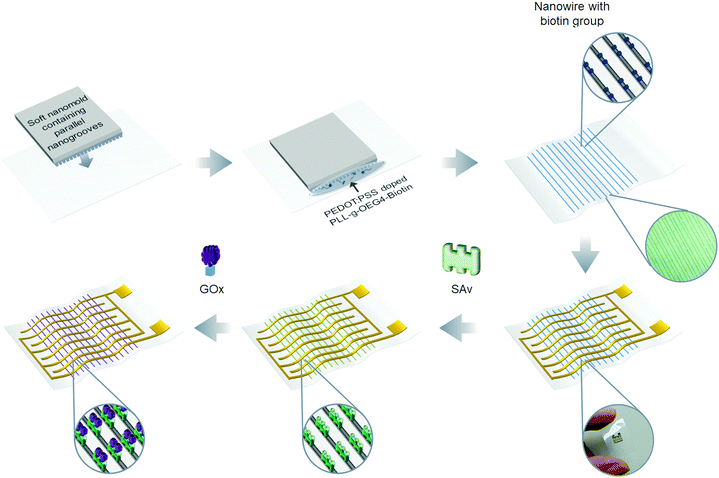 |
| Fig. 3 The schematic of the proposed nanostrip biosensor fabrication and enzyme immobilization. | |
The molecular structure of PEDOT:PSS doped with PLL-g-OEG4-biotin is given in Fig. S2a (ESI†). Such molecular design ensures the good conductivity of the nanowires and direct doping with biotin. After the biotinylated nanowires were structurally patterned, the interdigital antenna structure was deposited graphically with gold evaporation through a shadow mask. Finally, glucose oxidase (GOx) was immobilized on the nanowires through biotin–SAv conjugation. Therefore, the nanoscale glucose microwave biosensor was fabricated, which is based on nanowires linked to the GOx enzyme directly.
Scanning electron microscopy (SEM) (Fig. 4a) and optical microscopy (Fig. S3a, ESI†) images show that the nanowires are collimated and orderly arranged on the transparent PET substrates. The atomic force microscopy (AFM) (Fig. 4b) image shows that the nanowires are firmly adhered to the substrate and aligned parallel with each other with a height of 39 nm, a width of 100 nm and distances between the wires alternating between 3 and 5 μm, which is consistent with the template. After metal evaporation, the nanowires were further checked by laser confocal microscopy and electrical characterization. As shown in Fig. 4c, the nanowires are arranged vertically with the gold antenna, aligned on the flexible substrate uniformly. The IV curve of the conductive nanowires is straight and presents typical ohmic characteristics with a typical resistance around 0.3 MΩ (Fig. S3c, ESI†). Next, we used AFM to follow the enzyme immobilization process on the nanowires. Before the introduction of proteins, the surface of the nanowires is smooth (Fig. 4d). After SAv and enzyme modification, granular protrusions were observed on the nanowires, and the particle height increased after the GOx enzyme immobilization (Fig. 4e and f). The cross section analysis shows that the height of the nanowires is 1.6 nm (original), 10.8 nm (after SAv modification) and 16.1 nm (after GOx immobilization) (Fig. 4g). The height differences coincide with the size of SAv and GOx. Further observation by laser confocal microscopy (Fig. S4a and b, ESI†) shows that the average height of the nanowires before and after the immobilization of GOx by biotin–SAv conjugation was increased by 17.3 nm, which is also consistent with the results of AFM.
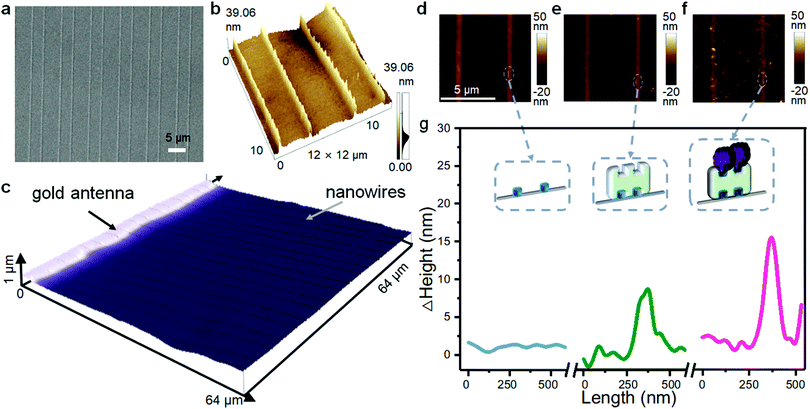 |
| Fig. 4 Surface morphology analysis of the nanowires fabricated on a PET substrate by SEM (a), AFM (b) and confocal microscopy (c); the AFM height images of the original nanowires (d), after SAv modification (e), and after GOx immobilization (f); and the extracted height information on the nanowires before (blue line), after SAv modification (green line), and after GOx immobilization (pink line) (g). | |
The microwave spectra of the nanostrip sensor after SAv and GOx immobilization are shown in Fig. S5 (ESI†). After both SAv and GOx binding the S11 magnitude increased. This is caused by the immobilization of the proteins, which increase the equivalent impedance of the nanowires.
The results of SEM, AFM, and laser confocal microscopy and the microwave spectrum confirm that highly ordered nanowires are patterned on the flexible PET substrate firmly and uniformly. The proteins have been successfully immobilized on the nanowires as well.
Glucose measurements
The proposed nanoscale microwave biosensor was used to detect different concentrations of glucose (Fig. 5a). The sensor was exposed to the sample without glucose and with three different concentrations of glucose of 0.1 nM, 10 nM, and 1 μM in turn. The S11 magnitude response decreased accordingly, and the glucose content in the low concentration range can be clearly distinguished by the proposed sensor. The decrease of the S11 value upon increased glucose concentration is due to the fact that under the catalysis of the GOx enzyme, the content of hydrogen peroxide in the solution increases, thus increasing the dielectric constant of the solution and decreasing the microwave peak. The above results also show that the characteristic glucose sensing frequency range is 0.9–2.0 GHz, and the peak value of the S11 magnitude is collected in real time in this frequency range. Fig. 5b shows the time dependent glucose measurement, where the response value changes regularly with increasing concentration from 0.1 nM to 10 mM glucose solutions.
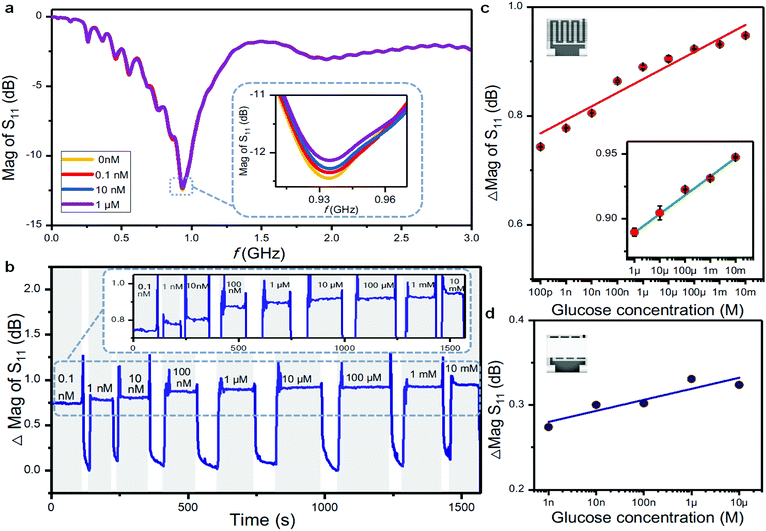 |
| Fig. 5 Reflection coefficient spectra of the proposed nanostrip sensor before and after contact with four different concentrations of glucose (a); real-time glucose detection from 0.1 nM to 10 mM (b); relationship between the response of the nanostrip-based microwave biosensor and the glucose concentration (c); and relationship between the response of the film-based microwave biosensor and the glucose concentration (d). | |
The ability of the sensor to return to the baseline was also tested. Based on five measurements after the removal of each glucose solution, the baseline is −13.1 dB with a standard deviation of 0.18%. There was very little difference in the measured values after removing the solution, indicating that the sensor has a stable baseline and small drift, which are essential for real-time sweat monitoring. We further tested the long-term stability of the sensor by measuring glucose concentrations of 0.1 nM, 10 nM, and 10 μM for 10, 50, and 100 min, respectively, and the differences are 0.25%, 0.67% and 0.43%. The experimental results show that the sensor can function stably for long periods in the presence of a glucose solution with a small drift (Fig. S6, ESI†).
We then characterized the dynamic response of the biosensor. After the addition of a glucose solution, the response magnitude of the biosensor increases rapidly with a fast response, which indicates that the operating speed of the gigahertz band is quick enough to meet the requirement of monitoring a chemical reaction.53,54 Typical Michaelis–Menten kinetics were observed as characterized by a gradual increase in the response loss up to the concentration of saturation (Fig. S7, ESI†).55 The Michaelis constant km is calculated as 4.6 × 10−2 μM, which is lower than that of the glucose sensor based on the optical fiber method (5.99 × 10−2 μM),56 microelectrode method (0.28 mM),57 and gel film method (30 mM).58 A lower value of km is deemed to correspond to higher catalytic activity of the immobilized enzyme.55 The results show that the proposed nanostrip biosensor doped directly with the GOx enzyme possesses high affinity for glucose. Referring to the reaction–diffusion theory, the geometry of the nanostrips ensures a higher diffusion rate than that of planar microstrips,59 and thus facilitates the contact between the immobilized enzyme and the glucose in solution. Besides, the uniform distribution of GOx doped on highly ordered nanowires, nano-scale linewidth and micro-size spacing provide a large reaction area with low steric hindrance, resulting in a high catalytic affinity.
Fig. 5c plots the S11 magnitude changes with the logarithm of the glucose concentration, which clearly presents a linear response from 0.1 nM to 10 mM with a sensitivity of 0.026 dB/log (nM). Within a glucose concentration range of 1 μM to 10 mM, the response linearity reached 0.992. We also compared the sensor response with the thin film-based microwave biosensor at the same glucose concentration (Fig. 5d). The polymer thin film was prepared by spin coating the same polymer ink composed of PEDOT:PSS and PLL–biotin, which has a typical thickness of ∼100 nm. In principle, the thin-film based microwave biosensor contains more GOx compared with the nanostrip sensor. However, the calculated sensitivity of the thin film-based microwave biosensor (0.013 dB/log (nM)) is only half of the nanostrip sensor. Besides, the nanostrip microwave sensor has better linearity and a larger dynamic working range.
Fig. S8 (ESI†) shows the reflection coefficient spectrum of the film-based microwave sensor, with a peak lower than that based on nanostrips. These results confirm that the introduction of the nanostrips, as a periodic nanostructured metamaterial, effectively enhances the resolution of the measured samples in the microwave frequency band.
To test the nanostrip sensor response in a real complex sample, we prepared a simulated sweat solution (0.1% sodium dihydrogen phosphate, 0.5% sodium chloride, 0.5% lactic acid, 0.1% urea, and different concentrations of glucose in ultrapure water, pH adjusted to 5.5 with NaOH60). Two glucose concentrations of 10 nM and 1 μM were measured with the nanostrip sensor. The concentrations measured by the sensor were 9.5 nM and 1.012 μM, respectively, representing accuracies of 95.3% and 101.2%. Then, the sensor was placed in several possible interfering substances for interference testing. As shown in Fig. S9 (ESI†), the response of the sensor to glucose is much higher than the others. Table S1 (ESI†) compares the performance of the nanostrip sensor with previously reported glucose measurements with other types of sensors. The nanoscale microwave biosensor reported herein exhibits a wider test range, shorter response time, and lower detection limit, reflecting the superiority of the sensor performance.
Epidermal sensing
One of the objects in developing flexible biosensors is for epidermal sensing applications, where the flexible substrate ensures conformal contact to the skin. We then tested the device bending effect on its sensing performance.
The nanostrip sensor was bent to 36° to simulate the curvature arising from attachment to the human body, then placed in 0.1 nM, 10 nM, and 1 μM glucose solutions and tested in turn. As shown in Fig. S10 (ESI†), the microwave spectrum of the bent sensor has a slightly positive frequency shift and a decrease in response value compared with the flat sensor. However, its response still effectively distinguishes trace concentrations of glucose, and it is consistent with the response trend of the flat sensor. As physical disturbance can induce changes in the magnitude and frequency of the microwaves, the proposed sensor is more suitable for attaching to relatively static parts of the body, such as the backside,61 where a complex bending or twisting situation is not likely to happen. In the following study for practical application, the interference caused by slight mechanical disturbance should be considered, for example, by introducing a bending correction algorithm.62 Under a fixed curvature, the sensor shows rather good performance for glucose sensing with high linearity (Fig. S11, ESI†) and the detection limit (LOD) is calculated as 0.1 nM. Currently, blood glucose measurement is still the gold standard for glucose monitoring. Some recent studies have proved that sweat glucose measurement is a compromise method which is non invasive.63 However, due to the fluctuation of the glucose level in real sweat, here we chose a simulated sweat sample containing spiked glucose as a test sample to prove the feasibility of the proposed nanostrip sensor for wearable glucose measurement.60,64 A porous polydimethylsiloxane (PDMS) film with a typical thickness of 281.8 μm and hole diameter of 200 μm was prepared to encapsulate the sensor (Fig. 6a). The porous thin film acts as a passivation layer to prevent signal interference,65 and provides a micro chamber for the catalytic reaction (Fig. S12, ESI†). The packed flexible epidermal glucose biosensor was then attached to the human skin, and the microwave spectrum of the sensor was monitored in real time using a miniaturized VNA with a typical size of 9 × 8.3 × 1.4 cm3 and a tablet (Fig. S12d, ESI†). In principle, a wireless signal-reading circuit and miniaturized VNA chip66 could be developed as an alternative to further miniaturize the detection system.
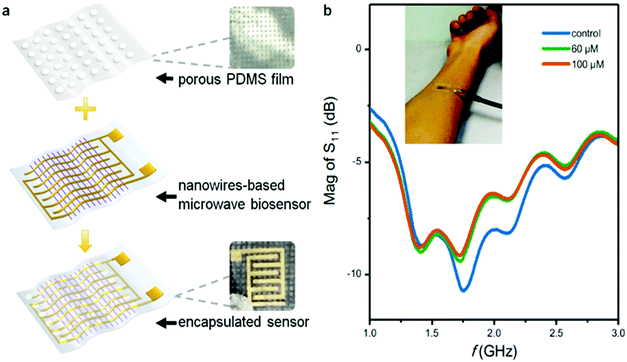 |
| Fig. 6 Packaging process with a PDMS porous membrane (a); and microwave spectrum from a sensor attached to the skin (b). | |
As shown in Fig. 6b, after the sensor was attached to the skin, obvious resonance peaks can be observed in the frequency range of 1.0–2.0 GHz. Two concentrations of simulated sweat (60 μM and 100 μM67) were used, representing the glucose levels of sweat before and after a meal, respectively. After the samples were added between the skin and the sensor patch, the peak value of the sensor immediately decreased with the increase of the glucose concentration. This result proves that the sensor can distinguish the glucose concentration from simulated sweat when attached to human skin. It is also noted that the peak value of the microwave signal is slightly weakened after contact with the skin, which is likely due to the attenuation of the electromagnetic wave in the lossy tissue. Though the sensor can still respond well to the glucose concentration change, a ground plane can be integrated in the sensor to prevent absorption.
Conclusions
This paper reported a flexible epidermal microwave biosensor integrated with nanostrip structures. The ordered nanostrips fabricated by a nanoscale printing approach strongly enhanced the microwave signals, which is proved by simulation and experiments. The GOx enzyme were further integrated into the nanostrips for specific glucose sensing. The sensing results prove that the nanostrip structures not only enhance the electric field of the microwaves but also ensure the high performance of glucose sensing, such as high sensitivity, fast response, high catalytic affinity, and low power consumption, which is due to the high surface-to-volume ratio and extremely small configuration of the nanostrips. The nanostrip-based microwave sensors are further prepared as an epidermal glucose biosensor, which is capable of conformal contact with the skin and non-invasive real-time monitoring of sweat glucose.
Experimental
Materials and reagents
Polydimethylsiloxane (PDMS) was prepared by SYLGARD 184 SILICONE ELASTOMER. Poly-L-lysine hydrobromide (PLL, MW 1/4 15–30 kDa), conducting polymer poly(3,4-ethylenedioxythiophene):poly(styrenesulfonate) (PEDOT:PSS), lactic acid, urea, and phosphate buffered saline (PBS, pH 7.4) tablets were purchased from Sigma Aldrich. Streptavidin (SAv) was purchased from Aladdin Industrial Corporation (Shanghai, China). Sodium dihydrogen phosphate and sodium chloride were purchased from Tianjin Real & Lead Chemical Corporation (Tianjin, China). Biotinylated GOx was purchased from Tianjin Xiensi Corporation (Tianjin, China). All chemicals and solvents used were of analytical reagent grade and were used as received. Deionized water was used throughout the experiment. All analytes were diluted with 10 mM PBS buffer (pH 7.4) for variable concentrations.
Sensor fabrication
First, well-defined soft nanogrooves were formed using a flat hybrid PDMS substrate, which was fabricated by thermal nanoimprint lithography.68 The formed template was combined with a PET flexible substrate (3 mm × 3 mm). Thereafter, a mixing ink (PEDOT:PSS-doped PLL-g-OEG4-biotin) with a special molecular design was applied between the template and substrate. The ink adhered to the silicon substrate according to the template shape and formed a uniform nanowire array with an average width of 100 nm and spacing between nanowires alternating between 3 and 5 μm. Next, a gold layer was deposited via thermal evaporation through a shadow mask with an interdigitated structure. Finally, SAv proteins were applied to the surface of the sensor and bound to the biotin groups of the nanowires through stable covalent bonds with two sites on one side of SAv. After 10 min of incubation, biotin–GOx was bound to the two sites on the other side of SAv that were available to connect to the nanowires and achieve enzyme immobilization. Using this method, the microwave biosensor based on a nanowire array with GOx was fabricated.
Instruments and measurements
After fabrication, the sensor design consists of a 50 Ω coplanar waveguide transmission line, which then is packaged with two port SMA connectors. A vector network analyzer (Keysight E5071C) was used to measure the sensor response to different glucose samples, and the changes of |S11| were observed in the GHz range. Finally, in the testing of sensor attachment to the skin, a miniaturized vector network analyzer (VNA 6000A, Xuanli Electronics Corporation, China) and a tablet were used.
Conflicts of interest
The authors declare no conflicts of interest.
Acknowledgements
The authors gratefully acknowledge financial support from the National Natural Science Foundation of China (NSFC No. 61674114, 91743110, 21861132001), National Key R&D Program of China (2017YFF0204604, 2018YFE0118700), Tianjin Applied Basic Research and Advanced Technology (17JCJQJC43600), the 111 Project (B07014), the Initial Scientific Research Fund of State Key Laboratory of Precision Measuring Technology and Instruments, Tianjin University (No. Pilq1902), and the Foundation for Talent Scientists of Nanchang Institute for Micro-technology of Tianjin University.
Notes and references
- W. Gao, S. Emaminejad, H. Y. Y. Nyein, S. Challa, K. Chen, A. Peck, H. M. Fahad, H. Ota, H. Shiraki, D. Kiriya, D.-H. Lien, G. A. Brooks, R. W. Davis and A. Javey, Nature, 2016, 529, 509–514 CrossRef CAS PubMed.
- M. Poh, N. C. Swenson and R. W. Picard, IEEE Trans. Biomed. Eng., 2010, 57, 1243–1252 Search PubMed.
- F. Lin, A. Wang, Y. Zhuang, M. R. Tomita and W. Xu, IEEE Trans. Ind. Informat., 2016, 12, 2281–2291 Search PubMed.
- T. Yang, X. Jiang, Y. Zhong, X. Zhao, S. Lin, J. Li, X. Li, J. Xu, Z. Li and H. Zhu, ACS Sens., 2017, 2, 967–974 CrossRef CAS PubMed.
- P. Adhikary, A. Biswas and D. Mandal, Nanotechnology, 2016, 27, 495501 CrossRef PubMed.
- X.-G. Yu, Y.-Q. Li, W.-B. Zhu, P. Huang, T.-T. Wang, N. Hu and S.-Y. Fu, Nanoscale, 2017, 9, 6680–6685 RSC.
- P. Slobodian, R. Danova, R. Olejnik, J. Matyas and L. Münster, Polym. Adv. Technol., 2019, 30, 1891–1898 CrossRef CAS.
- N. Tang, C. Zhou, L. Xu, Y. Jiang, H. Qu and X. Duan, ACS Sens., 2019, 4, 726–732 CrossRef CAS PubMed.
- Y.-D. Lee and W.-Y. Chung, Sens. Actuators, B, 2009, 140, 390–395 CrossRef CAS.
- Y. Yamamoto, D. Yamamoto, M. Takada, H. Naito, T. Arie, S. Akita and K. Takei, Adv. Healthcare Mater., 2017, 6, 1700495 CrossRef PubMed.
- L. Viry, A. Levi, M. Totaro, A. Mondini, V. Mattoli, B. Mazzolai and L. Beccai, Adv. Mater., 2014, 26, 2659–2664 CrossRef CAS PubMed.
- M. Jian, K. Xia, Q. Wang, Z. Yin, H. Wang, C. Wang, H. Xie, M. Zhang and Y. Zhang, Adv. Funct. Mater., 2017, 27, 1606066 CrossRef.
- Y. Chang, J. Zuo, H. Zhang and X. Duan, Nanotechnol. Precis. Eng., 2020, 3, 43–52 CrossRef.
- W.-P. Shih, L.-C. Tsao, C.-W. Lee, M.-Y. Cheng, C. Chang, Y.-J. Yang and K.-C. Fan, Sensors, 2010, 10, 3597–3610 CrossRef CAS PubMed.
- R. Alrammouz, J. Podlecki, A. Vena, R. Garcia, P. Abboud, R. Habchi and B. Sorli, Sens. Actuators, B, 2019, 298, 126892 CrossRef CAS.
- C. Zhou, X. Zhang, N. Tang, Y. Fang, H. Zhang and X. Duan, Nanotechnology, 2020, 31, 125302 CrossRef PubMed.
- C. Wang, K. Xia, H. Wang, X. Liang, Z. Yin and Y. Zhang, Adv. Mater., 2019, 31, 1801072 CrossRef PubMed.
- Z. Zhang, M. Azizi, M. Lee, P. Davidowsky, P. Lawrence and A. Abbaspourrad, Lab Chip, 2019, 19, 3448–3460 RSC.
- Y. Lin, M. Bariya, H. Y. Y. Nyein, L. Kivimäki, S. Uusitalo, E. Jansson, W. Ji, Z. Yuan, T. Happonen, C. Liedert, J. Hiltunen, Z. Fan and A. Javey, Adv. Funct. Mater., 2019, 29, 1902521 CrossRef.
- S. Haxha and J. Jhoja, IEEE Photonics J., 2016, 8, 1–11 Search PubMed.
- X. Xue, Z. Qu, Y. Fu, B. Yu, L. Xing and Y. Zhang, Nano Energy, 2016, 26, 148–156 CrossRef CAS.
- E. Porter, H. Bahrami, A. Santorelli, B. Gosselin, L. A. Rusch and M. Popović, IEEE Trans. Med. Imag., 2016, 35, 1501–1509 Search PubMed.
- G. Gennarelli, S. Romeo, M. R. Scarfì and F. Soldovieri, IEEE Sens. J., 2013, 13, 1857–1864 Search PubMed.
- J. Yao, S. Tjuatja and H. Huang, IEEE Sens. J., 2015, 15, 4338–4345 CAS.
- X. Yi, T. Wu, Y. Wang, R. T. Leon, M. M. Tentzeris and G. Lantz, Int. J. Smart Nano Mater., 2011, 2, 22–38 CrossRef.
- T.-G. Kang, J.-K. Park, G.-H. Yun, H. H. Choi, H.-J. Lee and J.-G. Yook, Sens. Actuators, B, 2019, 282, 145–151 CrossRef CAS.
- D. Girbau, A. Ramos, A. Lazaro, S. Rima and R. Villarino, IEEE Trans. Microw. Theory Techn., 2012, 60, 3623–3632 Search PubMed.
- X. Wang, O. Larsson, D. Platt, S. Nordlinder, I. Engquist, M. Berggren and X. Crispin, Sens. Actuators, B, 2012, 166-167, 556–561 CrossRef CAS.
- X. Zhang, C. Ruan, T. U. Haq and K. Chen, Sensors, 2019, 19, 787 CrossRef PubMed.
- D. Agranovich, I. Renhart, P. Ben Ishai, G. Katz, D. Bezman and Y. Feldman, Food Control, 2016, 63, 195–200 CrossRef CAS.
- Y.-F. Chen, H.-W. Wu, Y.-H. Hong and H.-Y. Lee, Biosens. Bioelectron., 2014, 61, 417–421 CrossRef CAS PubMed.
- H.-J. Lee, J.-H. Lee, H.-S. Moon, I.-S. Jang, J.-S. Choi, J.-G. Yook and H.-I. Jung, Sens. Actuators, B, 2012, 169, 26–31 CrossRef CAS.
- Q. Xue, X. Tang, Y. Li, H. Liu and X. Duan, ACS Sens., 2019, 5, 171–179 CrossRef PubMed.
- A. Mason, O. Korostynska, J. Louis, L. E. Cordova-Lopez, B. Abdullah, J. Greene, R. Connell and J. Hopkins, IEEE Trans. Biomed. Eng., 2018, 65, 698–705 CAS.
- T. Chen, S. Li and H. Sun, Sensors, 2012, 12, 2742–2765 CrossRef PubMed.
- Z. Jakšić, S. Vuković, J. Matovic and D. Tanasković, Materials, 2011, 4, 1–36 CrossRef PubMed.
- Z. Zhang, Z. Tian, C. Chang, X. Wang, X. Zhang, C. Ouyang, J. Gu, J. Han and W. Zhang, Nanotechnol. Precis. Eng., 2018, 1, 123–128 Search PubMed.
- Y. Li, L. Su, C. Shou, C. Yu, J. Deng and Y. Fang, Sci. Rep., 2013, 3, 2865 CrossRef PubMed.
- H. Bilgin, S. Zahertar, S. Sadeghzadeh, A. D. Yalcinkaya and H. Torun, Sens. Actuators, A, 2016, 244, 292–298 CrossRef CAS.
- Y. Zhang, J. Zhao, J. Cao and B. Mao, Sensors, 2018, 18, 1912 CrossRef PubMed.
- Q. Xue, Q. Wang, Z. Han, N. Tang, C. Zhou, W. Pan, Y. Wang and X. Duan, Adv. Mater. Interfaces, 2019, 6, 1970118 CrossRef.
- S. Dalirirad and A. J. Steckl, Sens. Actuators, B, 2019, 283, 79–86 CrossRef CAS.
- M. Wu, H. He, Z. Zhao and X. Yao, J. Phys. D: Appl. Phys., 2000, 33, 2398 CrossRef CAS.
- Y. Chen, M. Cao, T. Wang and Q. Wan, Appl. Phys. Lett., 2004, 84, 3367–3369 CrossRef CAS.
- S. Jo, D. Banerjee and Z. Ren, Appl. Phys. Lett., 2004, 85, 1407–1409 CrossRef CAS.
- D. Banerjee, S. H. Jo and Z. F. Ren, Adv. Mater., 2004, 16, 2028–2032 CrossRef CAS.
- D. Shreiber, M. Gupta and R. Cravey, Sens. Actuators, A, 2011, 165, 256–260 CrossRef CAS.
- Y.-H. Ye, Y. J. Huang, W. T. Lu, B. D. F. Casse, D. Xiao, S. P. Bennett, D. Heiman, L. Menon and S. Sridhar, Opt. Mater., 2011, 33, 1667–1670 CrossRef CAS.
- B. G. McMillan, L. E. A. Berlouis, F. R. Cruickshank, D. Pugh and P.-F. Brevet, Appl. Phys. Lett., 2005, 86, 211912 CrossRef.
-
R. Liu, Z. Zhang, R. Zhong, X. Chen and J. Li, Texas Deparment of Transportation, Austin, 2007.
- J. Zhou, D. H. Anjum, L. Chen, X. Xu, I. A. Ventura, L. Jiang and G. Lubineau, J. Mater. Chem. C, 2014, 2, 9903–9910 RSC.
- Z. Han, Y. Wang and X. Duan, Anal. Chim. Acta, 2017, 964, 170–177 CrossRef CAS PubMed.
- H. Ito, F. Nakajima, T. Furuta and T. Ishibashi, Semicond. Sci. Technol., 2005, 20, S191–S198 CrossRef CAS.
- V. Rawat, S. Dhobale and S. N. Kale, J. Appl. Phys., 2014, 116, 164106 CrossRef.
- T. Kong, Y. Chen, Y. Ye, K. Zhang, Z. Wang and X. Wang, Sens. Actuators, B, 2009, 138, 344–350 CrossRef CAS.
- P. A. Raymundo-Pereira, F. M. Shimizu, D. Coelho, M. H. O. Piazzeta, A. L. Gobbi, S. A. S. Machado and O. N. Oliveira, Biosens. Bioelectron., 2016, 86, 369–376 CrossRef CAS.
- T. Yasukawa, K. Goto and F. Mizutani, Electroanalysis, 2010, 22, 927–930 CrossRef CAS.
- A. Blandino, M. Macías and D. Cantero, Process Biochem., 2001, 36, 601–606 CrossRef CAS.
- P. R. Nair and M. A. Alam, Appl. Phys. Lett., 2006, 88, 233120 CrossRef.
- F. Wu, S. Zhang and Z. Tao, Mater. Corros., 2011, 62, 234–239 CrossRef CAS.
- M. E. B. Jalil, M. K. Abd Rahim, N. A. Samsuri, N. A. Murad, H. A. Majid, K. Kamardin and M. Azfar Abdullah, Prog. Electromagn. Res., 2013, 140, 633–652 CrossRef.
- F. Boeykens, H. Rogier and L. Vallozzi, IEEE Trans. Antennas Propag., 2014, 62, 1253–1260 Search PubMed.
- R. D. Munje, S. Muthukumar and S. Prasad, Sens. Actuators, B, 2017, 238, 482–490 CrossRef CAS.
- J. D. Yuen, S. A. Walper, B. J. Melde, M. A. Daniele and D. A. Stenger, Sci. Rep., 2017, 7, 40867 CrossRef CAS PubMed.
- X. Xuan, H. S. Yoon and J. Y. Park, Biosens. Bioelectron., 2018, 109, 75–82 CrossRef CAS PubMed.
- J. Nehring, M. Dietz, K. Aufinger, G. Fischer, R. Weigel and D. Kissinger, IEEE Trans. Microwave Theory Tech., 2016, 64, 892–905 Search PubMed.
- J. Moyer, D. Wilson, I. Finkelshtein, B. Wong and R. Potts, Diabetes Technol. Ther., 2012, 14, 398–402 CrossRef CAS PubMed.
- B. D. Gates, Q. Xu, J. C. Love, D. B. Wolfe and G. M. Whitesides, Annu. Rev. Mater. Res., 2004, 34, 339–372 CrossRef CAS.
Footnotes |
† Electronic supplementary information (ESI) available. See DOI: 10.1039/d0nh00098a |
‡ Qiannan Xue and Zheyu Li contributed equally to this work. |
|
This journal is © The Royal Society of Chemistry 2020 |