DOI:
10.1039/C9NH00719A
(Communication)
Nanoscale Horiz., 2020,
5, 730-738
Photothermal actuated origamis based on graphene oxide–cellulose programmable bilayers†
Received
13th November 2019
, Accepted 5th February 2020
First published on 5th February 2020
Abstract
The design and construction of 3D architectures enabled by stimuli-responsive soft materials can yield novel functionalities for next generation soft-bodied actuating devices. Apart from additive manufacturing processes, origami inspired technology offers an alternative approach to fabricate 3D actuators from planar materials. Here we report a class of near-infrared (NIR) responsive 3D active origamis that deploy, actuate and transform between multistable structural equilibria. By exploiting the nonlinear coefficient of thermal expansion (CTE) of graphene oxide (GO), graphene oxide/ethylene cellulose (GO/EC) bilayers are readily fabricated to deliver precise origami structure control, and rapid low-temperature-triggered photothermal actuation. Complexity in 3D shapes is produced through heterogeneously patterning GO domains on 2D EC thin films, which allows us to customize 3D architectures that adapt to various robotic functions. The strategy also enables the construction of material systems possessing naturally inaccessible properties, such as remotely controlled mechanical metamaterials with auxetic behavior and bionic flowers with a rapid blooming rate. Harnessing deformability with multiple degrees of freedom (DOF) upon light irradiation, this work leads to breakthroughs in the design and implementation of shape-morphing functions with soft origamis.
New concepts
Two-dimensional nanomaterials possessing unique physical and chemical properties have been widely employed to fabricate stimuli-responsive devices. Graphene oxide (GO), characterized by a highly negative coefficient of thermal expansion (CTE) and superior photothermal conversion capability, is a promising candidate for the construction of near-infrared (NIR) driven bilayer actuators, yet the humidity-dependent microstructural evolution within GO assembly has seldom been studied to reveal its significant contribution in the rapid, efficient and reversible photothermal actuation. In this work, we, for the first time, unravel the interplay between thermal conditions and water molecules’ reversible adsorption/desorption in GO via in situ thermal X-ray diffraction (XRD), and elucidate how the nonlinear CTE of GO can lead to low-temperature actuation in the as prepared GO–cellulose bilayers. Combined with mechanical finite element simulations, the actuating mechanism involving a complex photo-thermo-mechanical energy transducing behavior is further clarified to provide a rational designing guidance for photothermal actuators. These fundamental discoveries lead to the fabrication of programmable origamis capable of multistable shape-morphing under different NIR inputs, which offers novel opportunities for next-generation autonomous, untethered soft robotics and biomimetic devices.
|
Introduction
The combination of soft smart materials and advanced manufacturing technologies has given birth to diverse shape programmable 3D architectures ranging from the macroscopic to the mesoscopic1,2 scale. Complementary to the well-known additive 3D printing technology, origami, the centuries-old art exploiting 2D to 3D transformations, has been infused with planar engineering methods to enable top-down parallel formation of 3D geometries from 2D sheets. Taking inspiration from paper crafts (i.e. origami, kirigami and pop-up book), thin-film materials are firstly assigned or tailored as planar precursors, then reconfigured into target 3D shapes through deterministic transformation induced by internal stress3–5 or external modulation.6–9 Moreover, instead of static 3D structures, “active origamis” with autonomous and controllable deformability have been implemented in advanced research fields such as soft robotics,10 flexible electronics11 and biomedicine.12 These systems ingeniously leverage existing actuator techniques to achieve reversible and multimodal locomotion in 3D architectures, among which photothermal-mechanical transduction is a promising energy conversion route possessing advantages of wireless stimulation and remote motion control.13
An assembly strategy namely “asymmetric bilayer” has been widely adopted to fabricate energy-efficient photothermal actuators (PTAs), where mismatched coefficient of thermal expansion (CTE) between counterpart layers will lead to bending or torsion under selected light irradiation.13,14 Carbon allotropes, including graphite,15 carbon nanotubes (CNTs),16–21 graphene22–28 and graphene oxide (GO),29,30 are considered as superior active materials for bilayer PTAs due to their excellent photothermal conversion efficiency and heat conductivity. Apart from the prevalent flat-shaped bimorph PTAs, several recent works have demonstrated self-curing or rolling bilayer PTAs possessing 3D geometries under ambient conditions,19–23 yet they only provide limited precision in geometrical control and undesirable actuating speed while responding to external stimuli. As a result, significant challenges still exist in delivering PTA origamis with high DOF to perform exquisite robotic tasks in a programmable manner.
Herein we report an innovative strategy using 2D precursors to devise complex active origamis that exhibit various 3D architectures at room temperature. By studying the humidity-dependent negative thermal expansion behavior of GO assemblies,31 PTAs based on graphene oxide/ethylene cellulose (GO/EC) bilayers are successfully prepared to deliver rapid, reversible and low power triggered photothermal actuation. Patterning GO onto an ethylene cellulose (EC) substrate in a heterogeneous manner leads to segmented bilayer domains with varying bending directions and degrees, whilst curvature control in each domain is facilitated by manipulating residual thermal stress along the interface between GO self-assembly and EC. Our PTA-based active origamis are soft, lightweight, and can sustain multistable shape transformations attributing to the photo-thermo-mechanical transducing behavior of the bilayers. Benefitting from the versatility and simplicity of our planar fabricating process, various shape formats from tubular bimorphs to complex morpho-functional origamis are demonstrated, which elucidates the pathway towards agile control in soft robotics, self-propelling mechanical metamaterials as well as biomimetic applications.
Results and discussion
Generation of shape programmable bilayer origamis
Identifying materials set with a larger CTE mismatch is pivotal to deliver intensive deformation and fast actuation kinematics in PTAs. While most intrinsic negative thermal expansion (NTE) materials display a small NTE effect (e.g. graphene has a negative yet tiny CTE value of about −7 ppm K−1 at 300 K),32 GO is an exception in which the NTE behavior is highly correlated with water molecules’ reversible removal/intercalation between adjacent atomic layers, and its non-constant CTE shows a more negative value in a humid environment than in the dry state (−130 ppm K−1 for 25% and −68 ppm K−1 for 2% relative humidity (RH)).31 On the other hand, cellulose is a cluster of environment-friendly soft matters which can serve as soft substrates for flexible electronics33,34 and energy storage devices.35 Among all its derivatives we selected EC for PTA construction considering its highly positive CTE (∼150 ppm K−1)36 and favorable mechanical properties (refer to tensile test results in Note S1 and Fig. S1, S2, ESI†). Based on these material-level advancements, central to yielding room temperature-stable 3D architectures is to facilitate the bilayer's preparation at a non-ambient temperature.
As an illustrative example to showcase the facile fabrication protocol of our 3D-PTA, a bimorph with 5
:
1 aspect ratio (25 mm
:
5 mm) was firstly produced as depicted in Fig. 1a. Here an EC thin film served as a planar precursor with GO dispersion uniformly cast on top and dried in an ambient environment. Then the composite underwent a T0 = 90 °C vacuum oven annealing process, through which a GO/EC bimorph was monolithically formed with eliminated internal stress. During annealing the sample was sandwiched between glass plates to enhance lamination and maintain flatness. Thereafter, the action of taking the bimorph back to the ambient condition (T ≈ 25 °C, RH ≈ 70%) introduced a negative temperature gradient as ΔT = T − T0 = −65 °C, upon which GO with the negative CTE expanded, while EC with the positive CTE contracted, and the beam scrolled towards the EC side to reach its mechanics equilibrium guided by the inbuilt stress. Surface morphology evolution in GO has been observed assisted by optical confocal microscopy. As differentiated between Fig. 1b and c, the GO thin film prior to thermal treatment exhibited a root mean square (rms) surface roughness of ca. 0.97 μm, while the post-annealed sample was roughened to be ca. 1.53 μm. The enhancement of surface wrinkling implied an increased lateral interlocking of 2D lamellae attributed to an irreversible free water loss as confirmed by a peak shift in the X-ray diffraction (XRD) pattern (Fig. S3, ESI†). The self-assembling nature of individual GO sheets also resulted in a condensed interlamellar stacking and a coherent GO/EC interface (characterized by SEM in Fig. 1d).
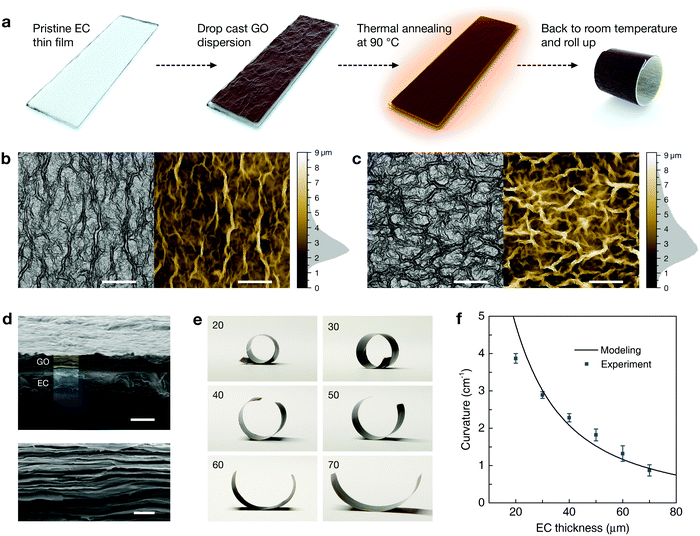 |
| Fig. 1 Fabrication, characterization and geometry control of bimorph 3D-PTAs. (a) Schematic of the 3D-PTA fabrication process. (b and c) Optical and confocal microscopic images of the GO surface (b) before and (c) after 90 °C thermal annealing. Histograms beside the color palette represent height distribution and surface roughness. (d) Cross-sectional SEM images of the GO/EC bilayer (top) and magnification of the condensed GO stack (bottom). (e) Photographs of bimorph 3D-PTAs with various EC thicknesses. Label unit: μm. (f) Experimental results and theoretical prediction of bimorph curvature variation over EC thickness change. Bimorphs with each EC thickness were fabricated in ten sets with error bars presented. Scale bars: 100 μm in (b and c), 20 μm for the top and 2 μm for the bottom image in (d). | |
3D-PTAs require predictable degrees of cylindrical curling (assessed by curvature, Fig. S4a, ESI†) to deliver high-fidelity geometries with reliable repeatability. We hereby developed a theoretical model (see derivation and discussion in Note S2 and Fig. S5, ESI†) to understand the governing parameters in the determination of coil curvature, and to provide design guidelines for more complicated 3D structures. The describing equation reveals that the ultimate curvature (κ) in the GO/EC bimorph is related to the fabrication conditions, both materials’ thermal and mechanical properties, as well as their spatial geometries:
where
m =
t1/
t2,
n =
E1/
E2;
α1,
t1,
E1 are the CTE, thickness and Young's modulus of EC, while
α2,
t2,
E2 are the corresponding parameters of GO, respectively. Specifically, the product of temperature gradient (Δ
T) and CTE mismatch is linearly proportional to the curvature, while the film thickness and Young's modulus of GO and EC affect the self-rolling magnitude in nonlinearity. In view of the complexity in precisely modifying the physical properties of both materials, realizing geometric control through adjusting the thickness ratio of GO/EC is expected to be feasible. Experimentally we achieved spatially varying curvatures
via altering the thickness of the EC film while fixing the dosage of GO dispersion (a 1.5 mg cm
−2 dose leads to
ca. 10 μm thickness in the GO layer, examined by SEM in
Fig. 1d). When 20 μm thick EC was applied, the GO/EC bilayer rolled up intensively into a tubular shape with a curvature as high as 3.87 ± 0.13 cm
−1 (see the curvature evaluation method in Fig. S4b, ESI
†). The bimorph's curvature decreased monotonically against the increment in EC layer's thickness, and finally ended up bending with a smaller curvature of 0.85 ± 0.16 cm
−1 when EC was as thick as 70 μm. These experimental results as captured in
Fig. 1e are in good agreement with the predictive mechanics model we developed (
Fig. 1f), where the curvature becomes a nonlinear polynomial function of EC's thickness when that of GO is a constant of 10 μm. The model predicts an extreme curvature of 11.24 cm
−1 at a 2.5
![[thin space (1/6-em)]](https://www.rsc.org/images/entities/char_2009.gif)
:
![[thin space (1/6-em)]](https://www.rsc.org/images/entities/char_2009.gif)
1 (GO
![[thin space (1/6-em)]](https://www.rsc.org/images/entities/char_2009.gif)
:
![[thin space (1/6-em)]](https://www.rsc.org/images/entities/char_2009.gif)
EC) thickness ratio (Fig. S6, ESI
†), indicating that further employing thinner EC films will lead to even more intensive self-rolling in bimorph configurations. The influence of RH on curvature is discussed in Fig. S7 (ESI
†).
Photothermal actuation: performance and mechanism
Actuation in PTAs is the macroscopic portrayal of nanoscale mechanisms. Given that a complete energy transducing loop consists of both photothermal and thermo-mechanical conversion processes, the combination of GO and EC provides a simple yet efficient system to achieve both functionalities simultaneously. The contemporary structural model of GO can be interpreted as an atomically thin, sp2-carbon dominated basal plane decorated with disordered oxygen-containing groups either in the plane or on the edge. The monolayer nature of the GO used in this work is characterized via atomic force microscopy (AFM) as shown in Fig. S8 (ESI†). Although oxidation introduced defects in GO inevitably segment the conjugated π network into nanoclusters,37 in phase lattice vibration still substantially exists as confirmed by the strong G band in Raman spectra38 (Fig. S9a, ESI†), which in turn ensures sufficient near-infrared (NIR) absorption (Fig. S10a, ESI†) and photothermal energy transfer through photon–phonon interactions. Concomitantly, as validated by X-ray photoelectron spectroscopy (XPS) analysis (Fig. S9b, ESI†), the enriched oxygen-functional groups in GO provide hydrophilic sites for ultrafast water diffusion and reversible hydration/dehydration upon thermally provoked RH changes.39 At room temperature, the abundant water molecules located at the hydrophilic region act as supportive pillars that hold adjacent GO sheets apart, and the desorption of water under thermal conditions will cause vertical collapse and transverse contraction within the stacked GO assembly,31 resulting in macroscale thermo-mechanical transduction as illustrated in Fig. 2a. With GO serving as the NIR-active and negative CTE layer, EC in contrast exhibits positive thermal expansion when receiving heat flux from GO across their compact interface, and is highly transparent over the spectrum, which enables omnidirectional NIR absorption in GO.
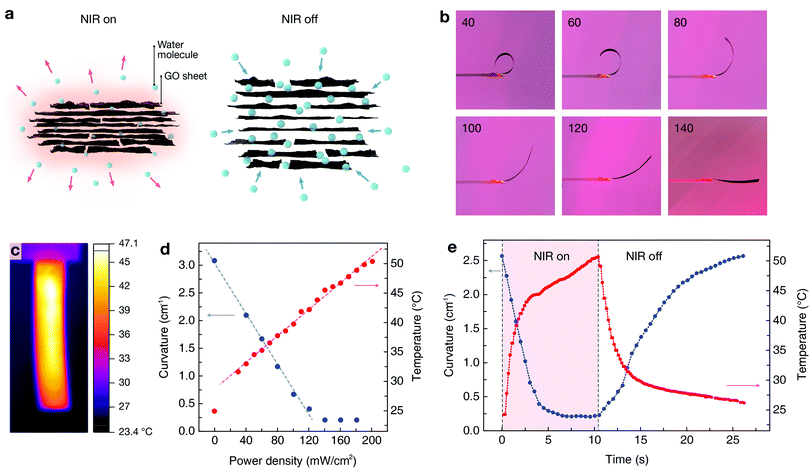 |
| Fig. 2 Photothermal actuation of bimorph 3D-PTAs. (a) Illustration of the NIR triggered thermohydration effect in GO. (b) Morphology change of the bimorph with increasing NIR intensity. Label unit: mW cm−2. (c) Thermogram of the bimorph exposed to 140 mW cm−2 NIR. (d) Corresponding curvature and temperature change of the bimorph as a function of light power density. (e) Diagram recording dynamic curvature and temperature variation during the actuation process under 140 mW cm−2 NIR. | |
The actuation performance of bimorph 3D-PTA (GO
:
EC 10 μm
:
30 μm) under NIR irradiation was studied as a benchmark (see the spectrum of light source in Fig. S10b, ESI†). When the light intensity was increased stepwise, the bimorph gradually uncurled itself to a specific curvature corresponding to each power input (Fig. 2b), suggesting the presence of thermomechanical equilibrium states in the photothermal trajectory, and the onset of complete flattening was under 140 mW cm−2 NIR exposure with an average bimorph temperature as low as 44.5 °C (Fig. 2c). The trends of curvature decrease and temperature rise against the power density ramp are plotted in Fig. 2d, wherein each data set can be fitted into a quasi-linear extrapolation. Fig. 2e and Movie S1 (ESI†) record the dynamic actuation of a bimorph exposed to 200 mW cm−2 NIR. The PTA unrolled ca. 430° within 5 s, then returned rapidly from the temporary flat state to its initial curvature after NIR was switched off. The total actuation amplitude of our 3D-PTA excels significantly when compared to most of the NIR-driven actuators,25–28,40 as well as those actuated by UV41–43 or visible light,17,18,44 without affecting the actuation rate (see comprehensive Ashby plot comparison in Fig. S11, ESI†). NIR with a lower intensity could also fully straighten the bimorph, but the actuation speed would be attenuated (Fig. S12, ESI†). Future attempts to improve the actuating speed of GO/EC PTA could be carried out by hybriding GO with carbon nanotubes (CNTs)20 or graphene nanosheets to achieve higher thermal conductivity in the photothermal active layer.
Conventional planar PTAs are strain-free at room temperature and strained to deform (bend/twist) upon heating. In contrast, self-rolling PTAs endure internal stress in an ambient environment; they are expected to fully flatten only when being heated up till their curing temperature T0 due to the complete relaxation of accumulated stress.20 Our 3D-PTAs manifested a low temperature actuating performance during photothermal heating. As shown in Fig. 2d, the temperature of the bimorph PTA increased almost linearly when NIR irradiation was increased from 0 to 200 mW cm−2, while its actuation started to saturate (fully unroll) from 45 °C (140 mW cm−2 NIR) onwards, way before reaching T0 = 90 °C. Besides, the PTA's recovery (rerolling) rate was slower than that of its actuation (unrolling, Fig. 2e). These phenomena indicate that the actuation mechanism cannot be simply explained by linear-CTE modulation, whereas the peculiar thermohydration trait of GO may play a role in the apparent temperature–curvature asymmetry. The first clue we reasoned was that RH decreases nonlinearly with temperature increment (refer to the Antoine equation and RH change against temperature in Fig. S13a, ESI†). Concurrently, water desorption in GO was more drastic in the low temperature region than at a higher temperature during thermal gravimetric analysis (TGA). As shown in Fig. S13b (ESI†), 11% of the total weight was lost at 50 °C, while the overall weight loss at 90 °C only increased up to 15%. We further employed in situ thermal XRD to provide direct evidence of microstructural evolution in GO upon consecutive heating. The fingerprint peak depicting the hydrophilic region shifted from 11.4° to 13.5° when the temperature increased from 30 °C to 110 °C (Fig. 3a), and could revert to 11.7° (Fig. S14, ESI†) upon cooling back to 30 °C. Meanwhile, enhancement of peak intensity was observed along temperature increase since water desorption triggered better alignment in stacked GO sheets. Similar to the trend of weight loss, d-spacing between the adjacent GO atomic layers also decreased in a monotonic yet nonlinear manner through heating (Fig. 3b). Considering temperature increment from 25 °C to 90 °C as the whole thermal trajectory, d-spacing decrement at 50 °C constitutes 79% of the total structural collapse of the layered GO assembly. The above results solidly confirm that water removal/insertion at hydrophilic sites is substantial and reversible, and such a mass transportation process is more prominent in the low temperature region (<50 °C). Therefore, it is expected that the GO layer can generate sufficient thermal contraction at around 50 °C, which is macroscopically reflected as low temperature actuating behavior in GO/EC bilayers.
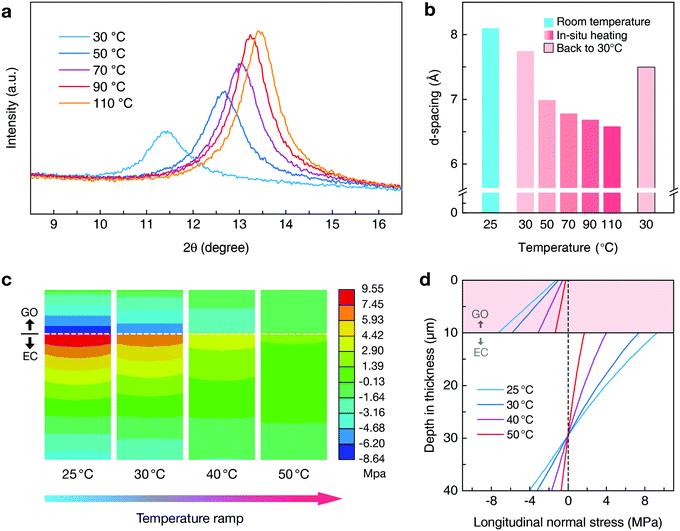 |
| Fig. 3 Actuation mechanism analysis based on in situ thermal XRD and FEA results. (a) XRD patterns of thin-film GO samples through consecutive temperature elevation. (b) Temporal trace of d-spacing for characteristic peaks in (a). (c) FEA results showing longitudinal normal stress distribution within the cross section of the GO/EC bilayer at various temperatures. Here positive values in the color palette represent tensile stress while negative ones stand for compression. (d) Diagram of quantified stress distribution extracted from FEA. GO is entirely compressed; EC is extended in the upper part while slightly compressed in its lower side. The neutral plane in the GO/EC bilayer is fixed at ca. 20 μm beneath the interface irrelevant to temperature change. | |
Stress distribution within the GO/EC bilayer was then investigated by finite element analysis (FEA). In the simulation, different CTE values were assigned to GO in a set of 10 °C intervals. We probed the cross section of the bilayer (Fig. 3c) and extracted its longitudinal normal stress distribution along the thickness direction as plotted in Fig. 3d. It is visualized that, at room temperature (25 °C), the bimorph is strongly strained with GO being compressed (negative stress) while EC being stretched (positive stress) owing to their mutual confinement. Axial stresses accumulate at the bearing interface within the composite and progressively recede towards both surfaces. Heat, or photothermal effect, contributes to the relaxation and flattening of the bimorph by releasing mismatch strain. The simulation result reveals that the internal strain can be freed by ca. 80% at 50 °C, which correlates well with the experimental observation of the low temperature onset of actuation.
Self-propelling origami architectures and mechanical metamaterials
We further exemplify the versatility of our 3D-PTAs by constructing multi-DOF origami architectures beyond standard bimorphs. Planar geometries, such as triangular and annular rings, are able to transform into sophisticated 3D architectures by programming the shape and relative position of self-rolling domains in 2D (dimensional specifications of 2D layouts are available in Fig. S15, ESI†). For instance, the EC thin film can be blade cut into an equilateral triangular ring (Fig. 4a1) followed by drop casting GO dispersion on its front. The as formed triangular bilayer consists of three equivalent beams, which will collectively and symmetrically bend inwards (Fig. 4a2) subsequent to thermal annealing and cooling. Being processed in 2D yet functioning in 3D, such an origami geometry with 3 DOF could be potentially used to trap moving objects given its ability to switch between open and closed states. Moreover, each individual beam in the triangular origami could be independently controlled if more precise NIR sources such as NIR lasers are employed. Another characteristic feature of our planar process is that one 2D precursor may end up having disparate 3D shapes due to arbitrary GO layouts. Assigning GO on top, or beneath EC at specific regions will dictate localized, bidirectional curvatures (positive or negative); meanwhile, the relative coverage of each GO domain also affects the ultimate contour of complex 3D architectures. We exemplified such a vision via patterning GO underneath EC in the shaded area while maintaining it above in the bare region according to the prescribed graphic design (Fig. 4b1–d1). As shown in Fig. 4b2, still utilizing the triangular EC substrate, the domains are programmed in a way that each beam bends convexly by half while concavely in the other bisection. Such a 2D plot renders a periodically undulating 3D frame structure which is highly distinct to its gripper-like counterpart. Another set of experiments was carried out with respect to annular rings. In Fig. 4c1 and d1 we designed two annuli with identical 2D geometry but variant styles of GO assignment, with alternating GO domains equidistantly patterned in one sample yet unequally in the other. Although both consequent 3D shapes look undulatory, they show inherent divergence in spatial symmetry, as the 3D annulus in Fig. 4c2 is centrosymmetric while the one in Fig. 4d2 is axisymmetric. Apart from microscale stress analysis, FEA can also predict macroscopic deformation of the origamis. We developed an annular model with the characterized thermal/mechanical parameters being assigned (Fig. 4e). The planar outline represents the strainless annulus at 90 °C, while its 3D architecture at room temperature (color scheme) could be closely simulated in agreement with the experimental result. Therefore, FEA can serve as a powerful tool for fast prototyping of unexplored 3D origami architectures. Furthermore, all above mentioned active origamis can reversibly actuate owing to the collective motions of GO/EC domains (Movie S2, ESI†), which enables metamorphoses between their 3D modes and flattened 2D states (Fig. 4a3, b3, d3 and e3), and characterizes them as self-deployable structures which can transform into space-efficient flat formats as needed. As cycling durability is important for PTAs, we exposed the 3D origamis to 200 continuous actuation cycles and found that the geometry remained almost unchanged as shown in Fig. S16a (ESI†). Despite their inherent compliance and lightweight nature, the actuations of these 3D architectures are by no means limited to unloaded modes. We examined the weight-lifting performance of a centrosymmetric annulus, which weighs merely 14.8 mg, by quantifying its actuation strokes and specific works under various normal loads (Fig. 4f, see Fig. S16b, ESI† for experimental details). Under a moderate load of 170 mg (1.67 mN), the PTA could fully revert to its 3D state from flatness with a ca. 8 mm stroke (20
000% of flat thickness). Peak specific work capacity of 3.38 J kg−1 occurred at 1020 mg resisting load (Fig. 4g), revealing the high thrust-to-weight ratio (ca. 69) achieved by our 3D-PTA origamis for robotic functionalities. Compared with other stimuli-responsive origamis (summarized in Table S2, ESI†), our PTA-based origami architectures are lightweight, less power consuming, remotely actuated, and render larger strokes as well as a moderate thrust-to-weight ratio.
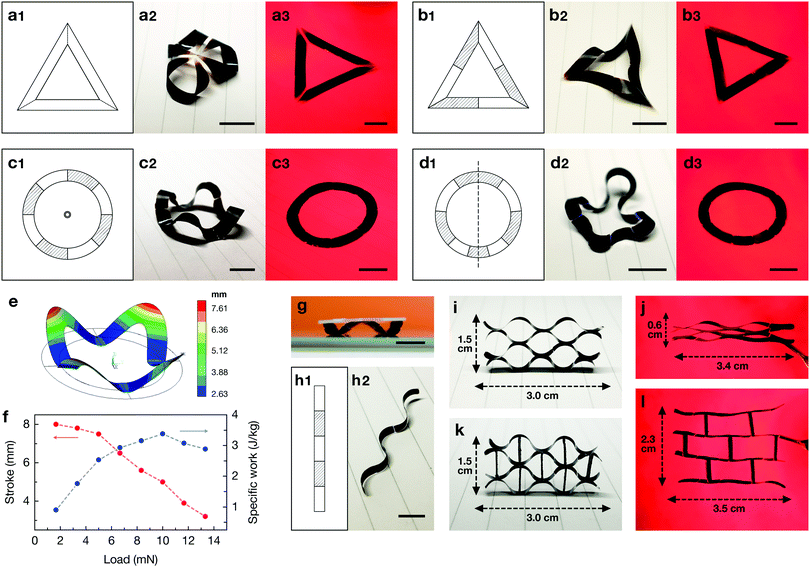 |
| Fig. 4 Various shape programmable 3D architectures derived from 2D layouts. (a–e) Graphic designs, room temperature stable 3D modes and high temperature planar states of (a) an equilateral triangular ring encoded with only positive curvature; (b) an equilateral triangular ring encoded with alternating positive and negative curvatures; (c) an annulus programmed with central symmetry; and (d) an annulus programmed with axial symmetry. (e) FEA macroscopic prediction of the centrosymmetric annulus. The color scale represents vertical displacement of the elements. (f) Weight-lifting performance of a centrosymmetric annulus origami as a function of resisting load. (g) Photograph of the origami lifting 1020 mg load. (h) Graphic design and 3D state of a wavy strip with equidistant domains. (i–l) 3D-PTA enabled mechanical metamaterials including (i and j) an assembly strategy for ultra-positive Poisson's ratio and (k and l) an assembly strategy for negative Poisson's ratio. The length direction of the stripe is regarded as the axial direction for Poisson's ratio calculation. All demonstrations shown above were fabricated with 10 μm GO and 30 μm EC. Reversible actuations were performed under 200 mW cm−2 NIR irradiation. Scale bars: 1 cm in (a–d) and (f), 0.5 cm in (g). | |
The concept of shape programming can be further expanded by integrating individual 3D-PTA units into one assembly with multiple DOF, which is attractive to yielding materials with conventionally inaccessible mechanical properties and functionalities, that is to say, mechanical metamaterials.45 Here we constructed metamaterials characterized by unusual Poisson's ratio utilizing the wavy-strip 3D-PTAs (Fig. 4h) as unit building blocks, through which strong heterogeneities in Poisson's ratios were established via differential assembling strategies. For instance, when four initially separated PTA strips were bonded together at their contacting hinges (Fig. 4i), they constituted a system which collapsed vertically while only slightly extended in the lateral direction under NIR activation (Fig. 4j), leading to a highly positive Poisson's ratio of 3.6. Furthermore, the entity could be reconfigured into a re-entrant honeycomb structure46 endowed with auxetic behavior by joining 3D-PTA stripes with NIR-nonresponsive stiff paper as solid, bracing connections (Fig. 4k). It exhibited expansion in both length and width when stimulated by light irradiation (Fig. 4l), since the straightening and alignment of wavy units along the horizontal direction will motivate vertical repelling through hinging motions, and a negative Poisson's ratio of −2.4 was obtained. The deformation of auxetic metamaterials are commonly driven by mechanical force induced stretch or compression due to the restricted usage of passive structural materials. In contrast, guided by our active origami scheme, untethered mechanical metamaterials capable of shape encoding, self-propelling and remote control have been realized, as both aforementioned meta-structures can rapidly switch between their high temperature and room temperature stable configurations without physical contact (Movie S3, ESI†).
Biomimetic origami
Biological systems can skillfully adopt the origami approach to create tissue and organs.47 For example, early-stage plant organs, such as flower buds (Fig. 5a), are generally configured into the folded status with deterministic folding patterns. These contractive conformations will subsequently unfold and flatten during organism maturation. In our case, we constructed botanical analogies with shape programmable 3D-PTAs and replicated the aforementioned plant growth processes harnessing the photothermal effect. A double-layered origami flower was firstly designed planarly in a floral form as depicted in Fig. 5b (dimensions specified in Fig. S17, ESI†). Since the initial petal curvature can be encoded by the EC paper's thickness, we chose 50 μm and 30 μm EC papers for outer and inner petals respectively to showcase the concept of spatial bio-gradient. As shown in Fig. 5c and d, translation from 2D layouts into 3D petals was achieved through an annealing induced curvature rendering process, and both petal groups exhibited uniform curvature owing to the excellent repeatability of our 3D-PTA generation protocol. Similarly, temperature variation serves as the trigger for shape-morphing in this plant-inspired system. By applying NIR with a crescendo of intensity, the artificial flower could mimic a series of intermediate blossom states until fully flattened (Fig. 5e, thermogram available in Fig. S18, ESI†), which declares its thermomechanical multistability property. The rapid and reversible dynamic deforming process under 200 mW cm−2 NIR is recorded in Movie S4 (ESI†). The amazing blooming rate of the origami bionic device is much faster than those of many flowers in nature including the much admired cereus or epiphyllum.
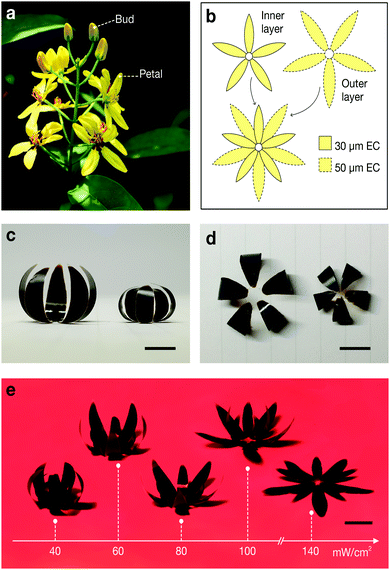 |
| Fig. 5 Biomimetic origami flower manifesting photothermal-triggered bloom. (a) Photograph of gold climber (Tristellateia australasiae A. Rich) buds and flowers revealing morphological differences before and after organism maturation. The photograph was taken by the author. (b) 2D graphical design for inner and outer layers of the origami flower. (c) Side view and (d) top view of already formed inner and outer petal groups at room temperature 3D states. (e) Morphology evolution of the origami flower along increasing NIR intensity. Scale bars: 1 cm in (c–e). | |
Conclusions
Our GO/EC bilayer system relies on a combination of material properties and geometrical design to produce spatially programmable and dynamically reconfigurable active origamis. The as derived mechanics model and FEA prediction allow us to precisely program origamis from planar processes, and the mechanism for fast, low temperature, and fully reversible photothermal actuation has been investigated on the nanoscale considering the thermohydration effect. By introducing novel features, such as domain patterning and multistable shape-morphing, into the fabrication of soft 3D-PTA origamis, this technique provides a platform on which tunable functionalities can be achieved on demand for soft robotics, mechanical metamaterials, artificial bio-systems and beyond.
Experimental
Materials preparation
8 wt% EC powder (Sigma-Aldrich 200654) was dissolved in a toluene/ethanol (4
:
1 volume ratio) binary solvent with stirring and a 70 °C water bath. The as formed viscous EC solution was bar coated onto a polyethylene terephthalate (PET) film, heated at 60 °C to fully evaporate the solvent, and subsequently peeled off to obtain EC films (thicknesses varying from 20 to 70 μm controlled by bar coating spacer). GO flakes were synthesized from commercial graphite powder (Sigma-Aldrich 282863) via a modified Hummers’ method.48 GO dispersion of 9 mg ml−1 was obtained by dilution and 30 min ultrasonication. GO thin films were prepared on silicon wafers for XRD characterization through drop casting, air drying and 12 hours 90 °C vacuum oven annealing. The film could be peeled off from the substrate for mechanical and optical tests.
Materials characterization
An optical confocal microscope (ZEISS, Smartproof5) was used to characterize the surface morphology and rms roughness of the GO assembly. A field emission SEM (JEOL 7600) was used to reveal the cross section of the GO/EC bilayer. An AFM (Oxford Instrument, Cypher S) was employed to determine the layer thickness and relative surface area of GO. Structural/chemical information of GO including lattice vibration modes and degree of oxidation was obtained using a Raman spectroscope (Thermo Scientific, iS 50) and an XPS (Kratos Analytical, AXIS Supra+). Light absorbance was measured using a UV-vis-NIR spectrophotometer (PerkinElmer, Lambda 950). Heat induced weight loss in GO was monitored by TGA (TA Instruments, Q500). In situ thermal XRD experiment was performed using a Bruker D8 DISCOVER (Cu-Kα radiation, λ = 1.5406 Å) with 1 °C min−1 heating rate and 30 minutes temperature stabilization before each scanning run.
Finite element analysis
FEA was conducted using commercial finite element software (ANSYS Workbench) with a steady-state thermal module. For stress distribution analysis (Fig. 3c and d) we constructed a representative volume element with real scale thickness (GO/EC 10 μm/30 μm) yet shrunken lateral dimensions of 100 μm × 100 μm to enhance the fineness of hexahedron meshing. For macroscopic shape prediction (Fig. S14, ESI†) the model was built with identical thickness, area and materials assignment according to real conditions. Mechanical parameters of GO and EC are indicated in Note S1, ESI,† while disparate CTE values of GO in all temperature intervals are adopted from Kotov group's work.31 90 °C was set as the environmental temperature for strainless models; then thermal stresses and deformations were calculated and visualized under arbitrary thermal conditions.
Fabrication and characterization of 3D-PTA origamis
The EC film was blade cut into specific shapes, followed by drop casting GO dispersion at predesigned regions. After air drying and 12 hours 90 °C vacuum oven annealing, 3D architectures could be immediately obtained upon cooling back to room temperature. 90 °C was selected as the annealing temperature since it could deliver sufficient temperature gradient (ΔT) for 3D-PTA fabrication, yet was not too high to expedite thermal reduction in GO. A NIR light source (Philips BR125 250 W) was utilized as stimulus, whose power density at different distance was measured by a NIR power meter (Linshang LS122A). The emission spectrum of the light source was determined using a spectroscopy CCD detector (Princeton Pixis 100B). Photothermal actuation of the samples was recorded by a digital camera, while an infrared camera (Fluke Ti200) was employed for temperature tracking.
Conflicts of interest
The authors declare no conflict of interest.
Acknowledgements
This work was supported by the Competitive Research Program (NRF-CRP13-2014-02) and NRF Investigatorship (Award No. NRF-NRFI2016-05) under the National Research Foundation, Prime Minister's Office, Singapore. D. Gao acknowledges the research scholarships awarded by Nanyang Technological University, Singapore.
Notes and references
- Y. Zhang, F. Zhang, Z. Yan, Q. Ma, X. Li, Y. Huang and J. A. Rogers, Nat. Rev. Mater., 2017, 2, 17019 CrossRef CAS.
- J. Rogers, Y. Huang, O. G. Schmidt and D. H. Gracias, MRS Bull., 2016, 41, 123–129 CrossRef.
- X. Guo, H. Li, B. Y. Ahn, E. B. Duoss, K. J. Hsia, J. A. Lewis and R. G. Nuzzo, Proc. Natl. Acad. Sci. U. S. A., 2009, 106, 20149–20154 CrossRef CAS PubMed.
- Z. Zhao, J. Wu, X. Mu, H. Chen, H. J. Qi and D. Fang, Sci. Adv., 2017, 3, 1602326 CrossRef PubMed.
- A. S. Gladman, E. A. Matsumoto, R. G. Nuzzo, L. Mahadevan and J. A. Lewis, Nat. Mater., 2016, 15, 413–418 CrossRef PubMed.
- S. Xu, Z. Yan, K. I. Jang, W. Huang, H. R. Fu, J. Kim, Z. Wei, M. Flavin, J. McCracken, R. Wang, A. Badea, Y. Liu, D. Q. Xiao, G. Y. Zhou, J. Lee, H. U. Chung, H. Y. Cheng, W. Ren, A. Banks, X. L. Li, U. Paik, R. G. Nuzzo, Y. G. Huang, Y. H. Zhang and J. A. Rogers, Science, 2015, 347, 154–159 CrossRef CAS PubMed.
- J. Wang, S. Li, D. Gao, J. Xiong and P. S. Lee, NPG Asia Mater., 2019, 11, 71 CrossRef CAS.
- Z. Yan, F. Zhang, F. Liu, M. Han, D. Ou, Y. Liu, Q. Lin, X. Guo, H. Fu, Z. Xie, M. Gao, Y. Huang, J. Kim, Y. Qiu, K. Nan, J. Kim, P. Gutruf, H. Luo, A. Zhao, K. C. Hwang, Y. Huang, Y. Zhang and J. A. Rogers, Sci. Adv., 2016, 2, 1601014 CrossRef PubMed.
- S. Li, D. M. Vogt, D. Rus and R. J. Wood, Proc. Natl. Acad. Sci. U. S. A., 2017, 114, 13132–13137 CrossRef CAS PubMed.
- D. Rus and M. T. Tolley, Nat. Rev. Mater., 2018, 3, 101–112 CrossRef.
- A. Lamoureux, K. Lee, M. Shlian, S. R. Forrest and M. Shtein, Nat. Commun., 2015, 6, 8092 CrossRef PubMed.
- K. Kuribayashi, K. Tsuchiya, Z. You, D. Tomus, M. Umemoto, T. Ito and M. Sasaki, Mater. Sci. Eng., A, 2006, 419, 131–137 CrossRef.
- Y. Hu, Z. Li, T. Lan and W. Chen, Adv. Mater., 2016, 28, 10548–10556 CrossRef CAS PubMed.
- Y. Zhao, L. Song, Z. Zhang and L. Qu, Energy Environ. Sci., 2013, 6, 3520–3536 RSC.
- M. Weng, P. Zhou, L. Chen, L. Zhang, W. Zhang, Z. Huang, C. Liu and S. Fan, Adv. Funct. Mater., 2016, 26, 7244–7253 CrossRef CAS.
- M. Amjadi and M. Sitti, Adv. Sci., 2018, 5, 1800239 CrossRef PubMed.
- J. Deng, J. Li, P. Chen, X. Fang, X. Sun, Y. Jiang, W. Weng, B. Wang and H. Peng, J. Am. Chem. Soc., 2016, 138, 225–230 CrossRef CAS PubMed.
- X. Zhang, Z. Yu, C. Wang, D. Zarrouk, J. W. Seo, J. C. Cheng, A. D. Buchan, K. Takei, Y. Zhao, J. W. Ager, J. Zhang, M. Hettick, M. C. Hersam, A. P. Pisano, R. S. Fearing and A. Javey, Nat. Commun., 2014, 5, 2983 CrossRef PubMed.
- Y. Tai, G. Lubineau and Z. Yang, Adv. Mater., 2016, 28, 4665–4670 CrossRef CAS PubMed.
- Y. Hu, G. Wu, T. Lan, J. Zhao, Y. Liu and W. Chen, Adv. Mater., 2015, 27, 7867–7873 CrossRef CAS PubMed.
- Y. Hu, J. Liu, L. Chang, L. Yang, A. Xu, K. Qi, P. Lu, G. Wu, W. Chen and Y. Wu, Adv. Funct. Mater., 2017, 27, 1704388 CrossRef.
- C. Z. Wu, J. Feng, L. L. Peng, Y. Ni, H. Y. Liang, L. H. He and Y. Xie, J. Mater. Chem., 2011, 21, 18584–18591 RSC.
- D. Kim, H. S. Lee and J. Yoon, Sci. Rep., 2016, 6, 20921 CrossRef CAS PubMed.
- L. Chen, M. Weng, P. Zhou, F. Huang, C. Liu, S. Fan and W. Zhang, Adv. Funct. Mater., 2018, 29, 1806057 CrossRef.
- M. Ji, N. Jiang, J. Chang and J. Sun, Adv. Funct. Mater., 2014, 24, 5412–5419 CrossRef CAS.
- W. Jiang, D. Niu, H. Liu, C. Wang, T. Zhao, L. Yin, Y. Shi, B. Chen, Y. Ding and B. Lu, Adv. Funct. Mater., 2014, 24, 7598–7604 CrossRef CAS.
- J. Mu, C. Hou, H. Wang, Y. Li, Q. Zhang and M. Zhu, Sci. Adv., 2015, 1, 1500533 CrossRef PubMed.
- E. Wang, M. S. Desai and S. W. Lee, Nano Lett., 2013, 13, 2826–2830 CrossRef CAS PubMed.
- H. Cheng, F. Zhao, J. Xue, G. Shi, L. Jiang and L. Qu, ACS Nano, 2016, 10, 9529–9535 CrossRef CAS PubMed.
- L. Chen, M. Weng, P. Zhou, L. Zhang, Z. Huang and W. Zhang, Nanoscale, 2017, 9, 9825–9833 RSC.
- J. Zhu, C. M. Andres, J. Xu, A. Ramamoorthy, T. Tsotsis and N. A. Kotov, ACS Nano, 2012, 6, 8357–8365 CrossRef CAS PubMed.
- W. Bao, F. Miao, Z. Chen, H. Zhang, W. Jang, C. Dames and C. N. Lau, Nat. Nanotechnol., 2009, 4, 562–566 CrossRef CAS PubMed.
- J. Xiong, S. Li, Y. Ye, J. Wang, K. Qian, P. Cui, D. Gao, M. F. Lin, T. Chen and P. S. Lee, Adv. Mater., 2018, 30, 1802803 CrossRef PubMed.
- W. Kang, M. F. Lin, J. Chen and P. S. Lee, Small, 2016, 12, 6370–6377 CrossRef CAS PubMed.
- Z. Gui, H. Zhu, E. Gillette, X. Han, G. W. Rubloff, L. Hu and S. B. Lee, ACS Nano, 2013, 7, 6037–6046 CrossRef CAS PubMed.
-
S. Lampman, Characterization and failure analysis of plastics, ASM International, 2003 Search PubMed.
- K. P. Loh, Q. Bao, G. Eda and M. Chhowalla, Nat. Chem., 2010, 2, 1015–1024 CrossRef CAS PubMed.
- P. Cui, J. Lee, E. Hwang and H. Lee, Chem. Commun., 2011, 47, 12370–12372 RSC.
- A. Buchsteiner, A. Lerf and J. Pieper, J. Phys. Chem. B, 2006, 110, 22328–22338 CrossRef CAS PubMed.
- R. R. Kohlmeyer and J. Chen, Angew. Chem., Int. Ed., 2013, 52, 9234–9237 CrossRef CAS PubMed.
- T. Yoshino, M. Kondo, J. Mamiya, M. Kinoshita, Y. Yu and T. Ikeda, Adv. Mater., 2010, 22, 1361–1363 CrossRef CAS PubMed.
- C. L. van Oosten, C. W. Bastiaansen and D. J. Broer, Nat. Mater., 2009, 8, 677–682 CrossRef CAS PubMed.
- X. Sun, W. Wang, L. Qiu, W. Guo, Y. Yu and H. Peng, Angew. Chem., Int. Ed., 2012, 51, 8520–8524 CrossRef CAS PubMed.
- T. Lan, Y. Hu, G. Wu, X. Tao and W. Chen, J. Mater. Chem. C, 2015, 3, 1888–1892 RSC.
- K. Bertoldi, V. Vitelli, J. Christensen and M. van Hecke, Nat. Rev. Mater., 2017, 2, 17066 CrossRef CAS.
- Y. P. Liu and H. Hu, Sci. Res. Essays, 2010, 5, 1052–1063 Search PubMed.
- M. J. Harrington, K. Razghandi, F. Ditsch, L. Guiducci, M. Rueggeberg, J. W. Dunlop, P. Fratzl, C. Neinhuis and I. Burgert, Nat. Commun., 2011, 2, 337 CrossRef PubMed.
- D. C. Marcano, D. V. Kosynkin, J. M. Berlin, A. Sinitskii, Z. Sun, A. Slesarev, L. B. Alemany, W. Lu and J. M. Tour, ACS Nano, 2010, 4, 4806–4814 CrossRef CAS PubMed.
Footnotes |
† Electronic supplementary information (ESI) available. See DOI: 10.1039/c9nh00719a |
‡ D. Gao and M.-F. Lin contributed equally to this work. |
§ Present affiliation: Department of Materials Engineering, Ming Chi University of Technology, New Taipei City 24301, Taiwan. |
|
This journal is © The Royal Society of Chemistry 2020 |