DOI:
10.1039/C9NA00488B
(Paper)
Nanoscale Adv., 2020,
2, 679-690
The SALSAC approach: comparing the reactivity of solvent-dispersed nanoparticles with nanoparticulate surfaces†
Received
8th August 2019
, Accepted 12th December 2019
First published on 12th December 2019
Abstract
We demonstrate that the ‘surface-as-ligand, surface-as-complex’ (SALSAC) approach that we have established for annealed nanoparticulate TiO2 surfaces can be successfully applied to nanoparticles (NPs) dispersed in solution. Commercial TiO2 NPs have been activated by initial treatment with aqueous HNO3 followed by dispersion in water and heating under microwave conditions. We have functionalized the activated NPs with anchoring ligands 1–4; 1–3 contain one or two phosphonic acid anchoring groups and 4 has two carboxylic acid anchors; ligands 1, 2 and 4 contain 6,6′-dimethyl-2,2′-bipyridine (Me2bpy) metal binding domains and 3 contains a 2,2′:6′,2′′-terpyridine (tpy) unit. Ligand functionalization of the activated NPs has been validated using infrared and 1H NMR spectroscopies, and thermogravimetric analysis. NPs functionalized with 1, 2 and 4 react with [Cu(MeCN)4][PF6] and those with 3 react with FeCl2·4H2O; metal binding has been investigated using solid-state absorption spectroscopy and scanning electron microscopy (SEM). Competitive binding of ligands 1–4 to TiO2 NPs has been investigated and shows preferential binding of phosphonic acid over carboxylic acid anchors. For the phosphonic acids, the binding orders are 3 > 1 > 2 which is rationalized in terms of relative pKa values (phosphonic acid and [HMe2bpy]+ or [Htpy]+) and the number of anchoring groups in the ligands. Ligand exchange between ligand-functionalized NPs and homoleptic metal complexes gives NPs functionalized with heteroleptic copper(I) or iron(II) complexes.
Introduction
Numerous methodologies have been developed for the synthesis of hierarchical structures1–4 at interfaces, including self-assembled monolayers,5–7 self-organization and self-assembly8,9 through van der Waals forces10–12 supramolecular chemistry,13 templating,14 sol–gel deposition techniques,15 pyrolysis4 and dye-stamping.16 In most cases the methods are either highly specific to the target structure, are dependent upon the preparation of complex soluble or volatile covalent structures or have significant elements of serendipity in the outcome.17
In the context of our research into dye-sensitized solar cells (DSCs), we developed a methodology18–20 which we have termed ‘surfaces-as-ligands, surfaces-as-complexes’ (SALSAC). This extends ‘simple’ self-assembly and self-organization at surfaces to the stepwise and hierarchical building up of new pre-defined and easily tailored architectures. Our inspiration and motivation lie in an appreciation of the exquisite complexity of biological systems and at the same time a desire to utilize self-assembly and self-organization strategies to achieve similar levels of complexity in synthetic systems. An added value of this approach is that the increasing complexity of the architectures can lead to emergent properties21 not found in the individual components. The concept of this paper relates to the transferability of modification reactions between extended surfaces and nanoparticulate materials.
Although we developed the SALSAC approach for the assembly of heteroleptic copper(I) dyes on the surface of semiconductor nanostructured surfaces for use in DSCs,20 it is clear that the experimentally simple approach of sequentially reacting a surface with components leading to a hierarchical assembly has wider applications. In its mature form, SALSAC has been developed into a powerful atom-efficient methodology allowing the preparation of multiply and specifically functionalized surfaces through step-wise assembly of structures incorporating anchoring ligands, metal ions and ancillary ligands.20 The SALSAC approach is illustrated in Fig. 1: a nanoparticulate surface is modified with a compound (Lanchor) which combines an anchoring group specific to the surface (e.g. carboxylic acid, carboxylate, phosphonic acid or phosphonate for a metal oxide) and a metal-binding domain such as 2,2′-bipyridine (bpy) or 2,2′:6′,2′′-terpyridine (tpy). Subsequent reaction with a metal ion (e.g. copper(I) in the case of DSC applications20) and concurrent or consecutive reaction with ancillary ligands (Lancillary) gives a surface functionalized with a heteroleptic metal complex containing both Lanchor and Lancillary ligands. An alternative strategy is to introduce metal ion and Lancillary by ligand exchange from a homoleptic complex.
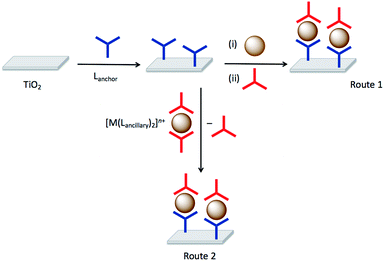 |
| Fig. 1 Surface modification (e.g. nanoparticulate TiO2) with a single anchor, single metal ion and an ancillary ligand (route 1), or with a single anchor followed by ligand exchange using a homoleptic metal complex (route 2). | |
Although we have focused on the addition of a single type of ancillary ligand in optimizing DSCs, we have also demonstrated that surfaces can be modified by sequential addition of two different ancillary ligands.22 Further development of this approach has the potential to introduce secondary surface sites which act purely to spatially separate the primary surface sites. In DSC applications, this translates to the introduction of co-adsorbants which can also bind to the metal oxide surface, typically through carboxylic acid or carboxylate, to inhibit molecular-dye aggregation.23 Spatial separation may also be important in preventing metal-binding domains of surface-attached anchoring ligands from binding the same metal ion, generating surface-bound homoleptic complexes rather than the desired heteroleptic species.24
Although numerous studies have described the attachment of anchor-functionalized complexes to surfaces, few studies relate to initial ligand functionalization of a surface through an anchor.25 While ligand functionalized surfaces have been subsequently metallated,26,27 to the best of our knowledge, there has been no systematic investigation of the SALSAC methodology and combined use to introduce functionality through the ancillary ligand other than our own work in the context of copper(I) DSCs. We now explore how the principles established for annealed nanoparticulate TiO2 surfaces (i.e. working electrodes in DSCs) can be extended to nanoparticles (NPs) of the same material dispersed in fluid phase. The fabrication and applications of functionalized metal oxide NPs is well established,28 but hierarchical assembly strategies are less well explored. It should be noted that when an anchoring ligand with an acidic functionality such as –CO2H or –PO3H2 is introduced, the protonation state and binding mode of the ligand is typically not well-defined, although the properties of the assembly are critically dependent on the protonation state.29–31 The functionalization of metal chalocogenide nanoparticles with carboxylic and phosphoric acids has attracted much recent attention and a few reports of selectivity and exchange of surface-bound ligands have been published.32–41
Experimental
General
Details of instrumentation are given in the ESI.†
The complex [Cu(MeCN)4][PF6] was prepared by the method of Kubas.42 4-(4-Bromophenyl)-6,6′-dimethyl-2,2′-bipyridine,181,443,304,435,446,458,46 [Fe(8)2][PF6]2,47 and [Cu(5)2][PF6]44 were prepared according to literature and their spectroscopic data matched those previously reported.
TiO2 nanoparticles (AEROXIDE TiO2 P25) were purchased from Evonik Industries. The spherical NPs have an average radius of 10.5 nm,48 leading to a surface area-to-volume ratio of 28%. In the Experimental section, the number of equivalents of NPs are defined as 0.28× the total number of TiO2 formula equivalents in the mass given, i.e. the effective surface concentration of TiO2.
Compound 2a
Solid 4-(4-bromophenyl)-6,6′-dimethyl-2,2′-bipyridine (340 mg, 1.00 mmol, 1 eq.), Cs2CO3 (1.629 g, 5 mmol, 5 eq.) and [Pd(PPh3)4] (57.8 mg, 0.05 mmol, 0.05 eq.) were placed in a 20 mL microwave vial under N2. Diethyl phosphonate (552 mg, 511 μL, 4 mmol, 4 eq.) and 15 mL dry THF were added. The mixture was degassed by bubbling N2 for 5–10 min and then the vial was capped. The reaction mixture was heated in the microwave reactor for 5 h at 130 °C and then cooled to room temperature, filtered through Celite and evaporated to dryness. The resulting yellow oil was dissolved in MeCN and the product isolated as pale-yellow crystals which were collected by filtration, washed with cold Et2O and dried under vacuum (200 mg). The crude product was used in the next step without further purification (see text).
Compound 7
5 (582 mg, 1.18 mmol, 1.0 eq.), Cs2CO3 (2.31 g, 7.08 mmol, 6.0 eq.) and [Pd(PPh3)4] (136 mg, 0.118 mmol, 10 mol%) were added to a microwave vial under N2. 3,5-Dimethyl-4-(4,4,5,5-tetramethyl-1,3,2-dioxaborolan-2-yl)isoxazole (1.05 g, 4.72 mmol, 4.0 eq.) and anhydrous toluene (20 mL) were added to another vial and N2 was bubbled through the solution for 15 min. The contents of the two vials were combined into one microwave vial and N2 was bubbled through the mixture for 10 min. The vial was sealed and the reaction mixture was heated to 110 °C for 4 h in a microwave reactor, after which time it was allowed to cool to room temperature. Then H2O (40 mL) was added and the reaction mixture was extracted with CH2Cl2 (3 × 50 mL) and dried over anhydrous MgSO4. The solvent was removed under reduced pressure to yield an off-white solid. The residue was dissolved in hot CHCl3 (1 mL), and the product was precipitated by addition of EtOH (10 mL). The precipitate was washed with EtOH (50 mL) and acetone (50 mL) to yield 7 as a beige solid (205 mg, 1.18 mmol, 33%). 1H NMR (500 MHz, CDCl3) δ/ppm: 8.55 (d, J = 1.6, 2H, HA3), 7.85 (dd, J = 8.3, J = 1.7, 4H, HB2), 7.44 (d, J = 1.6, 2H, HA5), 7.40 (dd, J = 8.2, J = 1.7, 4H, HB3), 2.74 (s, 6H, HMeA), 2.47 (s, 6H, HMeC), 2.33 (s, 6H, HMeC). 13C{1H} NMR (126 MHz, CDCl3) δ/ppm: 165.4 (CC3/C5), 158.8 (CC3/C5), 158.7 (CA6), 156.7 (CA2), 149.0 (CA4), 138.1 (CB1), 131.2 (CB4), 129.8 (CB3), 127.8 (CB2), 121.2 (CA5), 116.7 (CA3), 116.3 (CC4), 25.0 (CMeA), 11.8 (CMeC), 11.1 (CMeC). ESI-MS: m/z 527.19 [M + H]+ (calc. 527.24). HR ESI-MS: m/z 527.2440 [M + H]+ (calc. 527.2440).
[Cu(6)2][PF6]
Compound 6 (15 mg, 0.053 mmol, 2 eq.) was dissolved in acetone (0.5 mL) and [Cu(MeCN)4][PF6] (9.9 mg, 0.027 mmol, 1.0 eq.) was added to the solution. The solution was stirred for 10 min and then Et2O (3 mL) was added. The precipitate that formed was removed by centrifugation to yield [Cu(6)2][PF6] as a dark-red solid (17 mg, 0.02 mmol, 83%). No further purification was required. 1H NMR (500 MHz, acetone-d6) δ/ppm: 8.87 (dd, J = 5.1, J = 1.2, 2H, HA6), 8.21 (td, J = 8.0, J = 1.6, 2H, HA3), 8.16 (dd, J = 7.9, J = 1.6, 2H, HA4), 8.02 (s, 2H, HC1), 7.77 (m, 2H, HA5), 7.75–7.72 (overlapping m, 4H, HB4+C5), 7.67 (m, 2H, HC3), 7.64 (m, 2H, HB3), 7.54 (m, 2H, HB5), 7.64 (m, 2H, HC6), 7.41 (d, J = 8.4, 2H, HC4), 7.36 (ddd, J = 8.1, J = 6.8, J = 1.2, 2H, HC7), 7.24 (d, J = 8.1, 2H, HC8). 13C{1H} NMR (126 MHz, acetone-d6) δ/ppm: 157.9 (CB6), 153.2, (CA2) 152.9 (CB2), 149.6 (CA6), 138.8 (CA4+B4), 137.7 (CC2), 134.1 (CC4a), 133.1 (CC8a), 128.8 (CC8), 128.4 (CC4/C5), 128.3 (CC4/C5), 127.9 (CC1/C6), 127.8 (CC1/C6), 127.3 (CA5+C7), 125.8 (CB5), 125.3 (CC3), 123.3 (CA3), 120.7 (CB3). UV-vis (CH2Cl2, 2.02 × 10−5 mol dm−3), λ/nm (ε/dm3 mol−1 cm−1): 235 (103
500), 260 (64
700), 305 (36
600), 430 (5500), 554 (2300). ESI-MS: m/z 627.2 [M − PF6]+ (calc. 627.2). Found C 61.68, H 3.95, N 7.17; C40H28CuF6N4P requires C 62.14, H 3.65, N 7.25.
[Cu(7)2][PF6]
Compound 7 (15 mg, 0.038 mmol, 2 eq.) was dissolved in acetone (0.5 mL) and [Cu(MeCN)4][PF6] (5.31 mg, 0.019 mmol, 1.0 eq.) were added to the solution. The solution was stirred for 10 min and then Et2O (3 mL) was added. The precipitate that formed was separated by centrifuge to yield [Cu(7)2][PF6] as a red solid (14 mg, 0.010 mmol, 78%). No further purification was required. 1H NMR (500 MHz, acetone-d6) δ/ppm: 9.15 (d, J = 1.6, 4H, HA3), 8.20 (dd, J = 8.1, J = 1.7, 8H, HB2), 8.11 (d, J = 1.5, 4H, HA5), 7.66 (d, J = 8.1, J = 1.7, 8H, HB3), 2.52 (s, 12H, HMeA), 2.48 (s, 12H, HMeC), 2.31 (s, 12H, HMeC). 13C{1H} NMR (126 MHz, acetone-d6) δ/ppm: 165.4 (CC3/C5), 158.9 (CC3/C5), 158.7 (CA6), 153.6 (CA2), 150.7 (CA4), 137.0 (CB1), 133.3 (CB4), 130.8 (CB3), 128.8 (CB2), 124.5 (CA5), 118.8 (CA3), 116.5 (CC4), 25.5 (CMeA), 11.7 (CMeC), 10.9 (CMeC). UV-vis (CH2Cl2, 3.33 × 10−5 mol dm−3) λ/nm (ε/dm3 mol−1 cm−1): 285 (81
600), 325 (52
200), 495 (12
800). ESI-MS: m/z 1115.4 [M − PF6]+ (calc. 1115.4). HR ESI-MS: m/z 1115.4013 [M − PF6]+ (calc. 1115.4028). Satisfactory elemental analysis was not obtained.
Functionalization of commercial (non-activated nanoparticles): Procedure A
Ligand 1 (10 mg, 1 eq.) was dissolved in dry DMSO (10 mL). Commercial TiO2 NPs (48.2 mg, 8.4 TiO2 eq.) were added to the solution and dispersed with sonication for 10 min. The mixture was stirred at room temperature (ca. 295 K) for 48 h. Then the particles were separated by centrifugation (10 min. 9000 rpm) and washed with DMSO (3 × 5 mL) and EtOH (1 × 5 mL). The NPs were dried under high vacuum and the white powder was stored under N2 in a sealed vial.
Activation of commercial nanoparticles
Commercial TiO2 NPs (1 g) were dispersed in dilute aqueous HNO3 (15 mL, 13.6%) then the mixture was sonicated for 15 min and stirred for 30 min. The NPs were separated by centrifugation and washed once with water (15 mL). Afterwards the NPs were dispersed through sonication (10 min) in water (20 mL) and stirred overnight. The NPs were separated with centrifugation, washed twice with water (2 × 20 mL) and then dried over high vacuum. The activated NPs (950 mg) were stored in a sealed vial under N2.
Functionalization of activated nanoparticles (Procedure B)
Ligand 1 (3.73 mg, 1 eq.) was placed in a microwave vial and milliQ water (13 mL) was added. The mixture was dispersed by sonication for 1 min. Activated TiO2 NPs (60.0 mg, 28 TiO2 eq.) were added to the solution and dispersed with sonication for 10 min. The microwave vial was sealed and the reaction mixture was heated to 130 °C for 3 h in the microwave reactor. The reaction mixture was allowed to cool to room temperature. The functionalized NPs were separated from the solvent by centrifugation (10 min, 9000 rpm). Then the functionalized NPs were dried under high vacuum yielding a slightly yellow powder.
Analogous procedures were used to functionalize activated NPs with ligands 2, 3 or 4 starting with 2 (2.56 mg, 1 eq.), 3 (2.92 mg, 1 eq.), 4 (3.19 mg, 1 eq.) and activated TiO2 NPs (60.0 mg, 28 TiO2 eq.). For 4, the functionalized NPs were washed with DMSO (3 × 10 mL) and EtOH (3 × 10 mL); see discussion in manuscript. The functionalized NPs were dried under high vacuum and the white powder was stored under N2 in a sealed vial.
Functionalization of activated nanoparticles: competition between anchoring ligands (Procedure C)
Ligands 1 (3.73 mg, 1 eq.) and 2 (2.56 mg, 1 eq.) were placed in a microwave vial and milliQ water (13 mL) was added. The mixture was dispersed by sonication for 1 min. Activated TiO2 NPs (60.0 mg, 28 TiO2 eq.) were added to the solution and dispersed with sonication for 10 min. The microwave vial was sealed and the reaction mixture was heated to 130 °C for 3 h in a microwave reactor. The reaction mixture was allowed to cool to room temperature. The water was then removed under high vacuum and then some of the residue was dispersed in DMSO-d6 in an NMR tube.
An analogous procedure was used with combinations of ligands 1 (3.73 mg, 1 eq.) and 3 (2.92 mg, 1 eq.), or ligands 1 (3.73 mg, 1 eq.) and 4 (3.19 mg, 1 eq.) with activated TiO2 NPs (60.0 mg, 28 TiO2 eq.).
Functionalization of activated nanoparticles: competition between anchoring ligands (Procedure D)
Ligand 4 (3.19 mg, 1 eq.) was placed in a microwave vial and milliQ water (13 mL) was added. The mixture was dispersed by sonication for 1 min. Activated TiO2 NPs (60.0 mg, 28 TiO2 eq.) were added to the solution and dispersed with sonication for 10 min. The microwave vial was sealed and the reaction mixture was heated to 130 °C for 3 h in the microwave reactor. The reaction mixture was allowed to cool to room temperature. The water was then removed under high vacuum and then some of the residue was dispersed in DMSO-d6 in an NMR tube and the 1H NMR spectrum was recorded. The remaining residue was washed by dispersing it in DMSO (3 × 10 mL) and EtOH (3 × 10 mL). The washed residue was collected by centrifugation and was dried under high vacuum. Then some of the residue was dispersed in DMSO-d6. The NMR spectrum revealed that no excess free ligand was left on the functionalized NPs. Ligand 1 (2.49 mg, 1 eq.) was placed in a microwave vial and milliQ water (13 mL) was added. The mixture was dispersed by sonication for 1 min. The previously obtained NPs functionalized with 4 (40 mg) were added to the solution and dispersed with sonication for 10 minutes. The microwave vial was sealed and the reaction mixture was heated to 130 °C for 3 h in the microwave reactor. The reaction mixture was allowed to cool to room temperature. The water was then removed under high vacuum to yield a residue, a portion of which was dispersed in DMSO-d6 in an NMR tube.
Complexation of nanoparticles functionalized with anchored ligands
Stock solutions of [Cu(MeCN)4][PF6] (5.59 mg, 10 mL, 1.5 mM in acetone) and FeCl2·4H2O (2.88 mg, 10 mL, 1.5 mM in acetone) were prepared.
Activated NPs functionalized with ligands (10 mg) (Procedures B or C above) were dispersed by sonication (15 s) in water (1.35 mL) and the stock solution of [Cu(MeCN)4][PF6] in the case of 1, 2 or 4 (0.15 mL) or of FeCl2·4H2O in the case of 3 (0.15 mL) was added. The solutions in the sample vials were stirred for 72 h. The coloured NPs were separated by centrifugation (10 min, 13
200 rpm), then washed with acetone (3 × 1 mL) and dried under high vacuum.
SALSAC assembly of heteroleptic copper(I) complexes on activated nanoparticles
Stock solutions of [Cu(5)2][PF6], [Cu(6)2][PF6] and [Cu(7)2][PF6] (1.5 mM in acetone) were prepared. NPs functionalized with 1 (10 mg, see Procedure B) were dispersed in water (1.35 mL) by sonication (15 s) and then a portion (0.15 mL) of the stock solution of [Cu(5)2][PF6] was added. The mixture was stirred for 72 h. The orange-red NPs were separated from the supernatant liquid by centrifugation (10 min, 13
200 rpm), then washed with acetone (3 × 1 mL) and dried under high vacuum. The procedure was repeated using [Cu(6)2][PF6] or [Cu(7)2][PF6].
SALSAC assembly of heteroleptic iron(II) complexes on activated nanoparticles
A stock solution of [Fe(8)2][PF6]2 (1.5 mM in acetone) was prepared. NPs functionalized with 3 (10 mg, see Procedure B) were dispersed in water (1.35 mL) by sonication (15 s) and then a portion (0.15 mL) of the stock solution of [Fe(8)2][PF6]2 was added. The mixture was stirred for 72 h. The purple NPs were separated from the supernatant liquid by centrifugation (10 min, 13
200 rpm), then washed with acetone (3 × 1 mL) and dried under high vacuum.
Results and discussion
Choice of anchoring ligands
Based on our previous experience of functionalizing TiO2 surfaces,19,20,24 anchoring ligands 1 and 4 (Scheme 1) were selected for the present investigation to provide a comparison of carboxylic versus phosphonic acid anchors. The DSC community consider that phosphonic acid anchors give more stable attachment to TiO2 surfaces than carboxylic acids.49 Both 1 and 4 contain a bpy metal-binding domain for coordination to copper(I).19 The presence of the 6,6′-dimethyl substituents stabilizes a bis(diimine)copper(I) complex against oxidation by inhibiting a geometrical change from tetrahedral (favoured by Cu+) to square planar (favoured by Cu2+).50,51 Ligand 2 was designed to allow a comparison of mono- versus bis(phosphonic acid) anchors (2versus1) bearing the same bpy metal-binding domains. Both 2 and 3 carry one phosphonic acid group, but differ in the metal-binding unit; bpy and 2,2′:6′,2′′-terpyridine (tpy) can be addressed by copper(I) and iron(II), respectively. Each of ligands 1–4 contains a phenylene spacer between the anchoring group and the metal-binding units.
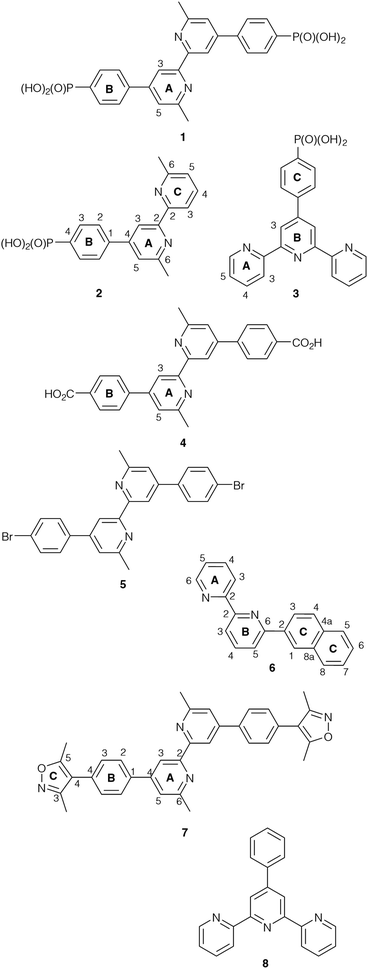 |
| Scheme 1 Structures of compounds 1–8. Atom numbering schemes are given where required for NMR spectroscopic assignments. | |
Compound 2 was prepared as described in the Experimental section. The base peak in the electrospray mass spectrum at m/z 339.06 (Fig. S1†) was assigned to [M − H]−. The 1H and 13C NMR spectra of 2 (Fig. S2 and S3†) were assigned by COSY, NOESY, HMQC and HMBC methods (Fig. S4–S6†) and were consistent with the structure shown in Scheme 1.
Nanoparticle activation and general procedure for functionalization
Our initial attempts to replicate TiO2 surface-functionalization using commercial NPs (AEROXIDE TiO2 P25) suspended in DMSO in place of screen-printed and annealed (commercial or in-house fabricated) surfaces following previously published protocols20,52 met with variable success. A significant observation came from DOSY experiments on a DMSO-d6 solution of 1 and a DMSO-d6 suspension of dried commercial NPs after treatment with 1 (Procedure A in the Experimental section). Fig. 2 shows a comparison of the 1H NMR spectra of the two samples. Apart from small changes (<0.1 ppm) in the chemical shifts of signals, the spectra are essentially identical and the signals remain sharp. This strongly suggests that free ligand 1 is present in the solution containing dispersed NPs. To unambiguously confirm this proposal, PFGSE spectra of 1 before and after exposure to commercial NPs were recorded. The proton signal at δ 8.525 ppm was selected to determine the diffusion coefficient by a two-parameter fit to the DOSY intensities. For 1 in DMSO-d6, a diffusion coefficient of 1.355 × 10−10 m2 s−1 was determined (Fig. S7a†), and for the solution containing the dispersed NPs the diffusion coefficient was 1.326 × 10−10 m2 s−1 (Fig. S7b†), identical to the previous value within experimental error. These data are consistent with either some or all of 1 remaining non-anchored during attempts to functionalize the NPs using Procedure A detailed in the Experimental section. This illustrates a general problem with non-activated NPs, that simple washing does not suffice to remove surface-adsorbed or aggregated ligand from NPs with anchored ligand.
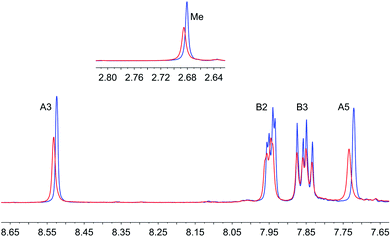 |
| Fig. 2
1H NMR spectrum (500 MHz, DMSO-d6, aromatic region with methyl region inset) of 1 (blue) and of the NPs after treatment with 1 following Procedure A in Experimental section (red). Chemical shifts in δ/ppm. | |
We therefore decided to activate the commercial TiO2 NPs prior to functionalization with the ligand. Literature activation procedures53 include treatment with HNO3 (ref. 54) and ultrasound sonication for to optimize particle dispersion.55 Initial tests revealed that replacing DMSO by water was advantageous for NP functionalization, and that reducing the amount of anchoring ligand with respect to the quantity of NPs was also beneficial. We therefore modified the procedure by an initial treatment of the commercial NPs with aqueous HNO3 (see Experimental section). Following this, the activated NPs were added to an aqueous suspension of 1, 2, 3 or 4 and dispersed with sonication before the suspension was heated at 130 °C for 3 h under microwave conditions before being separated from the solvent by centrifugation (see Procedure B in the Experimental section). Fig. 3 compares part of the FT-IR spectra of the activated NPs, free ligand 1 and activated NPs functionalized with 1; the full spectra are given in Fig. S8.† The spectrum of the functionalized NPs exhibits bands at 1629, 1601, 1570 and 1545 cm−1. While the bands appear to be characteristic of free ligand 1, closer inspection of Fig. 3 shows differences with the spectroscopic signature of pristine 1 which has absorptions at 1645, 1624, 1598 and 1545 cm−1. Significantly, this pattern of absorptions is retained in a mixture of commercial NPs (non-activated) and 1 (1647, 1624, 1600 and 1543 cm−1, Fig. 3). Thus, the IR spectroscopic data provide evidence that ligand 1 is anchored to the TiO2. A comparison of the IR spectra of ligand 3 and NPs functionalized with 3 is shown in Fig. S9.† Thermogravimetric analysis (TGA) of the activated NPs and those functionalized with 1 was carried out, and the results are displayed in Fig. 4. Both samples show a weight loss of about 2% in two steps (isotherm maxima <120 °C and <330 °C) attributed to the loss of physisorbed and chemisorbed water, respectively. The non-functionalized NPs undergo no further change in mass, even after being heated at 900 °C for 30 minutes. In contrast, the NPs functionalized with 1 exhibit a further 2% weight loss above ca. 430 °C ascribed to decomposition of the ligand. The profile of the TGA curve above 430 °C (red curve in Fig. 4) is similar to that of the thermal decomposition of 1 (Fig. S10†). Three measurements were made for the functionalized NPs to confirm reproducibility of the data.
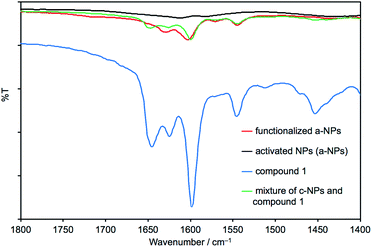 |
| Fig. 3 Solid-state IR spectra of activated NPs (a-NPs), pristine 1, a-NPs functionalized with 1 following Procedure B in the Experimental section, and a mixture of commercial NPs (c-NPs) and 1. The full IR spectra are presented in Fig. S8.† | |
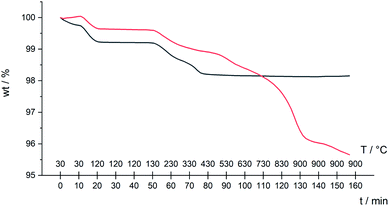 |
| Fig. 4 TGA curves for activated NPs only (black line), and activated NPs functionalized with 1 following Procedure B in the Experimental section (red line). | |
Further evidence for NP functionalization came from a comparison of the 1H NMR spectra of the pristine ligands 1, 2 or 3 in DMSO-d6 with those of suspended NPs functionalized with 1, 2 or 3 (Fig. 5, S11 and S12†). The functionalized NPs were prepared according to Procedure B in the Experimental section. After centrifugation and drying, the functionalized NPs were not washed. The ligand anchored to the NP is NMR silent, and Fig. 5b confirms that all of ligand 1 (shown to be present in the IR study of the same material) is anchored to the TiO2 surface. In the case of ligand 4, the bulk residue showed a signal due to free 4 and the material had to be thoroughly washed with DMSO and EtOH to completely remove traces of free ligand (Fig. S13†). This indicates that the carboxylic acid anchoring ligand 4 adsorbed less efficiently to the surface of the TiO2 NPs than the phosphonic acids 1, 2 and 3. We return to this later.
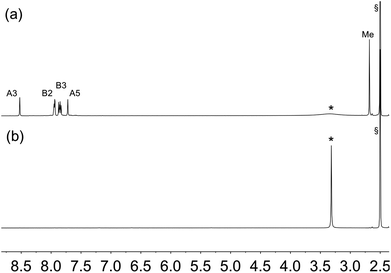 |
| Fig. 5
1H NMR (500 MHz, DMSO-d6, 298 K) of (a) ligand 1 and (b) suspended residue after activated TiO2 NPs had been after activated TiO2 NPs were functionalized with 1 following Procedure B in Experimental section, separated by centrifugation and dried. See Scheme 1 for atom labelling. Chemical shifts in δ/ppm. § = residual DMSO-d5. * = water. | |
To confirm that the functionalized NPs bear anchored ligands capable of coordination to metal ions, NPs functionalized as described above with 1, 2, 3 or 4 were dispersed in water by sonication and an acetone solution of [Cu(MeCN)4][PF6] (for 1, 2 and 4) or FeCl2·4H2O (for 3) was added. Colour changes were observed after about five minutes and the intensity of colour increased over a period of an hour (Fig. 6 and S14†). The orange and purple colours are, respectively, characteristic of [Cu(diimine)2]+ and [Fe(tpy)2]2+ chromophores, and imply that addition of the metal ion results in coordination by two adjacent ligands on a single NP or two ligands on adjacent NPs. Solid-state absorption spectra of the samples (Fig. 6c) exhibit metal-to-ligand charge transfer (MLCT) bands at 489 and 579 nm which, along with the band profiles, are typical of [Cu(diimine)2]+ and [Fe(tpy)2]2+ chromophores, respectively. Scanning electron microscopy (SEM) was used to image the TiO2 NPs before (Fig. 7a) and after functionalization with 1 and [Cu(MeCN)4][PF6] (Fig. 7b). The ‘halo’ around each NP in Fig. 7b is interpreted as the sheath of organic ligand 1. The dimensional relationship between the TiO2 NP and the ‘halo’ supports this proposal.
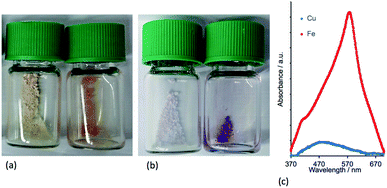 |
| Fig. 6 Photographs of (a) activated TiO2 NPs functionalized with 1 (left) and after treatment with [Cu(MeCN)4][PF6] (right), and (b) activated TiO2 NPs functionalized with 3 (left) and after treatment with FeCl2·4H2O (right). (c) Solid-state absorption spectra of the NPs functionalized with 1 or 3 and treated with [Cu(MeCN)4][PF6] (blue curve) and FeCl2·4H2O (red). | |
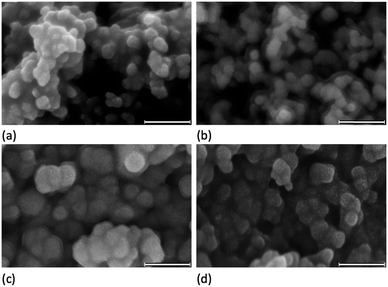 |
| Fig. 7 SEM images of (a) activated TiO2 NPs, and (b) activated TiO2 NPs functionalized with 1 and treated with [Cu(MeCN)4][PF6]. Activated TiO2 NPs functionalized with 1 and after treatment with (c) [Cu(5)2][PF6] or (d) [Cu(7)2][PF6]. Each scale bar = 100 nm. | |
The ligand-functionalized NPs can be stored as dry solids under N2 for periods of months without loss of activity.
Competition between different pairs of anchors on NPs
In applying the SALSAC approach to DSCs, we have demonstrated that devices incorporating dyes with phosphonic acid anchors outperform those with analogous dyes with carboxylic anchors.56,57 Similarly, Grätzel and coworkers have observed strong surface adhesion of ruthenium(II) dyes bearing phosphonic acid anchoring groups.58 Despite this latter finding being reported as early as 1995, the DSC community continues to favour the use of carboxylic acid anchoring units.
We decided to investigate the competitive binding to TiO2 NPs of pairs of ligands 1 and 3, 1 and 4, and 1 and 2 to gain insight into preferential binding of phosphonic and carboxylic acid anchors, and competition between spatially different ligands bearing phosphonic acids. Equimolar amounts of each pair of ligands (1 and 3, 1 and 4, or 1 and 2) were added to water and then TiO2 NPs, activated as described above were added and dispersed with sonication (see Procedure C in the Experimental section). Each mixture was heated to 130 °C for 3 hours in a microwave reactor. After cooling and removal of water in vacuo, part of the residue was dispersed in DMSO-d6 in an NMR tube and 1H NMR spectra of the suspended residues were then recorded. Once again, ligands anchored to the NP surface will not be observed. Fig. 8 displays the 1H NMR spectra of pristine ligands 1, 3 (Fig. 8a and b) and the suspended residue after activated NPs had been treated with a 1
:
1 molar mixture of 1 and 3 (Fig. 8c). Signals in Fig. 8c are attributed to ligands that are not bound to the surface. Fig. 8c shows both free ligands 1 and 3 in solution, and the small changes in chemical shifts on going from Fig. 8a or 8b to 8c are consistent with those described in the previous section. The molar ratio of ligands 1
:
3 in the solution investigated in Fig. 8c is 2
:
1 based on the relative integrals of the signals for protons HA3 and HA5 in each ligand (see Scheme 1 and Fig. 8c). This indicates that the TiO2 NPs preferentially bind 3. Three portions of the washed and dried functionalized NPs (shown to contain no free ligand, Fig. 8d) were dispersed in water by sonication and an acetone solution of [Cu(MeCN)4][PF6] was added to one portion, an acetone solution of both [Cu(MeCN)4][PF6] and FeCl2·4H2O to the second, and an acetone solution of FeCl2·4H2O to the third. Fig. 9a shows the visual difference between the samples, but definitive evidence that both 1 and 3 are bound to the surface comes from a comparison of the IR spectra (Fig. 9b) of NPs functionalized with 1, with 3 or after activated TiO2 NPs were functionalized with a 1
:
1 mixture of 1 and 3 following Procedure C in the Experimental section. The IR spectrum of the latter exhibits diagnostic absorptions of both ligands.
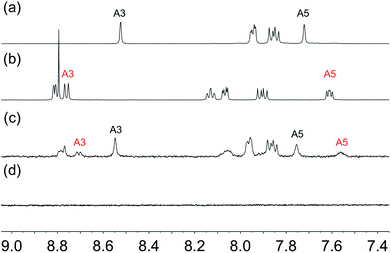 |
| Fig. 8 Aromatic regions of the 1H NMR (500 MHz, DMSO-d6, 298 K) of (a) ligand 1, (b) ligand 3, (c) suspended residue after activated TiO2 NPs were functionalized with 1 and 3 following Procedure C in the Experimental section, (d) residue after washing. See Scheme 1 for atom labelling. Chemical shifts in δ/ppm. | |
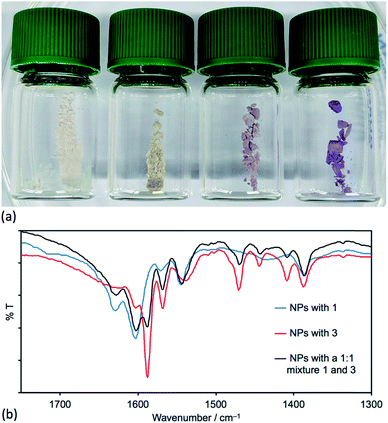 |
| Fig. 9 (a) Photographs of (left to right) activated TiO2 NPs after functionalized with a 1 : 1 mixture of 1 and 3, after one portion has been further treated with [Cu(MeCN)4][PF6], after another portion has been further treated with [Cu(MeCN)4][PF6] and FeCl2·4H2O, and after a final portion has been further treated with FeCl2·4H2O. (b) Solid-state FT-IR spectra of NPs functionalized with ligand 1 only, with 3 only, and activated TiO2 NPs functionalized with 1 and 3 following Procedure C in the Experimental section. | |
Next, we investigated the functionalization of TiO2 NPs with a 1
:
1 molar mixture of 1 and 2. Both ligands contain a 6,6′-Me2bpy unit, but ligand 1 contains two phosphonic acid groups while 2 contains one (Scheme 2). In the 1H NMR spectrum of the suspension of functionalized NPs in DMSO-d6 (Fig. S15c†) the ratio of integrals of signals for protons HA3 (or of HA5) of each ligand in solution is ca. 1
:
1. This is consistent with there being approximately twice as much 2 as 1 remaining in solution after activated TiO2 NPs have been treated with a 1
:
1 mixture of 1 and 2. Thus, 1 is preferentially bound to the surface.
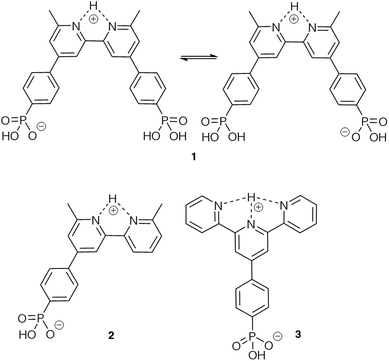 |
| Scheme 2 Zwitterionic structures of ligands 1–3. | |
In order to rationalize the results of the competition experiments, it is pertinent to note that we have previously demonstrated that in DMSO-d6, ligand 1 exists as a zwitterion.31 This is consistent with known values of pKa = 4.4 for [Hbpy]+,59 and of pKa(1) = 1.86 for PhPO(OH)2.60 On the other hand, the 6,6′-dimethyl substituents in 1 will render the Me2bpy unit a weaker base than bpy, as is observed for 1,10-phenanthroline (pKa for [Hphen]+ = 4.86 (ref. 61)) and 2,9-dimethyl-1,10-phenanthroline (pKa for [HMe2phen]+ is reported as 5.85 (ref. 62) and 6.17 (ref. 61)). The zwitterionic form of 1 should exhibit a cis-configuration arising from hydrogen bonding63 (Scheme 2). Similarly, we expect 2 to exist as a zwitterion in water and DMSO (Scheme 2). Values of pKa = 4.54 and 3.57 for [Htpy]+ and [H2tpy]2+ (measured in 0.2 M aqueous KCl at 298 K)64 indicate that 3 is also likely to show zwitterionic behaviour (Scheme 2), as has also been reported for 2,2′:6′,2′′-terpyridin-4′-ylphosphonic acid.65 We expect the availability of phosphonate groups in the zwitterionic forms of the ligands to favour surface binding31 and the observed binding orders of 3 > 1 > 2 can be rationalized in terms of the relative pKa values and the number of anchoring groups in the ligands.
When activated TiO2 NPs were treated under microwave conditions with a 1
:
1 mixture of ligands 1 and 4, we observed that only carboxylic acid 4 remained in solution (Fig. 10). The strong adsorption of the phosphonic acid 1 to NPs dispersed in solution is consistent with what we and Grätzel have observed for annealed TiO2 surfaces.56–58 The preferential binding of 1 with respect to 4 was also demonstrated by ligand displacement. Activated TiO2 NPs were dispersed in an aqueous suspension of 4 and then heated under microwave conditions at 130 °C for 3 hours. After cooling, the water was removed, and part of the residue was suspended in DMSO-d6. Sharp signals in the 1H NMR spectrum were consistent with the presence of non-anchored ligand (Fig. 10a). The remaining residue was thoroughly washed until, NMR analysis of a part of the residue dispersed in DMSO-d6 revealed no signal for free ligand 4 (Fig. 11b). We demonstrated that some 4 ligand remained and was surface-bound by treating the residue with [Cu(MeCN)4][PF6], The observation of an orange colour (see the photograph in Fig. 11) is characteristic of the formation of a [Cu(diimine)2]+ chromophore (see earlier). The functionalized NPs were then treated with 1 in aqueous solution under microwave conditions (see Procedure C in the Experimental section). After removal of water, the residue was analysed by 1H NMR spectroscopy (Fig. 11c). The spectrum exhibited sharp signals with the signature of ligand 4. No free ligand 1 (compare with Fig. 10a) was observed, confirming the displacement of surface-bound 4 by 1.
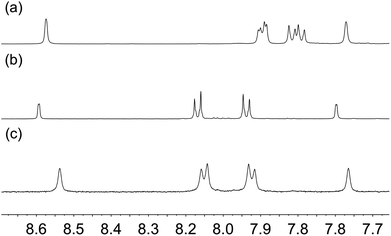 |
| Fig. 10
1H NMR (500 MHz, DMSO-d6, 298 K) of (a) ligand 1, (b) ligand 4, (c) suspended residue after activated TiO2 NPs had been treated with a 1 : 1 mixture of 1 and 4 following Procedure C in the Experimental section. | |
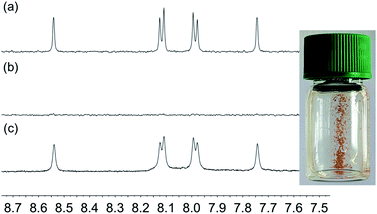 |
| Fig. 11
1H NMR (500 MHz, DMSO-d6, 298 K) of (a) suspended residue after activated TiO2 NPs had been treated with 4, (b) suspended residue after thorough washing (see text), and (c) suspended residue after NPs functionalized with 4 had been treated with 1 following Procedure D in the Experimental section. Chemical shifts in δ/ppm. The photograph shows the residue from (b) after treatment with [Cu(MeCN)4][PF6] (see text). | |
Application of SALSAC: preparation and characterization of homoleptic copper(I) complexes
To test the application of the SALSAC approach to the functionalization of NPs, we first prepared the homoleptic compounds [Cu(Lancillary)2][PF6] and [Fe(8)2][PF6]2. The iron(II) complex was prepared as previously described.47 The general method for the copper(I) complexes was the reaction of [Cu(MeCN)4][PF6] with two equivalents of Lancillary followed by precipitation of [Cu(Lancillary)2][PF6]. [Cu(5)2][PF6] has previously been described.44 Reaction of ligand 6 (ref. 45) with [Cu(MeCN)4][PF6] yielded [Cu(6)2][PF6]. Ligand 7 was prepared as shown in Scheme 3 and was characterized by 1H and 13C NMR spectroscopy (Fig. S16–S19†) with assignment of the signals by 2D methods (see Experimental section), electrospray MS (Fig. S20†) and IR spectroscopy (Fig. S21†). [Cu(7)2][PF6] was isolated as a red solid. The ESI mass spectra of [Cu(6)2][PF6] and [Cu(7)2][PF6] showed peaks at m/z 627.2 [M − PF6]+ and 1115.4, respectively, arising from the [M − PF6]+ ion. The 1H and 13C{1H} NMR spectra of [Cu(6)2][PF6] and [Cu(7)2][PF6] were assigned by COSY, NOESY, HMQC and HMBC methods and selected spectra are shown in Fig. S22–S28.† The solid-state IR spectra of the compounds (Fig. S29†) exhibit a characteristic strong absorption at 833 cm−1 from the [PF6]− ion. The solution absorption spectra of the two compounds (Fig. 12) exhibit intense absorption bands below ca. 340 nm arising from spin-allowed ligand-centred π* ← π transitions. The broad absorption band centred at 495 nm in [Cu(7)2][PF6] is assigned to an MLCT transition and is typical of homoleptic bis(diimine)copper(I) complexes in which the 6,6′-substituents are methyl groups.19,66 In contrast, [Cu(6)2][PF6] exhibits two bands at 430 and 554 nm which are reminiscent of those at 423 and 573 nm in [CuL2][PF6] where L = 4,4′-bis(4-bromophenyl)-6,6′-diphenyl-2,2′-bipyridine.44 The profile of the spectrum and the red-shift in absorption maximum are consistent with the effects of introducing phenyl substituents adjacent to the nitrogen donors in [Cu(dpp)2]+ (dpp = 2,9-diphenyl-1,10-phenanthroline),67,68 and have been attributed to the flattened, low-symmetry structure of the complex.68
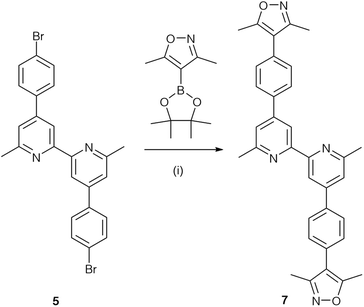 |
| Scheme 3 Synthetic route to compound 7. Conditions: (i) Cs2CO3, catalytic amount of [Pd(PPh3)4], toluene, 110 °C, 4 h in a microwave reactor; isolated in 33% yield. | |
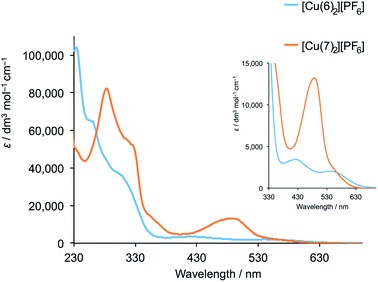 |
| Fig. 12 Solution absorption spectra of [Cu(6)2][PF6] (2.02 × 10−5 mol dm−3) and [Cu(7)2][PF6] (3.33 × 10−5 mol dm−3) in CH2Cl2. The inset shows an expansion of the MLCT region. | |
Application of SALSAC: NPs functionalized with heteroleptic copper(I) and iron(II) complexes
Route 2 in Fig. 1 summarized the SALSAC approach for TiO2 surface modification using ligand exchange between a surface-anchored ligand and one ligand from a homoleptic bis(diimine)copper(I) complex to produce a surface functionalized with a heteroleptic copper(I) complex (eqn (1)). | TiO2–Lanchor + [Cu(Lancillary)2]+ ⇄ TiO2–[(Lanchor)Cu(Lancillary)]+ + Lancillary | (1) |
To date, we have applied this strategy to annealed nanoparticulate TiO2 surfaces, and we now demonstrate an extension to TiO2 NPs dispersed in solution. NPs functionalized with 1 were dispersed in water, and an acetone solution of [Cu(5)2][PF6], [Cu(6)2][PF6] or [Cu(7)2][PF6] was added (see Experimental section). During a 72 hour period of stirring, the NPs became orange-red. A similar procedure was followed for ligand exchange between [Fe(8)2][PF6]2 and NPs functionalized with 3 which resulted in purple-coloured NPs. The NPs were separated by centrifugation, and were washed and dried. The observed colours of the NPs (Fig. 13) are diagnostic of [Cu(diimine)2]+ and [Fe(tpy)2]2+ chromophores, and their solid-state absorption spectra are shown in Fig. 14 and S30.† Absorption maxima are observed at 491, 498 and 488 nm for the copper(I) complexes with Lancillary = 5, 6 and 7, respectively, and at 577 nm for the surface bound iron(II) complex. That the latter arise from ligand exchange and not from residual homoleptic complexes is best demonstrated by comparing the solid-state spectrum of NPs functionalized with 1 after treatment with [Cu(6)2][PF6] with that of the homoleptic [Cu(6)2][PF6]. As discussed earlier, significant red-shifting is observed in the solution absorption spectrum of [Cu(6)2][PF6] due to flattening of the copper(I) coordination sphere, and this is also observed in the solid-state with an MLCT band centred at ca. 580 nm (Fig. 14).
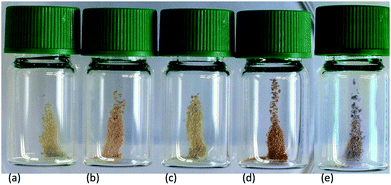 |
| Fig. 13 Photographs of activated TiO2 NPs functionalized (a) with 1, and after treatment with (b) [Cu(5)2][PF6], (c) [Cu(7)2][PF6], (d) [Cu(6)2][PF6] and (e) [Fe(8)2][PF6]2. | |
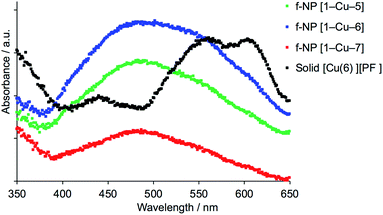 |
| Fig. 14 Solid-state absorption spectra of NPs functionalized with 1 after treatment with [Cu(5)2][PF6], [Cu(6)2][PF6] or [Cu(7)2][PF6], and, for comparison, the solid-state absorption spectrum of homoleptic [Cu(6)2][PF6]. | |
SEM images of the TiO2 NPs functionalized with 1 after treatment with [Cu(5)2][PF6] and [Cu(7)2][PF6] are displayed in Fig. 7c and d. Comparison with the images in Fig. 7a and b reveals an increase in particle size after reaction with the homoleptic copper complexes, consistent with the functionalization step in eqn (1).
Conclusions
We have demonstrated that the SALSAC approach to the hierarchical assembly of heteroleptic bis(dimiine)copper(I) complexes that we have previously established for annealed nanoparticulate TiO2 surfaces20 can be extended to NPs dispersed in solution. We have presented a general procedure for the activation of commercial TiO2 NPs by initial treatment with aqueous HNO3. After dispersion in water, the activated NPs were functionalized with anchoring ligands 1–4 by heating under microwave conditions, and the functionalized NPs were investigated using FT-IR, TGA, and 1H NMR spectroscopy. Ligands 1–3 contain phosphonic acid anchoring groups while 4 has two carboxylic acid anchors; ligands 1, 2 and 4 contain 6,6′-Me2bpy and 3 contains tpy metal binding domains. NPs functionalized with 1, 2 and 4 react with [Cu(MeCN)4][PF6] and those with 3 react with FeCl2·4H2O; metal binding has been investigated using solid-state absorption spectroscopy and SEM.
By reacting the activated NPs with mixtures of ligands, competitive binding of ligands to TiO2 has been investigated. For the phosphonic acids, the binding orders are 3 > 1 > 2 which can be rationalized in terms of relative pKa values (phosphonic acid and [HMe2bpy]+ or [Htpy]+) and the number of anchoring groups in the ligands. Competition experiments between 1 and 4 confirmed preferential binding of phosphonic over carboxylic acid anchors.
The final step in the SALSAC strategy is ligand exchange between ligand-functionalized NPs and homoleptic metal complexes to yield TiO2 NPs functionalized with heteroleptic complexes. We have demonstrated that this is successful for NPs functionalized with 1 reacted with three different [Cu(Lancillary)2][PF6] complexes and for NPs functionalized with 3 reacted with [Fe(8)2][PF6]2. This approach provides a versatile hierarchical approach to the formation of metal complex-functionalized NPs, and we are currently employing the SALSAC strategy in catalysis and sensing applications.
Conflicts of interest
There are no conflicts to declare.
Acknowledgements
We are grateful to the Swiss National Science Foundation (grant number 200020_182000) and the University of Basel for financial support. DOSY spectroscopy was performed by PD Dr D. Häussinger. TEM and SEM images were measured with the assistance of Susanne Erpel and Evi Bieler, respectively, at the Nano Imaging Lab, University of Basel and we also thank Dr Markus Dürrenberger. Ligands 3 and 6 were prepared by Alexandra Wiesler and Fabian Brunner (University of Basel), respectively. We also thank Mariia Karpacheva (University of Basel) for supplying [Fe(8)2][PF6]2.
References
- Z. Ren, Y. Guo, C.-H. Liu and P.-X. Gao, Front. Chem., 2013, 1, 1 CAS.
-
Modern Inorganic Synthetic Chemistry, ed. R. Xu and Y. Xu, Elsevier, Amsterdam, 2nd edn, 2017 Search PubMed.
-
Hierarchical Nanostructures for Energy Devices, ed. S. H. Ko and C. P. Grigoropoulos, RSC Nanoscience & Nanotechnology, No. 35, RSC, Cambridge, 2015 Search PubMed.
- S. Whitelam, Adv. Mater., 2015, 27, 5720 CrossRef CAS PubMed.
- S. Casalini, C. A. Bortolotti, F. Leonardi and F. Biscarini, Chem. Soc. Rev., 2017, 46, 40 RSC.
- A. Ulman, Chem. Rev., 1996, 96, 1533 CrossRef CAS PubMed.
- J. J. Gooding, F. Mearns, W. Yang and J. Liu, Electroanalysis, 2003, 15, 81 CrossRef CAS.
- A. Iyer and K. Paul, IET Nanobiotechnol., 2015, 9, 122 CrossRef PubMed.
- E. Mattia and S. Otto, Nat. Nanotechnol., 2015, 10, 111 CrossRef CAS PubMed.
- F. Tao, Pure Appl. Chem., 2008, 80, 45 CAS.
- S. De Feyter and F. C. De Schryver, J. Phys. Chem. B, 2005, 109, 4290 CrossRef CAS PubMed.
- A. Kumar, K. Banerjee and P. Liljeroth, Nanotechnology, 2017, 28, 082001 CrossRef PubMed.
-
D. N. Reinhoudt, Perspectives in Supramolecular Chemistry, Wiley, Chichester, 2008, vol. 20 Search PubMed.
- S. Stepanow, N. Lin, J. V. Barth and K. Kern, J. Phys. Chem. B, 2006, 110, 23472 CrossRef CAS PubMed.
- A. E. Danks, S. R. Hill and Z. Schnepp, Mater. Horiz., 2016, 3, 91 RSC.
- G. M. Whitesides, E. Ostuni, S. Takayama, X. Jiang and D. E. Ingber, Annu. Rev. Biomed. Eng., 2001, 3, 335 CrossRef CAS PubMed.
- R. E. P. Winpenny, J. Chem. Soc., Dalton Trans., 2002, 1 RSC.
- E. Schönhofer, B. Bozic-Weber, C. J. Martin, E. C. Constable, C. E. Housecroft and J. A. Zampese, Dyes Pigm., 2015, 115, 154 CrossRef.
- C. E. Housecroft and E. C. Constable, Chem. Soc. Rev., 2015, 44, 8386 RSC.
- F. J. Malzner, C. E. Housecroft and E. C. Constable, Inorganics, 2018, 6, 57 CrossRef.
-
From system complexity to energing properties, ed. M. Aziz-Alaoui and C. Bertelle, Springer, Berlin/Heidelberg, 2009 Search PubMed.
- F. J. Malzner, A. Prescimone, E. C. Constable, C. E. Housecroft and M. Willgert, J. Mater. Chem. A, 2017, 5, 4671 RSC.
- S. Y. Brauchli, F. J. Malzner, E. C. Constable and C. E. Housecroft, RSC Adv., 2014, 4, 62728 RSC.
- C. E. Housecroft, C. G. Palivan, K. Gademann, W. Meier, M. Calame, V. Mikhalevich, X. Zhang, E. Piel, M. Szponarski, A. Wiesler, A. Lanzilotto, E. C. Constable, A. Fanget and R. L. Stoop, Chimia, 2016, 70, 402 CrossRef CAS PubMed.
- For an overview, see: A. Winter, S. Hoeppener, G. R. Newkome and U. S. Schubert, Adv. Mater., 2011, 23, 3484 CrossRef CAS PubMed.
- See for example: W. R. McNamara, R. C. Snoeberger, G. Li, J. M. Schleicher, C. W. Cady, M. Poyatos, C. A. Schmuttenmaer, R. H. Crabtree, G. W. Brudvig and V. S. Batista, J. Am. Chem. Soc., 2008, 130, 14329 CrossRef CAS PubMed.
- S. P. Hill, T. Banerjee, T. Dilbeck and K. Hanson, J. Phys. Chem. Lett., 2015, 6, 4510 CrossRef CAS PubMed.
- See for example: S. Takahashi, S. Hotta, A. Watanabe, N. Idota, K. Matsukawa and Y. Sugahara, ACS Appl. Mater. Interfaces, 2017, 9, 1907 CrossRef CAS PubMed; J. Sun, E. J. Petersen, S. S. Watson, C. M. Sims, A. Kassman, S. Frukhtbeyn, D. Skrtic, M. T. Ok, D. S. Jacobs, V. Reipa, Q. Ye and B. C. Nelson, Acta Biomater., 2017, 53, 585 CrossRef PubMed; R. Bhandary, J. G. Alauzun, P. Hesemann, A. Stocco, M. In and P. H. Mutin, Soft Matter, 2017, 13, 8023 RSC; J. Kirschner, J. Will, T. J. Rejek, L. Portilla, M. Berlinghof, P. Schweizer, E. Spiecker, H.-G. Steinrück, T. Unruh and M. Halik, Adv. Mater. Interfaces, 2017, 4, 1700230 CrossRef; L. Zeininger, L. M. S. Stiegler, L. Portilla, M. Halik and A. Hirsch, ChemistryOpen, 2018, 7, 282 CrossRef PubMed and references therein.
- See for example: M. Nilsing, S. Lunell, P. Persson and L. Ojamäe, Surf. Sci., 2005, 582, 49 CrossRef CAS; C. Queffélec, M. Petit, P. Janvier, D. A. Knight and B. Bujoli, Chem. Rev., 2012, 112, 3777 CrossRef PubMed.
- V. Spampinato, N. Tuccitto, S. Quici, V. Calabrese, G. Marletta, A. Torrisi and A. Licciardello, Langmuir, 2010, 26, 8400 CrossRef CAS PubMed.
- A. J. Stephens, F. J. Malzner, E. C. Constable and C. E. Housecroft, Sustainable Energy Fuels, 2018, 2, 786 RSC.
- R. Calzada, C. M. Thompson, D. E. Westmoreland, K. Edme and E. A. Weiss, Chem. Mater., 2016, 28, 6716 CrossRef CAS PubMed.
- J. De Roo, K. De Keukeleere, Z. Hens and I. Van Driessche, Dalton Trans., 2016, 45, 13277 RSC.
- J. De Roo, Z. Zhou, J. Wang, L. Deblock, A. J. Crosby, J. S. Owen and S. S. Nonnenmann, Chem. Mater., 2018, 30, 8034 CrossRef CAS.
- L. Du, W. Wang, C. Zhang, Z. Jin, G. Palui and H. Mattoussi, Chem. Mater., 2018, 30, 7269 CrossRef CAS.
- C. Goldmann, F. Ribot, L. F. Peiretti, P. Quaino, F. Tielens, C. Sanchez, C. Chanéac and D. Portehault, Small, 2017, 13, 1604028 CrossRef PubMed.
- V. Grigel, L. K. Sagar, K. De Nolf, Q. Zhao, A. Vantomme, J. De Roo, I. Infante and Z. Hens, Chem. Mater., 2018, 30, 7637 CrossRef CAS.
- M. L. Kessler, H. E. Starr, R. R. Knauf, K. J. Rountree and J. L. Dempsey, Phys. Chem. Chem. Phys., 2018, 20, 23649 RSC.
- R. R. Knauf, J. C. Lennox and J. L. Dempsey, Chem. Mater., 2016, 28, 4762 CrossRef CAS.
- M. La Rosa, T. Avellini, C. Lincheneau, S. Silvi, I. A. Wright, E. C. Constable and A. Credi, Eur. J. Inorg. Chem., 2017, 2017, 5143 CrossRef CAS.
- A. Ritchhart and B. M. Cossairt, Inorg. Chem., 2019, 58, 2840 CrossRef CAS PubMed.
- G. J. Kubas, Inorg. Synth., 1979, 19, 90 CAS.
-
A. Hernández Redondo, Ph.D. thesis, University of Basel, 2009, http://edoc.unibas.ch/diss/DissB_8757.
- B. Bozic-Weber, S. Y. Brauchli, E. C. Constable, S. O. Fürer, C. E. Housecroft, F. J. Malzner, I. A. Wright and J. A. Zampese, Dalton Trans., 2013, 42, 12293 RSC.
- F. Brunner, S. Graber, Y. Baumgartner, D. Häussinger, A. Prescimone, E. C. Constable and C. E. Housecroft, Dalton Trans., 2017, 46, 6379 RSC.
- J. Wang and G. S. Hanan, Synlett, 2005, 1251 CAS.
- E. C. Constable, J. Lewis, M. C. Liptrot and P. R. Raithby, Inorg. Chim. Acta, 1990, 178, 47 CrossRef CAS.
-
https://www.aerosil.com/sites/lists/RE/DocumentsSI/TI-1243-Titanium-Dioxide-as-Photocatalyst-EN.pdf, accessed 31 July 2019.
- L. Zhang and J. M. Cole, ACS Appl. Mater. Interfaces, 2015, 7, 3427 CrossRef CAS PubMed.
- M. K. Eggleston, D. R. McMillin, K. S. Koenig and A. J. Pallenberg, Inorg. Chem., 1997, 36, 172 CrossRef CAS.
- N. A. Gothard, M. W. Mara, J. Huang, J. M. Szarko, B. Rolczynski, J. V. Lockard and L. X. Chen, J. Phys. Chem. A, 2012, 116, 1984 CrossRef CAS PubMed.
- Y. M. Klein, M. Willgert, A. Prescimone, E. C. Constable and C. E. Housecroft, Dalton Trans., 2016, 45, 4659 RSC.
- S. P. Pujari, L. Scheres, A. T. M. Marcelis and H. Zuilhof, Angew. Chem., Int. Ed., 2014, 53, 6322 CrossRef CAS PubMed.
- B. Kim, S. W. Park, J.-Y. Kim, K. Yoo, J. A. Lee, M.-W. Lee, D.-K. Lee, J. Y. Kim, B. S. Kim, H. Kim, S. Han, H. J. Son and M. J. Ko, ACS Appl. Mater. Interfaces, 2013, 5, 5201 CrossRef CAS PubMed.
- See for example: A. Estrada-Monje, R. Zitzumbo-Guzmán, J. A. Bañuelos-Díaz and E. A. Zaragoza-Contreras, Mater. Chem. Phys., 2019, 235, 121760 CrossRef CAS.
- B. Bozic-Weber, E. C. Constable, C. E. Housecroft, P. Kopecky, M. Neuburger and J. A. Zampese, Dalton Trans., 2011, 40, 12584 RSC.
- B. Bozic-Weber, V. Chaurin, E. C. Constable, C. E. Housecroft, M. Meuwly, M. Neuburger, J. A. Rudd, E. Schönhofer and L. Siegfried, Dalton Trans., 2012, 41, 14157 RSC.
- P. Péchy, F. P. Rotzinger, M. K. Nazeeruddin, O. Kohle, S. M. Zakeeruddin, R. Humphry-Baker and M. Grätzel, J. Chem. Soc., Chem. Commun., 1995, 65 RSC.
- A. Moissette, Y. Batonneau and C. Brémard, J. Am. Chem. Soc., 2001, 123, 12325 CrossRef CAS PubMed.
-
Metal Phosphonate Chemistry: From Synthesis to Applications, ed. A. Clearfield and K. Demadis, RSC Publishing, 2011, ch. 5, p. 166 Search PubMed.
- G. Clauti, G. Zassinovich and G. Mestroni, Inorg. Chim. Acta, 1986, 112, 103 CrossRef CAS.
- G. D. Stevens and R. A. Holwerda, Inorg. Chem., 1984, 23, 2777 CrossRef CAS.
- See for example: A. D. Burrows, R. W. Harrington and M. F. Mahon, Acta Crystallogr., Sect. C: Cryst. Struct. Commun., 1999, 55, 1921 CrossRef CAS; W. S. Wu, Acta Crystallogr., Sect. E: Struct. Rep. Online, 2011, 67, o2070 CrossRef PubMed; E. C. Constable, C. E. Housecroft, M. Neuburger, P. Rösel, G. E. Schneider, J. A. Zampese, F. Monti, N. Armaroli, R. D. Costa and E. Orti, Inorg. Chem., 2013, 52, 885 CrossRef PubMed.
- E. Farkas, É. A. Enyedy, G. Micera and E. Garribba, Polyhedron, 2000, 19, 1727 CrossRef CAS.
- M. K. Nazeeruddin, S. M. Zakeeruddin, R. Humphry-Baker, T. A. Kaden and M. Grätzel, Inorg. Chem., 2000, 39, 4542 CrossRef CAS.
- See for example: F. J. Malzner, S. Y. Brauchli, E. C. Constable, C. E. Housecroft and M. Neuburger, RSC Adv., 2014, 4, 48712 RSC; A. Büttner, S. Y. Brauchli, E. C. Constable and C. E. Housecroft, Inorganics, 2018, 6, 40 CrossRef CAS.
- A. K. Ichinaga, J. P. Kirchhoff, D. R. McMillin, C. O. Dietrich-Buchecker, P. A. Marnot and J. P. Sauvage, Inorg. Chem., 1987, 26, 4290 CrossRef CAS.
- M. T. Miller, P. K. Gantzel and T. B. Karpishin, Inorg. Chem., 1998, 37, 2285 CrossRef CAS.
Footnote |
† Electronic supplementary information (ESI) available: Instrument details; Fig. S1–S6: mass spectrum and NMR spectra of 2; Fig. S7: DOSY fits; Fig. S8 and S9: IR spectra of NPs functionalized with 1 and 3; Fig. S10; TGA curve of 1; Fig. S11–S13 and S15: NMR spectra of competition experiments; Fig. S14: photographs of activated TiO2 NPs functionalized 2 and 4; Fig. S16–S21: mass spectrum and NMR and IR spectra of 7; Fig. S22–S29: NMR and IR spectra of [Cu(6)2][PF6] and [Cu(7)2][PF6]; Fig. S30: absorption spectrum of NPs functionalized with 3 after treatment with [Fe(8)2][PF6]2. See DOI: 10.1039/c9na00488b |
|
This journal is © The Royal Society of Chemistry 2020 |
Click here to see how this site uses Cookies. View our privacy policy here.