DOI:
10.1039/D0FO02159H
(Paper)
Food Funct., 2021,
12, 328-339
Oroxylin A attenuates osteoarthritis progression by dual inhibition of cell inflammation and hypertrophy
Received
16th August 2020
, Accepted 17th November 2020
First published on 20th November 2020
Abstract
The imbalance between the anabolism and catabolism of the extracellular matrix (ECM) is of great importance to osteoarthritis (OA) development. Aberrant inflammatory responses and hypertrophic changes of chondrocytes are the main contributors to these metabolic disorders. In the present study, we found that Oroxylin A (ORA), a flavonoid compound derived from Oroxylum indicum, maintained ECM hemostasis of chondrocytes by Interleukin-1β (IL-1β) stimulation. Besides, it was demonstrated that IL-1β induced over-production of inflammatory mediators was attenuated by ORA treatment. Moreover, ORA could rescue IL-1β mediated hypertrophic alterations of chondrocytes. Mechanistically, ORA's protective effects were found to be associated with both NF-κB and Wnt/β-catenin signaling inhibition. Meanwhile, molecular docking analysis revealed that ORA could strongly bind to the inhibitor kappa B kinaseβ (IKKβ) and dishevelled, Dsh Homolog 2 (Dvl2), the upstream molecules of the NF-κB axis and β-catenin axis, respectively. In addition, ORA driven chondroprotective effects were also affirmed in a surgically induced OA mouse model. Taken together, the current study suggested that ORA might be a promising therapeutic option for the treatment of OA.
1. Introduction
Osteoarthritis (OA) is one of the most prevalent degenerative joint diseases worldwide, which leads to severe functional loss of joints and declines patients’ life quality significantly.1–3 Risk factors such as age, obesity, trauma, sex, and genetics are correlated with OA.4,5 The lesion of OA involves the total joint, including the articular cartilage exfoliation, subchondral bone remolding, and chronic synovitis.4 Over several decades, scientists have been trying to figure out the etiology of OA. Although the specific mechanism is still unknown, inflammatory microenvironments and hypertrophic changes of chondrocytes are increasingly recognized as contributors to the progression of OA.6 Among many harmful stimuli, the inflammatory factors are the most important ones. IL-1β, one of the most typical inflammatory stimuli, is implicated as a principal instigator of OA, and it is capable of inducing the expression of inflammation-related enzymes and many inflammatory mediators, leading to the destruction of the extracellular matrix (ECM) of cartilage.7 What is more, it was reported that IL-1β could induce phenotypic changes in chondrocytes, which inhibited the anabolism of cells themselves and disturbed the self-renewal of the ECM.8 Thus, targeting both the external inflammatory microenvironments and internal degeneration of chondrocytes is an effective strategy for OA management.
A series of signaling pathways are involved in IL-1β induced inflammation in OA.9,10 However, the NF-κB pathway stands as a central regulator.11 After being triggered by IL-1β, the IkB proteins would be phosphorylated by the IKK complex and degraded by proteomes, which release NF-κB p65 that translocate into the nucleus and bind to the target gene to trigger cascades of cytokine expression, such as adhesion molecules, inflammatory mediators, and several ECM degrading enzymes.11–13 According to much evidence published previously, vertically inhibiting the NF-κB axis, including modulating its upstream molecules or directly targeting NF-κB, proved to be conducive to OA treatment.14 On the other hand, Wnt signaling is another essential pathway activated by IL-1β and has drawn more and more attention for its potential for OA treatment.15–17 In the canonical Wnt/β-catenin pathway, the secreted Wnt proteins integrated with coreceptors, including the Frizzled (Fz)- and lipoprotein-related proteins (LRP) followed by activation of intracellular dishevelled proteins 2 (Dvl2). Then, the activated Dvl2 weakens the ability of GSK-3α to β-catenin phosphorylation, resulting in the escape of unphosphorylated β-catenin proteins from ubiquitylation and degradation. The free β-catenin would accumulate and would be transferred from the cytoplasm into the nucleus, where it regulates the transcription of Wnt target genes with the help of the lymphoid enhancer factor (LEF)/T-cell factor (TCF) family.18,19 In the early stages of chondrogenesis, the Wnt signaling pathway was shown to regulate cell proliferation and differentiation, thus contributing a lot to limb development. However, in the process of OA, the over-activated Wnt signaling pathway converts the proliferation status of chondrocytes to hypertrophic and calcified conditions.15 In addition, the application of small-molecule inhibitors of the Wnt/β-catenin axis was proved to be capable of inhibiting IL-1β- and tumor necrosis factor α (TNFα)-induced cartilage degradation.20,21 Hence, emphasizing both NF-κB and Wnt/β-catenin axis inhibition by IL-1β stimulation is instructive to drug exploitation for OA treatment.
Oroxylum indicum is a kind of traditional plant that exists in many Asian countries such as China and Thailand.22 The seeds of Oroxylum indicum can be used as an auxiliary food material in some soups and can be used to make tea. Furthermore, as a traditional Chinese medicine admired by ancient Chinese herbalists, Oroxylum indicum has been used in countless Chinese medicinal formulae to treat a considerable number of diseases.23 Oroxylin-A (ORA) is an active ingredient of Oroxylum indicum, which exerts anti-inflammatory, anti-apoptotic, and anti-tumor effects.24 Huang et al. found the protective effect of ORA against lipopolysaccharide and/or D-galactosamine-induced acute liver injury in mice by inhibiting the activation of NF-κB.25 Meanwhile, it was shown that ORA could prevent the deterioration of inflammation-related tumorgenesis and acute lung injury via NF-κB signaling inhibition.26,27 What is more, ORA was also reported to inactivate the Wnt pathway, arousing cell cycle arrest in the G2/M phase to suppress the development and growth of colorectal cancer.28 More importantly, the protective effects of ORA in attenuating rheumatoid arthritis, another prevalent joint disease caused by immune disarrangement, has been demonstrated in an animal model.29 However, whether ORA could prevent OA progression has not yet been investigated. Therefore, in the current study, the chondroprotective effects of ORA on IL-1β induced primary human chondrocytes and surgically induced OA mouse models were assessed, and the underlying molecular mechanism was also explored.
2. Materials and methods
2.1. Reagents
Oroxylin-A (purity > 98%) was bought from Yuanye Bio-Technology Co., Ltd (Shanghai, China). For an in vitro study, ORA was dissolved in DMSO to 200 mM and stored at −20 °C. Before every experiment, the stock solution of ORA was diluted with a basal medium to various working concentrations. For an in vivo study, ORA was dissolved in 0.5% sodium carboxymethylcellulose (CMC-Na, diluted in saline). Type II collagenase was obtained from Solarbio (Beijing, China). Recombinant human IL-1β was acquired from PeproTech (NJ, USA). Anti-p65, anti-collagen X, anti-β-catenin, anti-Lamin B1, and anti-GADPH were purchased from Abcam (Cambridge, UK), the antibody of iNOS was acquired from Sigma-Aldrich (St Louis, MO, USA), RUNX-2 primary antibody, goat anti-rabbit, and anti-mouse IgG-HRP were obtained from Bioworld (OH, USA), and primary antibodies against COX-2 and IκBα were purchased from Cell Signaling Technology (Danvers, MA, USA). Alexa Fluor®488 labeled and Alexa Fluor®594 labeled goat anti-rabbit IgG (H + L) secondary antibodies and 4′,6-diamidino-2-phenylindole (DAPI) were obtained from Yeasan (Shanghai, China). Cell culture reagents were obtained from Gibco (Grand Island, NY, USA). Human collagen II, aggrecan, MMP13, ADAMTS-5, TNF-α, and IL-6 ELISA kits were obtained from R&D Systems (Minneapolis, MN, USA). A PEG2 ELISA kit was purchased from KeyGen BiotechCo., Ltd (Nanjing, China). A cell counting kit-8 (CCK-8) was purchased from Dojindo Co., Ltd (Kumamoto, Japan).
2.2. Primary human chondrocyte culture
The Medical Ethical Committee of the Second Affiliated Hospital, Wenzhou Medical University, has proved this study. The collection of human cartilage tissue followed the guidelines of the Declaration of Helsinki.30 Signed informed consent was obtained from all patients involved in our study. The protocol for isolation of primary human articular chondrocytes was based on our previous study.31 Briefly, the articular cartilage samples were collected from the patient who received amputation surgeries due to severe traffic trauma. The hyaline cartilage was rinsed three times with PBS and then sliced into pieces. Then the tissue suspension was centrifuged and the supernatants were removed. The precipitated tissues were digested by tenfold of the collagenase II solution (300 U mL−1, dissolved in DMEM/F12) at 37 °C for 5 hours. After digestion, the isolated chondrocytes and tissue debris were washed with PBS and resuspended at a density of 2 × 105 cells per mL in complete DMEM/F12 medium (supplemented with 10% FBS and 1% penicillin–streptomycin) and seeded in a 75 mm2 culture flask under an atmosphere of 5% CO2 at 37 °C. Chondrocytes no later than three passages were used for the following experiments.
2.3. Cell viability assay
A CCK-8 was used to measure the cytotoxicity of ORA to chondrocytes. The digested chondrocytes were seeded into 96-well plates at a density of 5000 cells per well followed by treatment with different concentrations of ORA (0, 2.5, 5, 10, 20, and 50 μM) for 24 h or 48 h. At the designed time points, the cells were rinsed with PBS and incubated with 100 μl of CCK-8 working solution (1
:
9 diluted in DMEM/F12) for 3 h at 37 °C. Finally, the absorbance of the reactive solution was read at 450 nm using a microplate reader (Thermofisher).
2.4. NO measurement
Griess reagent assay was used to detect the nitrite production of culture supernatants after chondrocytes were treated differently. In brief, the supernatants were collected and mixed with an equal volume of the Griess reagent, followed by incubation at 37 °C for 30 minutes. A microplate reader was used to measure the optical density at 540 nm.
2.5. Enzyme-linked immunosorbent assay (ELISA)
The levels of MMP13, ADAMTS-5, collagen II, aggrecan, PGE2, TNF-α, and IL-6 in the cell culture medium were measured using commercial ELISA assay kits according to their manufacturers’ instructions. The supernatants from different treatment cell groups were collected and further assessed, and the results were expressed as picograms per milliliter.
2.6. Immunofluorescence staining
Immunofluorescence staining was used in the in vitro study to measure the contents and location of collagen II, MMP-13, and P65 in chondrocytes after different treatments. As for collagen II and MMP-13 staining, the cells were firstly seeded into 24-well plates. After reaching 80% of frequency, the cells were treated with IL-1β alone or combined with 20 μM of ORA for 24 h. For p65 examination, the incubation time was reduced to 2 h. The cells were rinsed thrice with PBS, fixed in a 4% paraformaldehyde solution for 10 min, and sequentially permeabilized with a 0.5% Triton X-100 solution for 15 min. Then, the cells were incubated with 5% goat serum for 1 h at 37 °C and incubated with anti-collagen II (1
:
200), anti-MMP-13 (1
:
200), and anti-p65 (1
:
200) at 4 °C for 8 h. Subsequently, the cells were washed three times with PBS and treated with Alexa Fluor®488 labeled or Alexa Fluor®594 conjugated secondary antibodies (1
:
400) for 1 h at room temperature. After being labeled with DAPI for 10 min, fluorescence images were captured using an inverted fluorescence microscope (Leica, Germany).
2.7. Protein extraction
Whole-cell proteins were extracted from chondrocytes using RIPA lysis buffer containing protease inhibitors. Nuclear and cytoplasmic proteins were extracted using a Nuclear and Cytoplasmic Protein Extraction Kit (Beyotime, Shanghai, China). Lysates were lysed on ice and sonicated by ultrasound. After being centrifuged at 12
000 rpm for 30 min at 4 °C, the supernatants were collected, and the content of protein samples was quantitated in the final concentration of 30 μg/20 μl using a BCA protein assay kit (Beyotime, Shanghai, China).
2.8. Western blotting
Sodium dodecyl sulfate-polyacrylamide gel electrophoresis (SDS PAGE) was used to separate the protein samples, and the obtained gel blots were transferred onto polyvinylidene difluoride membranes (Bio-Rad, USA). After being blocked with 5% nonfat milk for 2 h at room temperature, the membranes were incubated with the primary antibody against GAPDH (1
:
5000), iNOS (1
:
1000), COX-2 (1
:
1000), p65 (1
:
1000), IκBα (1
:
1000), Lamin B1 (1
:
1000), RUNX-2 (1
:
500), collagen X (1
:
1000) and β-catenin(1
:
1000) at 4 °C overnight. On the next day, the membranes were washed three times with TBST, followed by incubation with the corresponding secondary antibodies for 2 h. After being rinsed 3 times again with PBS, finally the blots were visualized using electrochemiluminescence plus reagent (Invitrogen). For quantification, the greyscale of the obtained blots was detected using Image Lab 3.0 software (Bio-Rad).
2.9. ALP staining
For the ALP activity determination, initially the cells were cultured in a hypertrophic medium for 2 weeks. The composition of the hypertrophic medium was according to the previously published method: complete DMEM medium supplemented with 1% (v/v) insulin–transferrin–selenium (ITS), 50 mg ml−1 ascorbate-2-phosphate, 40 mg ml−1L-proline, 100 nM dexamethasone, and 1 nM triiodothyronine (all from Sigma). After 14 days of induction, the level of alkaline phosphatase (ALP), a marker of chondrocyte hypertrophy, was assayed using a BCIP/NBT ALP color development kit (Beyotime) according to the manufacturer's instructions.
2.10. Molecular docking
The molecular structure of ORA was drawn using ChemBioDraw, and the energy of ORA was minimized using ChemBio3D software. The 3D crystal structures of IKKβ (code: 3RZF), p50 (code: 1NFK), proteasome (code: 1D3Z), Dvl2 (code: 3CC0), TCF-4 (code: 1JDH), and LEF-1 (code: 3OUX) were downloaded from the Protein Data Bank (https://www.rcsb.org/). Protein–ligand docking analysis was simulated with AutoDock Vina and the binding scores were determined by default parameters. Finally, the 3D view image of the lowest energy conformation of the protein–ligand complex was created using PyMOL (Version 1.7.6) and the relative 2D view image was generated using Ligplot+ software.
2.11. Animal model
Forty-five 8-week-old C57BL/6 female mice were acquired from the Animal Center of Chinese Academy of Sciences, Shanghai. All animal procedures were performed in accordance with the Guidelines for Care and Use of Laboratory Animals of Wenzhou Medical University and approved by the Animal Ethics Committee of Wenzhou Medical University. The mouse OA model was established by surgical destabilization of the medial meniscus (DMM) as antecedently described with slight modifications.32 Briefly, following anesthesia with 2% (w/v) pentobarbital (40 mg kg−1, ip), the joint capsule of the right knee was incised just medial to the patellar tendon by scalpel, and the medial meniscus and meniscotibial ligament were transected sequentially, followed by layer-by-layer suture of soft tissues and skin. For the sham group, the arthrotomy surgery without meniscus and ligament transection was performed on the same knee. After the operation, penicillin (80
000 U kg−1, im) was injected for three days to prevent possible infection. The mice were randomly divided into three groups (n = 15 each): sham group, DMM group, and DMM + ORA treatment group. 4 weeks after surgery, the ORA treatment groups received ORA at a dosage of 10 mg kg−1 day−1 by oral gavage for 4 consecutive weeks, while the DMM mice received only an equivalent volume of saline (contained 0.5% CMC-Na). Eight weeks postoperative, all mice were sacrificed, and the joint samples were collected for the following radiographic and histological investigation.
2.12. Radiographic testing
X-ray examination of mice was carried out at eight weeks postoperative. The osteophyte formation, joint narrowing as well as calcification of the cartilage surface were detected with a Kubtec Model XPERT.8 X-ray machine (KUB Technologies Inc.). The radiographs were obtained under the setting of 160 μA and 50 kV.
2.13. Histopathological analysis
After removing the appendant tissues, the joint sample of mice was collected and fixed in 4% paraformaldehyde for 48 h. Then, the joints were decalcified with a 10% ethylenediaminetetraacetic acid (EDTA) solution using a shaker at 4 °C for 1 month. After that, the decalcified tissues were dehydrated in gradient alcohols, followed by embedding in paraffin. A microtome was used to cut the sample into 5-μm-thick sections. Then, Safranin O-fast green (S–O) staining was performed for morphological observation and quantification. The stained slides were captured digitally using a microscope. The severity of OA, according to histological finding, was quantified by using the Osteoarthritis Research Society International (OARSI) scoring system based on the previously published protocol.33
2.14. Statistical analysis
The above-mentioned experiments were all performed at least five times independently. The sample size for each experiment was determined based on our previous experiments. All data were presented as mean ± SD, as indicated in the figure legends. Unpaired Student's t-test (for two groups), one-way ANOVA (for multiple groups), and two-way ANOVA (for multiple groups and time points) were performed, followed by the Tukey–Kramer test using the GraphPad Prism software version 8.0. p < 0.05 was considered to be statistically significant.
3. Results
3.1. ORA showed no cytotoxicity to primary human chondrocytes at the concentration of 0–50 μM.
Fig. 1A represents the chemical structure of ORA (5,7-dihydroxy-6-methoxy-2-phenylchromen-4-one). A CCK-8 assay was used to test the cytotoxicity of ORA to chondrocytes at increasing concentrations (0, 2.5, 5, 10, 20, and 50 μM) for 24 h and 48 h. As is shown in Fig. 1B and C, ORA increases the viability of chondrocytes at concentrations ranging from 2.5 to 20 μM (both 24 and 48 h). Although the 50 μM ORA group exerts no cytotoxicity compared with the control group, the cell viability decreases at this concentration in comparison with the 20 μM ORA group. Therefore, 5, 10, and 20 μM concentrations of ORA were chosen for subsequent experiments.
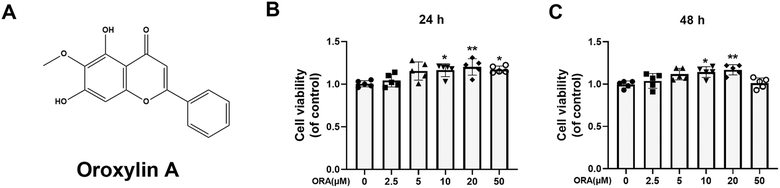 |
| Fig. 1 Effect of ORA on the viability of human chondrocytes. (A) Chemical structure of ORA. (B and C) Cell viability of chondrocytes after treatment with different concentrations of ORA for 24 h and 48 h. All data were presented as mean ± SD. **p ≤ 0.01 and *p ≤ 0.05 compared to the control group. | |
3.2. ORA inhibited IL-1β induced imbalance of ECM hemostasis in human chondrocytes
MMP-13 and ADAMTS-5 are two representative metalloproteinases that play a vital role in the deterioration of the ECM. According to the ELISA results, IL-1β improved the expression of MMP-13 and ADAMTS-5, resulting in the decline of collagen-II and aggrecan (Fig. 2A–D). However, such a situation can be reversed by ORA treatment in a dose-dependent manner. What is more, the results of immunofluorescence staining of MMP-13 and collagen II are in agreement with the ELISA results. The ORA treated chondrocytes showed higher collagen II intensity but lower MMP13 intensity than those of the IL-1β stimulated group (Fig. 2E and F).
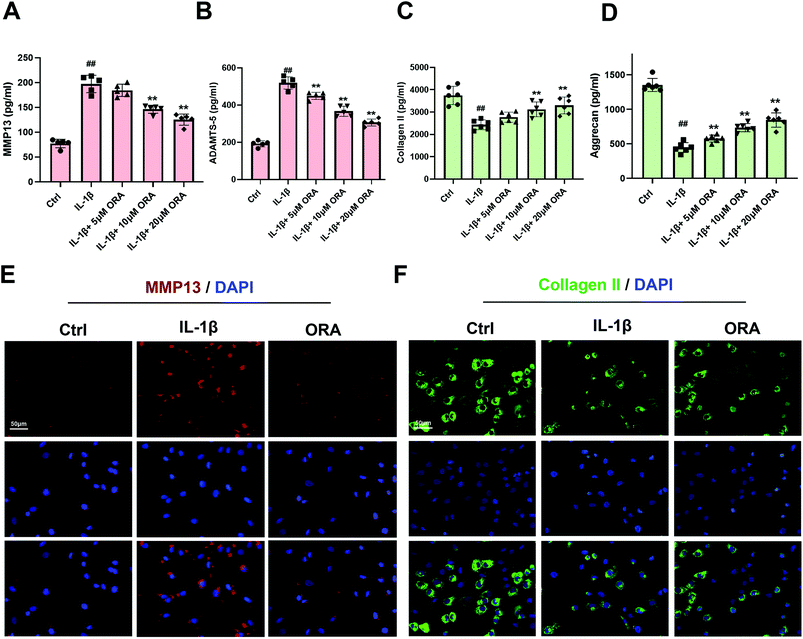 |
| Fig. 2 Influence of ORA on IL-1β-mediated ECM degeneration in human chondrocytes. (A–D) The levels of MMP13, ADAMTS-5, Collagen II, and aggrecan in the culture medium of chondrocytes treated as indicated were quantified by ELISA. (E and F) The representative MMP13 and collagen II images were obtained by immunofluorescence combined with DAPI staining for nuclei. All data were presented as mean ± SD. ##p ≤ 0.01 compared to the control group; **p ≤ 0.01 compared to the IL-1β treated group. | |
3.3. ORA attenuated IL-1β induced cell inflammation in human chondrocytes by inhibiting NF-κB activation
According to the Griess reagent and ELISA assay results, IL-1β stimulation raised the level of endogenic NO, PGE2, IL-6, and TNF-α. However, this phenomenon was reverted by the treatment of ORA in a dose-dependent manner (Fig. 3A–D). Meanwhile, as is shown in Fig. 3E and F, ORA also down-regulated the IL-1β mediated increased levels of COX-2 and iNOS in a dose-dependent manner. Followed by the detection of the critical molecule changes in the NF-κB axis, IL-1β was found to decrease the levels of IκBα in the cytoplasm but increase the p65 level in the nucleus according to the western blot results. Nevertheless, it was observed that pretreatment with ORA in chondrocytes inhibited the degradation of IκBα and declined the nuclear translocation of p65 in a dose-dependent manner (Fig. 3G and H). Immunofluorescence staining of p65 was implemented to intuitively examine the nuclear translocation of p65. Chondrocytes were divided into three groups: (1) control group, (2) IL-1β-stimulated group, and (3) IL-1β + ORA (20 μM) treatment group. In the control group, substantial p65-active proteins were found in the cytoplasm. In contrast, in the IL-1β-stimulated group, the great mass of p65-active proteins was found to be located in the nucleus. As expected, in the ORA pretreatment group, such a nuclear translocation phenomenon had been significantly blocked (Fig. 3I).
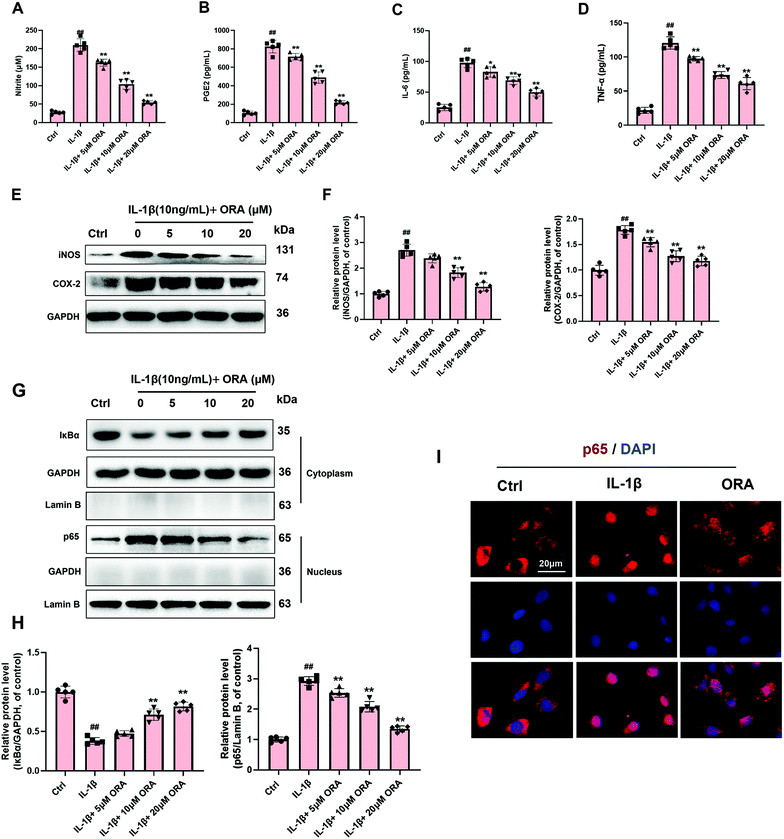 |
| Fig. 3 Influence of ORA on IL-1β-induced inflammatory reaction in human chondrocytes. (A–D) Effect of ORA on IL-1β-mediated NO, PGE2, IL-6, and TNF-α production in human chondrocytes. (E and F) iNOS and COX-2 levels in chondrocytes treated as indicated were measured by western blot. (G and H) The protein level of IκBα in the cytoplasm and p65 in the nucleus in chondrocytes treated as indicated were detected by western blot. (I) The nuclear translocation of p65 was detected by immunofluorescence combined with DAPI staining for nuclei. All data were presented as mean ± SD (n = 6). ##p ≤ 0.01 compared to the control group; **p ≤ 0.01 compared to the IL-1β treated group. | |
3.4. ORA attenuated the IL-1β induced hypertrophic changes in chondrocytes by inhibiting the Wnt/β-catenin signaling pathway
After IL-1β stimulation, the hypertrophic markers of chondrocytes, including RUNX-2 and collagen X, were increased significantly. Not surprisingly, ORA administration caused a concentration-dependent decrease of these two markers. Except for the western blot results (Fig. 4A and B), the ALP activity was found to be elevated in the IL-1β group, whereas ORA treated cells expressed lower ALP levels in a dose-dependent manner (Fig. 4C). For hypertrophy related signaling exploration, the critical molecules in the Wnt/β-catenin pathway were detected. The western blot analysis indicated that IL-1β enormously improved the β-catenin level in both the cytoplasm and nucleus. However, ORA pretreatment prominently suppressed such an increasing trend in a dose-dependent manner, especially at the nuclear level (Fig. 4D and E).
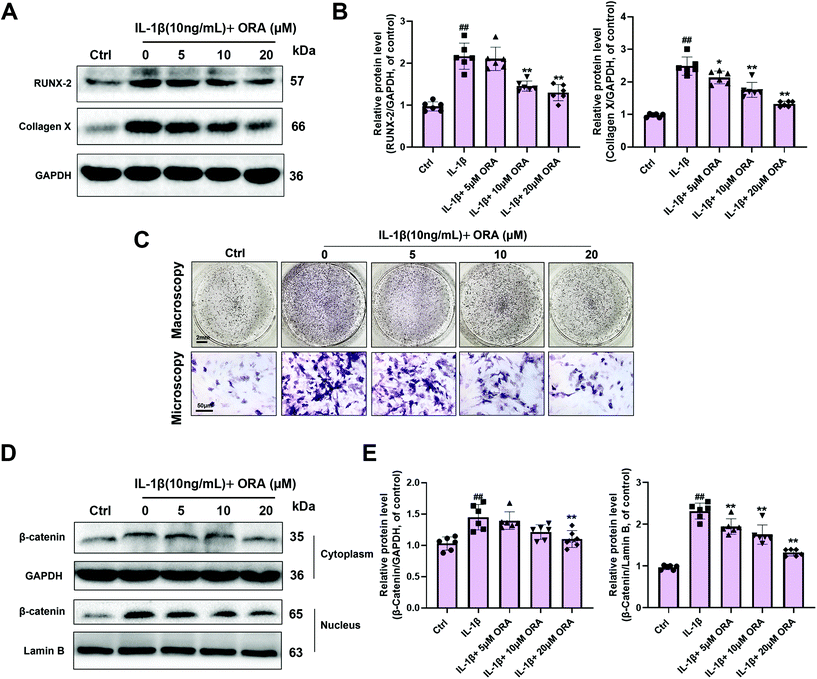 |
| Fig. 4 Influence of ORA on IL-1β-mediated hypertrophic changes in human chondrocytes. (A and B) RUNX-2 and Collagen X levels in chondrocytes treated as indicated were measured by western blot. (C) ALP activity of different treatment groups was visualized using BNIP/NBT kits. (D and E) The protein levels of β-catenin in both the cytoplasm and nucleus in chondrocytes treated as indicated were detected by western blot. All data were presented as mean ± SD. ##p ≤ 0.01 compared to the control group; **p ≤ 0.01 compared to the IL-1β treated group. | |
3.5. ORA targeted IKKβ and Dvl2 according to the molecular simulation
In order to detect the potential target signaling molecules of ORA, molecular docking was applied. The affinity of ORA to IKKβ, proteasome, and p50/p65 interaction in the NF-κB axis was firstly studied. After calculating using AutoDock Vina (Fig. 5A), the highest binding score of the top 18 formed conformations was found in ORA/IKKβ complex (7.76 ± 0.29), followed by ORA/p50/p65 complex (6.70 ± 0.22) and the ORA/proteasome complex (5.06 ± 0.24). In the Wnt/β-catenin axis, the highest scores were presented in the binding model of ORA to Dvl2 (Fig. 5B). Specifically, according to the simulation, it revealed that ORA could be well embedded in the protein structure of IKKβ and Dvl2, and several intermolecular interactions were formed. Four hydrogen bonds were formed between ORA and the Cys99 and Glu100 residues of IKKβ. Meanwhile, in the 2D image, five hydrophobic bonds were formed between ORA and the around residues of IKKβ, including Met96, Ile165, Val152, Val29, Asp103, and Tyr98 (Fig. 5C). Similarly, a strong hydrogen bond was formed between ORA and the Trp363 residue of Dvl2, and a large number of hydrophobic bonds were formed between ORA and His342, Arg338, Asn276, Leu278, Val341, Met272, Asp365, and Ser364 as shown in Fig. 5D. These bonds support the strong affinity of ORA to IKKβ and Dvl2.
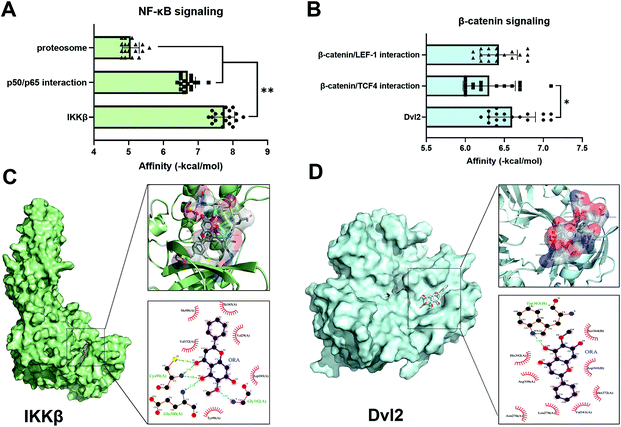 |
| Fig. 5
In silico molecular docking analysis of ORA to target molecules. (A) Quantification of the affinity of ORA to the key molecules in the NF-κB axis. (B) Quantification of the affinity of ORA to the key molecules in the Wnt/β-catenin axis. (C) Local interaction, including formed hydrogen bonds and hydrophobic bonds between ORA and around residues of IKKβ. (D) Local interaction, including formed hydrogen bonds and hydrophobic bonds between ORA and around residues of Dvl2. The data in the figures represent mean ± SD. Significant differences among the different groups are indicated as *P < 0.05 and **P < 0.01. | |
3.6. ORA ameliorates OA development in a mouse DMM model
In order to study the role of ORA in OA development in vivo, a mouse OA model was established through surgery. X-ray examination revealed that the DMM group showed conspicuous stricture of the joint space, abnormal osteophyte formation, and increased calcification of the cartilage surface in comparison with the sham group. However, in the ORA treated group, although a narrow gap between the joints still existed, the calcification of the cartilage became milder than in the DMM group, and the number of osteophytes were fewer (Fig. 6A). Histologically, Safranin O staining revealed that the DMM group exhibited severe pathological changes, including the bumpy of cartilage, distinct hypocellularity and loss of glycosaminoglycan (less red stained), in contrast to the sham group. Nevertheless, these changes can be alleviated by treatment with ORA (10 mg kg−1) (Fig. 6B). Accordingly, the OARSI scores were in accordance with the histological findings. The score of the DMM group was higher than that of the sham control as expected, while treatment with ORA significantly reduced the score (Fig. 6C).
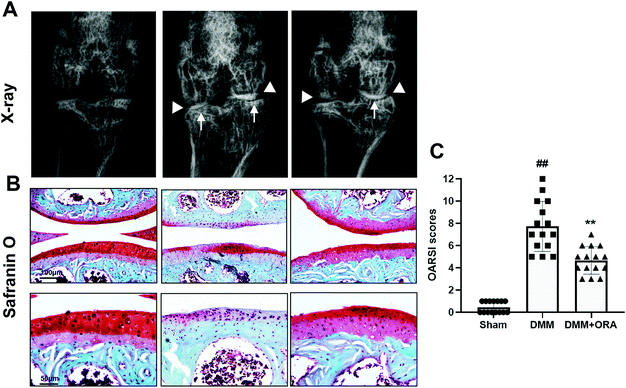 |
| Fig. 6 ORA attenuates OA development in vivo. (A) Digital X-ray image of mouse knee joints from different experimental groups. Narrowing of joint space was found in both the OA and treatment groups (white triangles). The calcification of the cartilage surface was obviously shown in the OA group (white arrows). (B) Representative S–O staining of the cartilage from different experimental groups at eight weeks post-surgery. (C) The OARSI scores of different groups. The data in the figures represent the average ± SD. Significant differences among the different groups are indicated as ##P < 0.01 and **P < 0.01. | |
4. Discussion
In recent years, OA has carried increasing medical burdens due to population aging and obesity.1,2 Epidemiological investigation revealed that 9.6% of men and 18.0% of women over 60-year-old have symptomatic OA.3 Although social, economic, and health problems of OA are seriously urgent, currently, it is still a medical conundrum without effective remedy except for total joint replacement for late-stage patients.34,35 Early intervention of OA is highly advocated by “stepped care strategy”.36 Clinically, Non-Steroidal Anti-Inflammatory Drugs (NSAIDs) are widely used as a first-line treatment due to their remarkable effects on pain management.37 However, NSAIDs are not able to delay the disease development, and the patients themselves may suffer some side effects such as dyspepsia, gastric risk, and thrombus formation.37,38 What is more, although glucosamine has applied for millions of OA patients, its place in the treatment of osteoarthritis needs more in-depth research.39 Therefore, effective agent treatments, which can significantly improve OA in terms of etiology with high biosecurity, are strongly needed.
ORA is an active ingredient of Oroxylum indicum and can be obtained by dietary consumption.23,24 A great deal of research showed that ORA exerts the anti-inflammatory effects on acute lung injury, liver injury, hypertrophy, tumor, neurodegenerative diseases, etc.25,28,40–42 However, whether ORA shows protective effects against OA and the exact underlying mechanisms are still under investigation. Therefore, in this study, we focused on solving out this problem.
Pro-inflammatory factors, such as IL-1β, are thought to be key triggers contributing to the pathological process of OA.43 A number of enzymes and mediators involved in the pathophysiology of OA can be induced by IL-1β such as TNF-a, IL-6, iNOS, and COX-2, which take an important part in the progression of OA.7,31 iNOS and COX-2 are two enzymes that induce the production of NO and PGE2, respectively.44 NO acts as a mediator to cause ECM degradation by upregulating matrix metalloproteinases (MMPs) and inhibiting collagen and proteoglycan synthesis.45 Like NO, PGE2 also contributes to the pathogenesis of OA by upregulating MMPs and ADAMTS, followed by enhancement of the degradation of the cartilage matrix components.46 Both MMPs and ADAMTS can cause the degradation of type II collagen and aggrecan, thus destroying the ECM.47 Moreover, under the long-term chronic inflammatory stimulation, chondrocytes tend to suffer phenotypic alterations.48 The RUNX-2 gene would be highly elevated in hypertrophic chondrocytes, which results in the secretion of type X collagen and MMP13, and consequently lead to the calcification of the cartilage.8 In the current study, ORA was shown to inhibit the imbalance of ECM homeostasis, which not only increases collagen II and aggrecan synthesis but also decreases the level of MMP13 and ADAMTS-5. Meanwhile, it was demonstrated that chondrocyte inflammation would be attenuated by ORA according to the dose-dependent decrease in the TNF-α, IL-6, PGE2, NO, COX-2 and iNOS levels. What is more, the hypertrophy markers of chondrocytes including the RUNX-2, collagen X and ALP levels were also down-regulated by ORA treatment in a dose-dependent manner. Hence, according to the results, we concluded that ORA inhibits the degeneration of IL-1β induced chondrocytes by mitigating the inflammatory response and hypertrophic transition.
Furthermore, the exact protective mechanism of ORA against OA needs to be explored. To investigate its anti-inflammatory effects, we firstly examined the NF-κB pathway activation, which is crucial inflammatory signaling for OA.11 Our research showed that ORA inhibited IL-1β-induced NF-κB activation in human chondrocytes by inhibiting IκBα degradation and rendering p65 nuclear translocation. For upstream target exploration, three potential targets (IKKβ, proteome, and p50) were mostly studied.14 In mammalian cells, NF-κB exists in an inactive form in the cytosol and is associated with inhibitory IκB proteins. IKKβ phosphorylation is necessary for the activation of the canonical pathway, which phosphorylates IκBα and marks them out for destruction, thereby relieving the translocation of NF-κB into the nucleus.49 Thus, IKKβ is the first core inhibitory target for the NF-κB axis. Then, the 26S proteasome is the crucial site for intracellular protein degradation, which also mediates IκB degradation in the NF-κB cascades and thereby promotes the nuclear translocation of NF-κB.14 Pharmacological inhibition of the proteasome–ubiquitin system by targeting proteasomes, E1/E2 enzymes, and E3 ubiquitin ligases is recognized as another practical approach for NF-κB inhibition. Moreover, canonical NF-κB activation needs p50/p65 homodimer formation, and thus inhibition of the p50/p65 interaction is also worth considering in drug discovery.14 Herein, the affinity of ORA to bind to these target molecules and interactions were screened by molecular docking analysis. The calculated scores indicated that ORA has the highest binding affinity to IKKβ compared to proteome and p50. Thus, the potential mechanism behind the inhibitory effects of ORA on the NF-κB axis might be related to targeting IKKβ.
To investigate the underlying mechanism of ORA in attenuating hypertrophic changes of chondrocytes, the Wnt/β-Catenin axis had been examined. This signaling emerged as a fundamental growth control pathway and is closely related to degenerative diseases and cancer.50 Plenty of research has proved that Wnt signaling is critically involved in the pathological process of OA.15–17In vitro, IL-1β has been demonstrated to successfully activate Wnt/β-catenin signaling.21 However, currently, our study has suggested that ORA treatment could decrease the level of β-catenin in both the cytoplasm and nucleus. Similarly, the potential targets were also detected by docking studies. There are also three usually studied inhibitory targets which are Dvl2, LEF-1, and TCF-4.51–53 Dvl2 is the upstream molecule which is responsible for GSK-3β inhibition, and thus help β-catenin to escape from the destructive complex to translocate into the nucleus.18 At the same time, LEF-1 and TCF-4 are the nuclear binding sites of β-catenin for its transcriptional activation.53 Thus, inhibiting the interaction between β-catenin and LEF-1 or TCF-4 is helpful for Wnt signaling inactivation. After the calculation, although there was no significant difference between the binding of Dvl2 and β-catenin/LEF-1interaction, the highest binding score was found for the Dvl2 docking model. Considering the western blot results, which revealed the significantly decreased level of β-catenin in the nucleus, the possible target of ORA on Wnt signaling might be associated with the inhibition of Dvl2.
The mouse model of DMM has been widely used to mimic human OA. In the present study, the mice showed cartilage calcification, hypocellularity, and loss of glycosaminoglycan. However, ORA administration could mitigate these detrimental phenomena, and the OARSI score is consistent with this. These data indicated that ORA is a promising therapeutic in ameliorating the development of OA in vivo. Additionally, a mouse dose of 10 mg kg−1 is equal to a human dose of 0.81 mg kg−1, that is, 48.6 mg of ORA for a human weighing 60 kg.54 As the stem bark of Oroxylum indicum contains approximately 1800 mg kg−1 ORA,55 48.6 mg of ORA is equivalent to 27 g of stem bark for a human weighing 60 kg. Therefore, those data indicated that the effective dosage of ORA could be achieved via dietary intake.
5. Conclusions
In the current study, we demonstrated that ORA significantly inhibited IL-1β induced ECM degradation by inhibiting cell inflammation and hypertrophy in human chondrocytes. The potential mechanism might be associated with dual inhibition of the NF-κB and Wnt/β-catenin axes. Meanwhile, the molecular simulation indicated that the underlying molecular targets might be IKKβ and Dvl2, respectively. The chondroprotective effects of ORA were also observed in a surgically induced OA mouse model. These results support the potential use of ORA as an effective therapeutic agent against OA.
Conflicts of interest
The authors declare that there are no conflicts of interest.
Acknowledgements
The funding of this project was provided by the General Project of Education Department of Zhejiang Province (Y202043146), and Wenzhou Science and Technology Planning Project (Y20190520).
References
- S. Glyn-Jones, A. Palmer, R. Agricola, A. Price, T. Vincent, H. Weinans and A. J. Carr, Osteoarthritis, Lancet, 2015, 386, 376–387 CrossRef CAS.
- WHO Scientific group, The burden of musculoskeletal conditions at the start of the new millennium, W. H. O. Tech. Rep. Ser., 2003, 919, 1–218 Search PubMed.
- T. Neogi and Y. Zhang, Epidemiology of osteoarthritis, Rheum. Dis. Clin. North. Am., 2013, 39, 1–19 CrossRef PubMed.
- B. Heidari, Knee osteoarthritis prevalence, risk factors, pathogenesis and features: Part I, Caspian J. Intern. Med., 2011, 2, 205–212 Search PubMed.
- V. K. Srikanth, J. L. Fryer, G. Zhai, T. M. Winzenberg, D. Hosmer and G. Jones, A meta-analysis of sex differences prevalence, incidence and severity of osteoarthritis, Osteoarthr. Cartil., 2005, 13, 769–781 CrossRef PubMed.
- M. B. Goldring and M. Otero, Inflammation in osteoarthritis, Curr. Opin. Rheumatol., 2011, 23, 471–478 CrossRef CAS PubMed.
- K. S. Santangelo, G. J. Nuovo and A. L. Bertone, In vivo reduction or blockade of interleukin-1beta in primary osteoarthritis influences expression of mediators implicated in pathogenesis, Osteoarthr. Cartil., 2012, 20, 1610–1618 CrossRef CAS PubMed.
- Q. Tang, G. Zheng, Z. Feng, M. Tong, J. Xu, Z. Hu, P. Shang, Y. Chen, C. Wang and Y. Lou, Wogonoside inhibits IL-1β induced catabolism and hypertrophy in mouse chondrocyte and ameliorates murine osteoarthritis, Oncotarget, 2017, 8, 61440 CrossRef PubMed.
- Z. Jenei-Lanzl, A. Meurer and F. Zaucke, Interleukin-1β signaling in osteoarthritis–chondrocytes in focus, Cell. Signalling, 2019, 53, 212–223 CrossRef CAS PubMed.
- E. Hedbom and H. J. Hauselmann, Molecular aspects of pathogenesis in osteoarthritis: the role of inflammation, Cell. Mol. Life Sci., 2002, 59, 45–53 CrossRef CAS PubMed.
- K. B. Marcu, M. Otero, E. Olivotto, R. M. Borzi and M. B. Goldring, NF-kappaB signaling: multiple angles to target OA, Curr. Drug Targets, 2010, 11, 599–613 CrossRef CAS PubMed.
- Y. Yamamoto and R. B. Gaynor, IkappaB kinases: key regulators of the NF-kappaB pathway, Trends Biochem. Sci., 2004, 29, 72–79 CrossRef CAS PubMed.
- P. Viatour, M. P. Merville, V. Bours and A. Chariot, Phosphorylation of NF-kappaB and IkappaB proteins: implications in cancer and inflammation, Trends Biochem. Sci., 2005, 30, 43–52 CrossRef CAS PubMed.
- S. Cheemanapalli, N. Chinthakunta, N. M. Shaikh, V. Shivaranjani, R. R. Pamuru and S. K. Chitta, Comparative binding studies of curcumin and tangeretin on up-stream elements of NF-kB cascade: a combined molecular docking approach, Netw. Model. Anal. Health Inform. Bioinform., 2019, 8, 15 CrossRef.
- N. Sassi, L. Laadhar, M. Allouche, A. Achek, M. Kallel-Sellami, S. Makni and S. Sellami, WNT signaling and chondrocytes: from cell fate determination to osteoarthritis physiopathology, J. Recept. Signal Transduction Res., 2014, 34, 73–80 CrossRef CAS PubMed.
- A. Stampella, S. Monteagudo and R. Lories, Wnt signaling as target for the treatment of osteoarthritis, Best Pract. Res., Clin. Rheumatol., 2017, 31, 721–729 CrossRef PubMed.
- Y. Zhou, T. Wang, J. L. Hamilton and D. Chen, Wnt/beta-catenin Signaling in Osteoarthritis and in Other Forms of Arthritis, Curr. Rheumatol. Rep., 2017, 19, 53 CrossRef PubMed.
- J. Shan, D. L. Shi, J. Wang and J. Zheng, Identification of a specific inhibitor of the dishevelled PDZ domain, Biochemistry, 2005, 44, 15495–15503 CrossRef CAS PubMed.
- G. Hahne and T. N. Grossmann, Direct targeting of β-catenin: Inhibition of protein–protein interactions for the inactivation of Wnt signaling, Bioorg. Med. Chem., 2013, 21, 4020–4026 CrossRef CAS PubMed.
- A. Rojas, R. Mardones, K. Pritzker, A. J. van Wijnen, M. A. Galindo and F. Las Heras, Dickkopf-1 reduces hypertrophic changes in human chondrocytes derived from bone marrow stem cells, Gene, 2019, 687, 228–237 CrossRef CAS PubMed.
- G. Zhong, R. Liang, J. Yao, J. Li, T. Jiang, J. Liu, Y. Le, C. Shen, H. Long, H. Lu, S. Ma, J. Luo, Z. Miao, W. Su, L. Zheng and J. Zhao, Artemisinin Ameliorates Osteoarthritis by Inhibiting the Wnt/beta-Catenin Signaling Pathway, Cell. Physiol. Biochem., 2018, 51, 2575–2590 CrossRef CAS PubMed.
- R. C. Gupta, V. Sharma, N. Sharma, N. Kumar and B. Singh, In vitro antioxidant activity from leaves of Oroxylum indicum (L.) Vent.-A North Indian highly threatened and vulnerable medicinal plant, J. Pharm. Res., 2008, 1, 65–72 Search PubMed.
- R. Lawania, A. Mishra and R. Gupta, Oroxylum indicum: a review, Pharmacogn. J., 2010, 2, 304–310 CrossRef.
- L. Lu, Q. Guo and L. Zhao, Overview of oroxylin A: a promising flavonoid compound, Phytother. Res., 2016, 30, 1765–1774 CrossRef CAS PubMed.
- H. Huang, X. Zhang and J. Li, Protective effect of oroxylin A against lipopolysaccharide and/or D-galactosamine-induced acute liver injury in mice, J. Surg. Res., 2015, 195, 522–528 CrossRef CAS PubMed.
- T. Ni, Z. He, Y. Dai, J. Yao, Q. Guo and L. Wei, Oroxylin A suppresses the development and growth of colorectal cancer through reprogram of HIF1alpha-modulated fatty acid metabolism, Cell Death Dis., 2017, 8, e2865 CrossRef CAS PubMed.
- T. L. Tseng, M. F. Chen, M. J. Tsai, Y. H. Hsu, C. P. Chen and T. J. Lee, Oroxylin-A rescues LPS-induced acute lung injury via regulation of NF-kappaB signaling pathway in rodents, PLoS One, 2012, 7, e47403 CrossRef CAS PubMed.
- J. Yao, R. Hu, J. Sun, B. Lin, L. Zhao, Y. Sha, B. Zhu, Q. D. You, T. Yan and Q. L. Guo, Oroxylin A prevents inflammation-related tumor through down-regulation of inflammatory gene expression by inhibiting NF-kappaB signaling, Mol. Carcinog., 2014, 53, 145–158 CrossRef CAS PubMed.
- Y.-l. Wang, J.-M. Gao and L.-Z. Xing, Therapeutic potential of Oroxylin A in rheumatoid arthritis, Int. Immunopharmacol., 2016, 40, 294–299 CrossRef CAS PubMed.
- G. A. O. T. W. M. Association, World Medical Association Declaration of Helsinki: ethical principles for medical research involving human subjects, J. Am. Coll. Dent., 2014, 81, 14 Search PubMed.
- Q. Tang, Z. Feng, M. Tong, J. Xu, G. Zheng, L. Shen, P. Shang, Y. Zhang and H. Liu, Piceatannol inhibits the IL-1β-induced inflammatory response in human osteoarthritic chondrocytes and ameliorates osteoarthritis in mice by activating Nrf2, Food Funct., 2017, 8, 3926–3937 RSC.
- S. Glasson, T. Blanchet and E. J. O. Morris, The surgical destabilization of the medial meniscus (DMM) model of osteoarthritis in the 129/SvEv mouse, Osteoarthr. Cartil., 2007, 15, 1061–1069 CrossRef CAS PubMed.
- S. Glasson, M. Chambers, W. Van Den Berg and C. Little, The OARSI histopathology initiative–recommendations for histological assessments of osteoarthritis in the mouse, Osteoarthr. Cartil., 2010, 18, S17–S23 CrossRef PubMed.
- M. Kloppenburg and F. Berenbaum, Osteoarthritis year in review 2019: epidemiology and therapy, Osteoarthr. Cartil., 2020, 28, 242–248 CrossRef CAS PubMed.
- M. Khalid, S. Tufail, Z. Aslam and A. J. I. J. O. C. R. Butt, Osteoarthritis: From complications to cure, Int. J. Clin. Rheumatol., 2017, 12, 160 Search PubMed.
- A. J. Smink, C. H. van den Ende, T. P. V. Vlieland, B. A. Swierstra, J. H. Kortland, J. W. Bijlsma, T. B. Voorn, H. J. Schers, S. M. Bierma-Zeinstra and J. Dekker, “Beating osteoARThritis”: development of a stepped care strategy to optimize utilization and timing of non-surgical treatment modalities for patients with hip or knee osteoarthritis, Clin. Rheumatol., 2011, 30, 1623–1629 CrossRef PubMed.
- C. Ding, Do NSAIDs affect the progression of osteoarthritis?, Inflammation, 2002, 26, 139–142 CrossRef CAS PubMed.
- K. H. Maniar, I. A. Jones, R. Gopalakrishna and C. T. Vangsness Jr., Lowering side effects of NSAID usage in osteoarthritis: recent attempts at minimizing dosage, Expert Opin. Pharmacother., 2018, 19, 93–102 CrossRef CAS PubMed.
- C. A. Heyneman and R. S. Rhodes, Glucosamine for osteoarthritis: cure or conundrum?, Ann. Pharmacother., 1998, 32, 602–603 CrossRef CAS PubMed.
- M. Ye, Q. Wang, W. Zhang, Z. Li, Y. Wang and R. Hu, Oroxylin A exerts anti-inflammatory activity on lipopolysaccharide-induced mouse macrophage via Nrf2/ARE activation, Biochem. Cell Biol., 2014, 92, 337–348 CrossRef CAS PubMed.
- P. W. Liu, M. F. Chen, A. P. Tsai and T. J. Lee, STAT1 mediates oroxylin a inhibition of iNOS and pro-inflammatory cytokines expression in microglial BV-2 cells, PLoS One, 2012, 7, e50363 CrossRef CAS PubMed.
- N. T. Saldaña, G. J. G. Rivas and L. L. E. Montemayor, Oroxylin A reduces angiotensin II-induced hypertrophy and mitochondrial dysfunction by activating sirtuin 3 in cardiac myocytes, J. Mol. Cell. Cardiol., 2017, 112, 148 CrossRef.
- M. Kobayashi, G. R. Squires, A. Mousa, M. Tanzer, D. J. Zukor, J. Antoniou, U. Feige and A. R. Poole, Role of interleukin-1 and tumor necrosis factor α in matrix degradation of human osteoarthritic cartilage, Arthritis Rheum., 2005, 52, 128–135 CrossRef CAS PubMed.
- P. Needleman and P. Manning, Interactions between the inducible cyclooxygenase (COX-2) and nitric oxide synthase (iNOS) pathways: implications for therapeutic intervention in osteoarthritis, Osteoarthr. Cartil., 1999, 7, 367–370 CrossRef CAS PubMed.
- S. B. Abramson, Osteoarthritis and nitric oxide, Osteoarthr. Cartil., 2008, 16, S15–S20 CrossRef.
- M. Attur, H. E. Al-Mussawir, J. Patel, A. Kitay, M. Dave, G. Palmer, M. H. Pillinger and S. B. Abramson, Prostaglandin E2 exerts catabolic effects in osteoarthritis cartilage: evidence for signaling via the EP4 receptor, J. Immunol., 2008, 181, 5082–5088 CrossRef CAS PubMed.
- C. R. Flannery, MMPs and ADAMTSs: functional studies, Front. Biosci., 2006, 11, 544–569 CrossRef CAS PubMed.
- E. G. J. Ripmeester, U. T. Timur, M. M. J. Caron and T. J. M. Welting, Recent insights into the contribution of the changing hypertrophic chondrocyte phenotype in the development and progression of osteoarthritis, Front. Bioeng. Biotechnol., 2018, 6, 18 CrossRef PubMed.
- J.-L. Wang, L. Li, M.-B. Hu, B. Wu, W.-X. Fan, W. Peng, D.-N. Wei and C.-J. Wu, In silico drug design of inhibitor of nuclear factor kappa B kinase subunit beta inhibitors from 2-acylamino-3-aminothienopyridines based on quantitative structure–activity relationships and molecular docking, Comput. Biol. Chem., 2019, 78, 297–305 CrossRef CAS PubMed.
- B. T. MacDonald, K. Tamai and X. He, Wnt/β-catenin signaling: components, mechanisms, and diseases, Dev. Cell, 2009, 17, 9–26 CrossRef CAS PubMed.
- Y. Zhang, B. A. Appleton, C. Wiesmann, T. Lau, M. Costa, R. N. Hannoush and S. S. Sidhu, Inhibition of Wnt signaling by Dishevelled PDZ peptides, Nat. Chem. Biol., 2009, 5, 217–219 CrossRef CAS PubMed.
- N. Polachi, B. Subramaniyan, P. Nagaraja, K. Rangiah and M. Ganeshan, Extract from Butea monosperma inhibits β-catenin/Tcf signaling in SW480 human colon cancer cells, Gene Rep., 2018, 10, 79–89 CrossRef.
- G. Hahne and T. N. Grossmann, Direct targeting of beta-catenin: Inhibition of protein-protein interactions for the inactivation of Wnt signaling, Bioorg. Med. Chem., 2013, 21, 4020–4026 CrossRef CAS PubMed.
- A. B. Nair and S. Jacob, A simple practice guide for dose conversion between animals and human, J. Basic Clin. Pharm., 2016, 7, 27 CrossRef PubMed.
- R. Arimboor, K. Salini and C. Arumughan, HPTLC estimation of oroxylin A in Oroxylum indicum herbal formulations, J. Planar Chromatogr.–Mod. TLC, 2013, 26, 274–278 CrossRef CAS.
Footnote |
† These authors contributed equally to this work. |
|
This journal is © The Royal Society of Chemistry 2021 |