DOI:
10.1039/C9FO02237F
(Paper)
Food Funct., 2020,
11, 711-721
Baicalein reduces hepatic fat accumulation by activating AMPK in oleic acid-induced HepG2 cells and high-fat diet-induced non-insulin-resistant mice
Received
24th September 2019
, Accepted 18th December 2019
First published on 19th December 2019
Abstract
Nonalcoholic fatty liver disease (NAFLD) has become the most common liver disease worldwide; thus, a dietary supplement that can restrict hepatic fat accumulation is needed. Baicalein, a major component of Scutellaria baicalensis, is used as a dietary supplement in Eastern and Western cultures and can reduce hepatic fat accumulation. However, the detailed mechanism by which baicalein exerts this effect has yet to be elucidated in vivo and in vitro. In this study, we characterized the hepatic fat-lowering activity of baicalein and found that baicalein reduced hepatic fat accumulation by activating AMPK and suppressing SREBP1 cleavage, thus consequently inhibiting the transcriptional activity of SREBP1 and the synthesis of hepatic fat in oleic acid-induced HepG2 cells and high-fat diet-induced non-insulin-resistant mice. Moreover, baicalein improved NAFLD by decreasing TC, increasing HDLC, decreasing LDLC, affecting antioxidant activity, and exerting other effects. Therefore, the mechanism of baicalein with regard to NAFLD prevention and treatment might involve effects on multiple targets and pathways. Our study supports the use of baicalein as a dietary supplement due to its ability to reduce hepatic fat accumulation and to ameliorate NAFLD-related biochemical abnormalities.
1. Introduction
Nonalcoholic fatty liver diseases (NAFLDs) include nonalcoholic simple fatty liver, nonalcoholic steatohepatitis and hepatocellular carcinoma.1 Recent studies revealed that approximately 20% to 30% of adults have NAFLD in Western countries. NAFLD has become the most common liver disease worldwide.2 Additionally, patients with NAFLD have a higher incidence of liver cancer, which is seriously harmful to human health and affects quality of life.3
Although the underlying mechanism of the development and progression of NAFLD is complex and multifactorial, which is known to be closely related to obesity, diabetes, genetics, malnutrition, the environment, etc.4 NAFLD is characterized by fat accumulation, ranging from simple accumulation to steatosis combined with varying degrees of inflammation and fibrosis.5 Therefore, directly limiting the accumulation of fats, especially fatty acids (the elementary components of fat) and triglycerides (TGs) in the liver, is a fundamental approach for NAFLD prevention and treatment. Fatty acids in the liver are mainly derived from the diet and de novo synthesis.6 The diet is the leading source of liver fatty acids, and dietary restriction is beneficial for NAFLD.7 However, for many severe NAFLD patients, diet restriction alone or in combination with lifestyle intervention, did not achieve favorable therapeutic benefits.8,9 A dietary supplement or drug intervention that could restrict the synthesis of fatty acids is also needed.
Flavonoids, a class of polyphenol secondary metabolites, are found in large quantities in fruits, vegetables, grains, cola, tea, coffee, cocoa, beer, and red wine.10 Baicalein (5,6,7-trihydroxyflavone, PubChem CID: 5281605) is a flavonoid found in Scutellaria baicalensis (also known as “Huangqin”). Decoctions and tinctures prepared from the root of S. baicalensis have been widely used for the prevention and treatment of fever, viral infections, bacterial infections, inflammation, and cancer.11–13 In addition to traditional formulations, baicalein is given as a dietary food supplement in Eastern and Western cultures.14 Baicalein is also considered a natural compound with low toxicity. Single oral doses of 100–2800 mg of baicalein were safe and well tolerated by healthy subjects.15 Despite a relatively high tolerated dose, the excessive uptake of baicalein is not advisable. The appropriate dose of baicalein depends on several factors, such as the user's age, health, and several other conditions. In our and other previous studies, baicalein was also reported to reduce hepatic fat in diabetic mice.16,17 However, the detailed mechanism through which baicalein reduces hepatic fat in vivo and in vitro and improves NAFLD has yet to be elucidated.
In this study, we investigated the mechanism through which baicalein improved fat accumulation in vitro and in vivo and evaluated the preventive effects of baicalein on NAFLD in mice without diabetes and insulin resistance (IR) induced by a high-fat diet (HFD).
2. Materials and methods
2.1. Materials
Baicalein (purity, more than 98%; HPLC), isolated from a water–ethanol extract of S. baicalensis, was purchased from Nantong Feiyu Biological Technology Co., Ltd (Shanghai, China). The Western Bright ECL kit, Dulbecco's modified Eagle's medium (DMEM), hematoxylin, eosin, oil red O, antibiotics, Lipofectamine 3000, fetal bovine serum, and trypsin were purchased from Solarbio Co., Ltd (Beijing, China). Hygromycin B was purchased from Roche Co, Ltd (Basel, Switzerland). The luciferase assay kit and the luciferase reporter vector were purchased from Promega Co., Ltd (Beijing, China). Sodium carboxymethylcellulose (CMC-Na) and other chemical reagents were purchased from Damao Co., Ltd (Tianjin, China). The insulin kit was purchased from Cusabio (Wuhan, China). The membrane protein extraction kit, the BCA protein assay kit and the kits used to measure the level of glucose (glucose oxidase method), glycogen, aspartate transaminase (AST), alanine transaminase (ALT), superoxide dismutase (SOD), total antioxidative capacity (T-AOC), glutathione peroxidase (GPx), TG, total cholesterol (TC), high-density lipoprotein cholesterol (HDLC), and low-density lipoprotein cholesterol (LDLC) were purchased from Jiancheng Institute of Biotechnology (Nanjing, China). The polyvinylidene fluoride (PVDF) membrane was purchased from Millipore Co., Ltd (Massachusetts, USA). HepG2 (human hepatocellular carcinoma) cells were obtained from the American Type Culture Collection (Maryland, USA).
Primary antibodies against adenosine 5′-monophosphate (AMP)-activated protein kinase (AMPK; 1
:
1000, PA555883), phospho-AMPK (T183/172) (pAMPK; 1
:
1000, PA247163), precursor sterol regulatory element-binding protein 1 (pSREBP1; 1
:
1000, PA005992), and phospho-SREBP1 (1;1000, PA050140) were purchased from Cusabio Technology Co., Ltd (Wuhan, China). Primary antibodies against acetyl coenzyme a carboxylase (ACC; 1
:
1000, bs2745R), phospho-ACC (Ser79) (pACC; 1
:
1000, bs3039R), fatty acid synthase (FASN; 1
:
1000, bs1498R), and mature SREBP1 (mSREBP1; 1
:
1000, bs1402R) were purchased from Bioss Technology Co., Ltd (Beijing, China). Primary antibodies against β-actin (1
:
1000, K200058 M) and secondary antibodies were purchased from Solarbio Co., Ltd (Beijing, China).
Male Kunming mice (16–20 g) were purchased from Shandong Laboratory Animal Center (Jinan, China) with the permission number SCXK 2014-0007. The animal husbandry system was maintained under standard laboratory conditions. All animal procedures were performed in accordance with the Guidelines for Care and Use of Laboratory Animals of Shandong University of Technology and approved by the Animal Ethics Committee of Shandong University of Technology.
2.2.
In vitro studies
2.2.1. Cell culture.
HepG2 cells were cultured in DMEM supplemented with 10% heat-inactivated fetal bovine serum and antibiotics (hereafter referred to as cell culture medium) in 75 cm2 tissue culture flasks in 5% humidified CO2 at 37 °C. The medium was routinely changed every 3 days, and the cells were passaged by trypsinization before reaching confluency. A fatty HepG2 cell model was induced by oleic acid according to the method reported previously with slight modifications.18 Briefly, HepG2 cells were treated with 1 mM oleic acid to induce excessive fat synthesis, and the differentiation of HepG2 cells was confirmed by TC and TG tests after oleic acid treatment.
2.2.2. Measurement of intracellular TC and TG.
The cytotoxicity of baicalein at a range of doses (0 μM, 0.01 μM, 0.05 μM, 0.1 μM, 0.5 μM, 1 μM, 3 μM, 5 μM, 7.5 μM, 10 μM, 12.5 μM, 15 μM, 17.5 μM, and 20 μM) was measured by MTT colorimetric assay. Following 12 h of starvation, the fatty HepG2 cells were washed with PBS and treated with DMSO (negative control) or a range of baicalein doses (l μM and 5 μM) for 24 h. Then, the TC and TG of the cells were determined by a commercial kit. The analyses were performed in 6 replicate wells, and each experiment was repeated 3 times.
2.2.3 SREBP1 transcriptional activity assay.
The transcriptional activity of SREBP1 was measured as previously described with slight modifications.19 Briefly, three tandem SREBP1 binding sites, 5′-AAAATCACCCCACTGCAAACTCCTCCCCCTGC-3′, were inserted into pGL4.26 by primer annealing to generate the SREBP1 reporter plasmid pSRE-Luc. HepG2 cells were cotransfected with pSRE-Luc and the control Renilla vector pRL-TK by using Lipofectamine 3000. After 24 h, the cells were switched to culture medium with 200 lg mL−1 hygromycin B. The medium was refreshed every three days for approximately two weeks until colonies formed. Individual colonies were visually identified and isolated with cloning cylinders. The cells were treated with a range of baicalein doses (0 μM, l μM, and 5 μM) and with 1 mM oleic acid for 24 h. SREBP1 transcriptional activity was measured using a luciferase assay kit.
2.2.4. Immunoblot analysis in vitro.
HepG2 cells were treated with 1 mM oleic acid to induce excessive fat synthesis. The fatty HepG2 cells received either DMSO or baicalein (1 μM or 5 μM) for 24 h (for pAMPK, pACC, and phospho-SREBP: 1 h, 1 h, and 6 h, respectively). After the treatment, the cells were washed with PBS and harvested by gentle scraping and centrifugation at 2000g and 4 °C for 3 min. The protein was extracted using the protein extraction kit with a lysis buffer containing 1 mM Na3VO4 and 0.1 mM phenylmethanesulfonyl fluoride. The protein concentration of each sample was determined by BCA assay. For AMPK, pAMPK, mSREBP1, and β-actin, the protein samples were separated by 12% SDS-polyacrylamide gel electrophoresis (SDS-PAGE). The protein samples were then transferred onto a PVDF membrane and blocked with 5% skim milk for 4 h at room temperature. The membrane was incubated with primary antibodies diluted in Tris-buffered saline with Tween-20 (TBST) containing 2.5% skim milk at 4 °C overnight. The immune complex was recognized by a secondary antibody conjugated to horseradish peroxidase (1
:
1000 in TBST). The peroxidase activity was visualized using an ECL kit. The densitometric analysis of the immunoblots was performed using a gel documentation system. For the detection of pSREBP1, phospho-SREBP, ACC, pACC, and FASN, the protein samples were separated by 8% SDS-PAGE.
2.2.5. Inhibition of AMPK phosphorylation.
Following 12 h of starvation, the fatty HepG2 cells were treated with 10 μM compound C (dorsomorphin) or DMSO for 1 h. Then, fatty HepG2 cells incubated with compound C or DMSO were treated with 5 μM baicalein or DMSO. The TG and TC levels of the cells were also measured following the procedures described in section 2.2.2. The SREBP transcriptional activity was determined following the procedures described in section 2.2.3. The expression of phospho-SREBP1, pSREBP1, mSREBP1, ACC, FASN, and β-actin were determined following the procedures described in section 2.2.4. The analyses were performed in six replicate wells, and each experiment was repeated 3 times.
2.3.
In vivo studies
2.3.1. Establishment of the NAFLD mouse model and experimental groups.
NAFLD mice were induced with an HFD. The HFD contained 36.4% starch, 25.6% butter, 20% protein, 1% cholesterol and 0.1% bile acid. A total of 24 NAFLD mice were randomly administered vehicle (MD, 0.5% CMC-Na), 50 mg kg−1 d−1 baicalein (LB), or 200 mg kg−1 d−1 baicalein (HB) by gavage for 3 months (n = 8). Another 8 mice were fed a normal diet and used as the negative control (NM).
2.3.2. Measurement of body weight, blood lipid profiles, and oxidative stress and the tissue function assay.
Food intake was measured during the last week of the in vivo studies. All mice were fasted and had free access to water for 16 h before sacrifice. The body weights were measured, and the blood samples were collected and centrifuged at 4000g for 5 min at 4 °C to obtain the plasma. The fasting insulin (FINS), fasting plasma glucose (FPG), 2 h-postprandial glucose (2h-PG), and homeostasis model assessment-IR (HOMA-IR, HOMA-IR = FPG × FINS/22.5) were assayed using a commercially available assay kit. The livers were immediately excised, collected and stored in liquid nitrogen for oxidative stress analysis or in 10% formalin for histological studies. The levels of TC, TG, LDLC, and HDLC in the plasma and the levels of TC and TG in the liver were measured with commercially available kits. The levels of SOD, MDA, GPx, and T-AOC in hepatic tissues were assayed to evaluate antioxidant capacity using commercially available kits. The levels of ALT and AST in the plasma were also measured by commercially available kits.
2.3.3. Immunoblot analysis in vitro.
For the immunoblot analyses, the abundance of AMPK, pAMPK, phospho-SREBP1, pSREBP1, mSREBP1, ACC, FASN, pACC and β-actin in the livers excised from the NM, MD, LB and HB groups was analyzed as described above.
2.3.4. Histological study.
For the histological analyses, tissue samples were fixed in formalin solution for 24 h and then embedded in paraffin. Sections (3 μm thick) were stained with hematoxylin–eosin. In addition, cryosections of livers were stained with oil red O and counterstained with hematoxylin to visualize the lipid droplets. The stained samples were observed with an optical microscope at 40× or 200× magnification.
2.4. Statistical analysis
Statistical analysis was performed using SPSS 17.0, and the results are expressed as the mean ± standard deviation for in vitro experiments and the mean ± standard error for in vivo experiments. Significant differences (*P < 0.05, **P < 0.01 and ***P < 0.001) were analyzed using one-way ANOVA followed by the least significant difference (LSD) test for multiple comparisons.
3. Results
3.1.
In vitro studies
3.1.1. Effects of baicalein on intracellular TC and TG.
To study the hepatic fat-lowering effect of baicalein, we established a fatty HepG2 cell model by treating the cells with high levels of oleic acid. Furthermore, we measured the TG and TC levels in fatty HepG2 cells to characterize the effects of baicalein on hepatic fat accumulation. After a 24 h treatment, baicalein decreased the TG and TC levels in HepG2 cells in a dose-dependent manner. Baicalein (5 μM) decreased TG and TC to 44.9% and 30.9%, respectively (Fig. 1A and B).
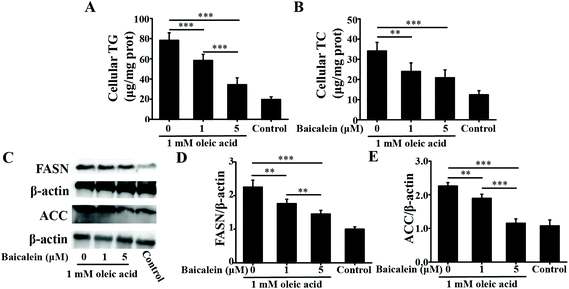 |
| Fig. 1 Effects of baicalein on cellular TG, TC, FASN and ACC in oleic acid-induced fatty HepG2 cells. A: Levels of cellular TG. B: Levels of cellular TC. C: Immunoblot analysis of FASN and ACC. D: Densitometry analysis of FASN. E: Densitometry analysis of ACC. The values are shown as the means ± standard deviations, n = 3 for each group. Significant differences (*P < 0.05, **P < 0.01 and ***P < 0.001) were analyzed using one-way ANOVA followed by the LSD test for multiple comparisons. | |
3.1.2. Effects of baicalein on the abundance of ACC and FASN.
To further demonstrate the effects of baicalein on hepatic lipid synthesis, we determined the abundance of key lipid-synthesizing enzymes, ACC and FASN, in fatty HepG2 cells. After a 24 h treatment, baicalein decreased the abundance of ACC and FASN in a dose-dependent manner. Compared with 1 mM oleic acid alone, the abundance of ACC and FASN in HepG2 cells was decreased by 43.2% and 35.6%, respectively, following treatment with 5 μM baicalein and 1 mM oleic acid (Fig. 1C–E). Our data indicated that baicalein reduced lipid synthesis.
3.1.3. Effects of baicalein on SREBP1.
The effect of baicalein on the transcriptional activity of SREBP1 was assayed in HepG2 cells. Oleic acid significantly increased the SREBP1 transcription level and baicalein, at concentrations of 1 μM and 5 μM, significantly decreased the SREBP1 transcription level, with 5 μM baicalein exhibiting the strongest inhibition (Fig. 2A). Furthermore, we measured the abundance of phospho-SREBP1, pSREBP1, and mSREBP1 in fatty HepG2 cells to characterize the effects of baicalein on SREBP1. Over 24 h of treatment (for phospho-SREBP1: 6 h of treatment), baicalein increased the phosphorylation of SREBP1 and the abundance of pSREBP1 and decreased the abundance of mSREBP1 in fatty HepG2 cells in a dose-dependent manner (Fig. 2B–E).
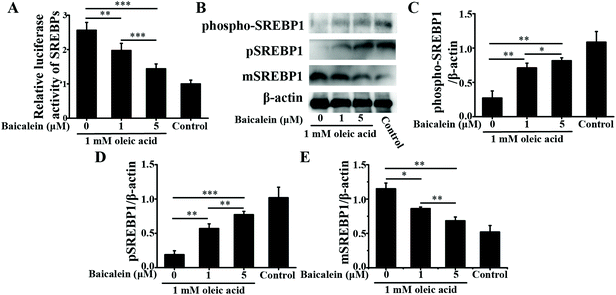 |
| Fig. 2 Effects of baicalein on the cleavage and nuclear transcriptional activity of SREBP1 in oleic acid-induced fatty HepG2 cells. A: Transcriptional activity of SREBP1. B: Immunoblot analysis of phospho-SREBP1, pSREBP1 and mSREBP1. C: Densitometry analysis of phospho-SREBP1. D: Densitometry analysis of pSREBP1. E: Densitometry analysis of mSREBP1. The values are shown as the means ± standard deviations, n = 3 for each group. Significant differences (*P < 0.05, **P < 0.01 and ***P < 0.001) were analyzed using one-way ANOVA followed by the LSD test for multiple comparisons. | |
3.1.4. Effects of baicalein on the phosphorylation of AMPK.
To explain the mechanism by which baicalein reduces lipid synthesis, we measured the phosphorylation of AMPK. After a 1 h treatment, baicalein increased the phosphorylation of AMPK in a dose-dependent manner. Compared with 1 mM oleic acid alone, 5 μM baicalein and 1 mM oleic acid increased the phosphorylation of AMPK in HepG2 cells by 2.75-fold (Fig. 3A).
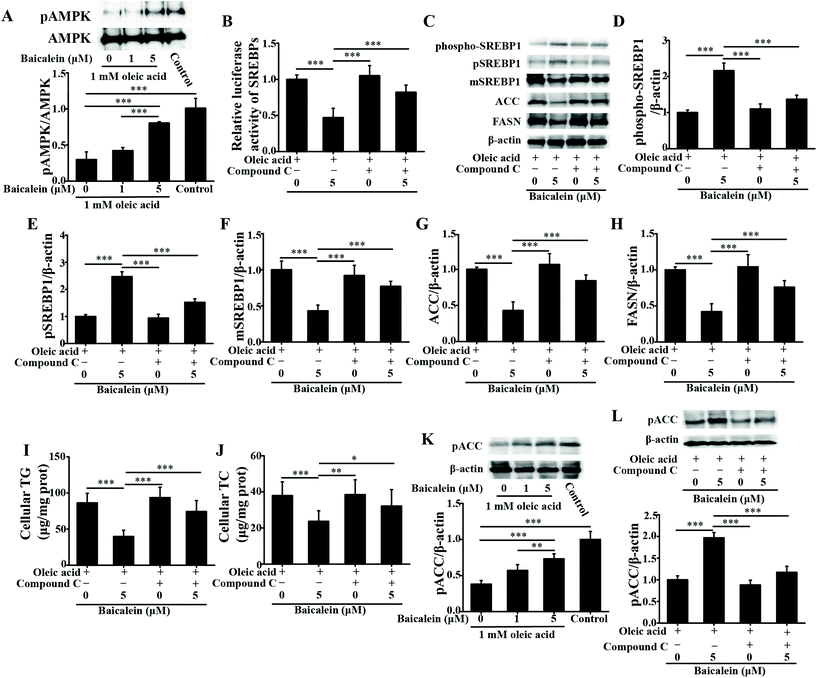 |
| Fig. 3 Effects of baicalein on AMPK and the downstream signaling pathway of AMPK in oleic acid-induced fatty HepG2 cells. A: Immunoblot (upper panel) and densitometry analysis (lower panel) of pAMPK/AMPK. B: Effect of compound C on the transcriptional activity of SREBP1 regulated by baicalein. C: Immunoblot analysis of the effect of compound C on phospho-SREBP1, pSREBP1, mSREBP1, FASN, and ACC regulated by baicalein. D: Densitometry analysis of the effect of compound C on phospho-SREBP1 regulated by baicalein. E: Densitometry analysis of the effect of compound C on pSREBP1 regulated by baicalein. F: Densitometry analysis of the effect of compound C on mSREBP1 regulated by baicalein. G: Densitometry analysis of the effect of compound C on FASN regulated by baicalein. H: Densitometry analysis of the effect of compound C on ACC regulated by baicalein. I: Effect of compound C on cellular TG regulated by baicalein. J: Effect of compound C on cellular TC regulated by baicalein. K: Immunoblot (upper panel) and densitometry analysis (lower panel) of pACC. L: Immunoblot (upper panel) and densitometry analysis (lower panel) of the effect of compound C on pACC regulated by baicalein. The values are shown as the means ± standard deviations, n = 3 for each group. Significant differences (*P < 0.05, **P < 0.01 and ***P < 0.001) were analyzed using one-way ANOVA followed by LSD test for multiple comparisons. | |
3.1.5. Effects of an AMPK phosphorylation inhibitor on the inhibitory activity of baicalein on lipid synthesis.
To further confirm that baicalein reduces lipid synthesis by regulating AMPK phosphorylation, we treated fatty HepG2 cells with DMSO (control), baicalein (5 μM), compound C (dorsomorphin, an AMPK phosphorylation inhibitor, 10 μM) or a combination of compound C and baicalein (Fig. 3B–J). Compared with baicalein treatment, the AMPK phosphorylation inhibitor significantly increased the SREBP1 transcription level, the abundance of mSREBP1, the abundance of ACC, and the abundance of FASN. Moreover, compound C significantly decreased the phosphorylation of SREBP1 and the abundance of pSREBP1. In addition, compared with baicalein, compound C significantly increased intracellular TC and TG levels. These data indicated that the phosphorylation of AMPK plays an important role in the hepatic fat-lowering effect of baicalein. In addition, baicalein increased the phosphorylation of ACC, and the AMPK phosphorylation inhibitor inhibited this increase (Fig. 3K and L).
3.2.
In vivo studies
3.2.1. Effects of baicalein on bodyweight and food intake.
Our data indicated that the bodyweights of the HFD groups were higher than those of the NM group, and the food intakes of the HFD groups were lower than those of the NM group. Moreover, the bodyweights and food intake levels were not different among the MD, LB, and HB groups (Table 1).
Table 1 The effect of baicalein on bodyweight, food intake, FPG, 2h-PG, FINS, and HOMA-IR in HFD-induced NAFLD mice
|
NM |
MD |
LB |
HB |
NM, Normal mice administered 0.5% CMC-Na; MD, NAFLD mice administered 0.5% CMC-Na; LB, NAFLD mice administered 50 mg kg−1 d−1 baicalein in 0.5% CMC-Na; HB, NAFLD mice administered 200 mg kg−1 d−1 baicalein in 0.5% CMC-Na. Values are shown as the mean ± standard error, n = 8 for each group. Significant differences (*P < 0.05, **P < 0.01 and ***P < 0.001 compared with NM group) were analyzed using one-way ANOVA followed by LSD test for multiple comparisons. |
Bodyweiht (g) |
43.58 ± 1.80 |
52.68 ± 5.59* |
51.68 ± 5.53* |
53.46 ± 8.26* |
Food intake (g d−1) |
5.41 ± 0.18 |
4.69 ± 0.34* |
4.83 ± 0.25* |
4.60 ± 0.20* |
FPG (mM) |
3.95 ± 0.25 |
4.82 ± 0.48 |
4.97 ± 0.21 |
4.76 ± 0.38 |
2h-PG (mM) |
4.80 ± 0.30 |
5.52 ± 0.52 |
5.67 ± 0.57 |
5.85 ± 0.52 |
FINS (mIU L−1) |
348.60 ± 24.66 |
368.47 ± 38.08 |
373.62 ± 57.93 |
365.42 ± 56.69 |
HOMA-IR |
62.06 ± 8.42 |
79.16 ± 12.22 |
81.84 ± 12.60 |
76.01 ± 13.34 |
3.2.2. Effects of baicalein on insulin sensitivity.
To investigate the effect of baicalein on insulin sensitivity in HFD-induced NAFLD mice, we measured the levels of blood glucose and insulin following the administration of baicalein. However, in our experiment, the HFD did not result in IR, and FPG, 2h-PG, FINS, and HOMA-IR showed no significant differences among all the groups (Table 1).
3.2.3 Effects of baicalein on plasma lipid profiles.
The levels of TC, TG and LDLC were increased significantly in the MD group compared with the NM group, and the level of HDLC decreased significantly after the HFD was administered for 3 months. When baicalein was given, the plasma lipid profile markedly improved, especially with high doses of baicalein (HB). The levels of TC, TG, and LDLC in the HB group were 33.5%, 30.8% and 36.5% lower than those in the MD group, respectively, and the HDLC level in the HB group was 41.9% higher than that in the MD group (Fig. 4A–D). These data indicated that baicalein improved plasma lipid profiles.
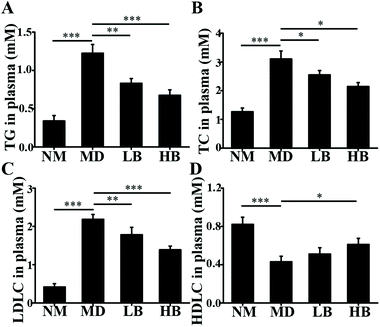 |
| Fig. 4 Effects of baicalein on the lipid profiles of HFD-induced NAFLD mice. A: Effects of baicalein on TG in plasma. B: Effects of baicalein on TC in plasma. C: Effects of baicalein on LDLC in plasma. D: Effects of baicalein on HDLC in plasma. NM, Normal mice administered 0.5% CMC-Na; MD, NAFLD mice administered 0.5% CMC-Na; LB, NAFLD mice administered 50 mg kg−1 d−1 baicalein in 0.5% CMC-Na; HB, NAFLD mice administered 200 mg kg−1 d−1 baicalein in 0.5% CMC-Na. The values are shown as the means ± standard errors, n = 8 for each group. Significant differences (*P < 0.05, **P < 0.01 and ***P < 0.001) were analyzed using one-way ANOVA followed by LSD test for multiple comparisons. | |
3.2.4. Effects of baicalein on hepatic lipid profiles.
The levels of hepatic TC and TG were significantly increased in the MD group after an HFD was administered for 3 months compared with the NM group. When baicalein was given, the hepatic lipid profile markedly improved. The levels of TG and TC in the HB group were 64.9% and 51.6% lower than those in the MD group, respectively (Fig. 5A and B). These data indicated that baicalein improved hepatic lipid profiles.
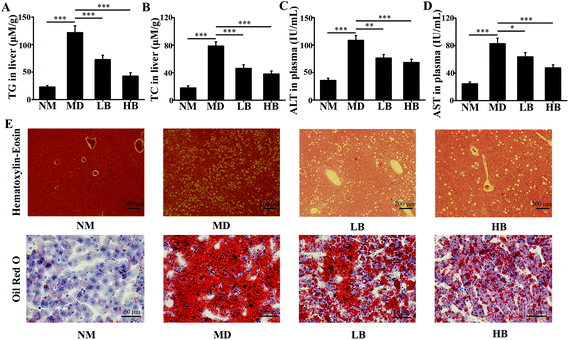 |
| Fig. 5 Effects of baicalein on liver lipid profiles, function, architecture, and morphology of the liver in HFD-induced NAFLD mice. A: Effect of baicalein on TG in the liver. B: Effects of baicalein on TC in the liver. C: Effect of baicalein on ALT in plasma. D: Effect of baicalein on AST in plasma. E: Effect of baicalein on liver histology. NM, Normal mice administered 0.5% CMC-Na; MD, NAFLD mice administered 0.5% CMC-Na; LB, NAFLD mice administered 50 mg kg−1 d−1 baicalein in 0.5% CMC-Na; HB, NAFLD mice administered 200 mg kg−1 d−1 baicalein in 0.5% CMC-Na. The values are shown as the means ± standard errors, n = 8 for each group. Significant differences (*P < 0.05, **P < 0.01 and ***P < 0.001) were analyzed using one-way ANOVA followed by LSD test for multiple comparisons. | |
3.2.5. Effects of baicalein on the function, architecture and morphology of the liver.
For liver function, compared to the MD group, the baicalein-treated groups showed significantly increased the ALT and AST levels (Fig. 5C and D). For liver architecture morphology, the histological evaluation of liver specimens showed cellular swelling and large and/or small hollow spaces in the MD group compared with the NM group (Fig. 5E). The livers treated with baicalein had noticeably improved morphology and architecture of the liver. Low levels of cellular swelling or hepatic steatosis were observed in the baicalein-treated groups. These results indicated that baicalein improved the architecture, morphology and function of the liver.
3.2.6. Effect of baicalein on oxidative stress.
The T-SOD, MDA, T-AOC, and GPx values representing the level of oxidative stress in the liver were determined. Compared with the MD group, the baicalein-treated group showed significantly increased the levels of T-SOD, T-AOC and GPx and reduced MDA levels in the liver (Fig. 6A–D).
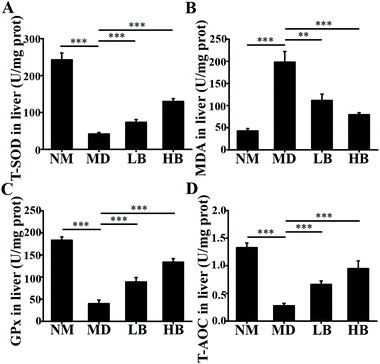 |
| Fig. 6 Effects of baicalein on oxidative stress in the livers of HFD-induced NAFLD mice. A: Effect of baicalein on T-SOD in the liver. B: Effects of baicalein on MDA in the liver. C: Effects of baicalein on GPx in the liver. D: Effect of baicalein on T-AOC in the liver. NM, Normal mice administered 0.5% CMC-Na; MD, NAFLD mice administered 0.5% CMC-Na; LB, NAFLD mice administered 50 mg kg−1 d−1 baicalein in 0.5% CMC-Na; HB, NAFLD mice administered 200 mg kg−1 d−1 baicalein in 0.5% CMC-Na. The values are shown as the means ± standard errors, n = 8 for each group. Significant differences (*P < 0.05, **P < 0.01 and ***P < 0.001) were analyzed using one-way ANOVA followed by LSD test for multiple comparisons. | |
3.2.7. Effects of baicalein on AMPK, SREBP1, ACC and FASN.
To further assess the hepatic fat-lowering effect of baicalein in vivo, we examined the abundance of pSREBP1, the abundance of mSREBP1, the abundance of ACC, the abundance of FASN, the phosphorylation of AMPK, the phosphorylation of SREBP1, and the phosphorylation of ACC by immunoblotting. According to densitometry analysis, the abundance of pSREBP1 was increased by 1.19-fold and 1.31-fold, the abundance of mSREBP1 was decreased by 23.4% and 35.2%, the abundance of ACC was decreased by 27.0% and 35.4%, and the abundance of FASN was decreased by 25.0% and 35.2% in the LB group and HB group, respectively, compared with the MD group (Fig. 4A–F). In addition, in the LB and HB groups, the phosphorylation of AMPK groups was increased by 1.83-fold and 2.01-fold, the phosphorylation of SREBP1 was increased by 1.77-fold and 2.13-fold, and the phosphorylation of ACC was increased by 1.56-fold and 2.17-fold, respectively, compared with that in the MD group (Fig. 7F–I). These results suggested that baicalein increased the phosphorylation of AMPK, inhibited the transcriptional activity of SREBP1, and inhibited the synthesis of hepatic fat.
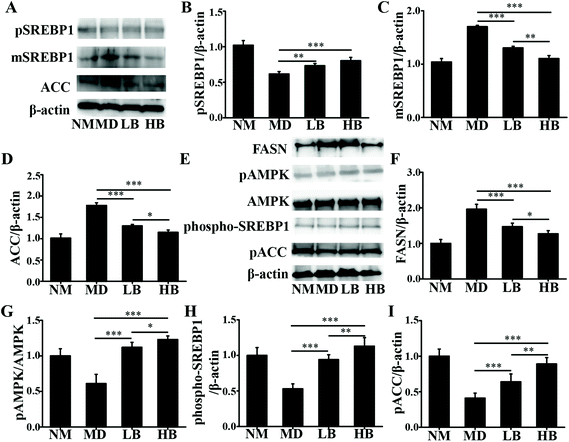 |
| Fig. 7 Effects of baicalein on pSREBP1, mSREBP1, FASN, ACC, pAMPK, phospho-SREBP1, and pACC in HFD-induced NAFLD mice. A: Immunoblot analysis of pSREBP1, mSREBP1, and ACC in the liver. B: Densitometry analysis of pSREBP1/β-actin in the liver. C: Densitometry analysis of mSREBP1/β-actin in the liver. D: Densitometry analysis of ACC/β-actin in the liver. E: Immunoblot analysis of FASN, pAMPK/AMPK, phospho-SREBP1, and pACC in the liver. F: Densitometry analysis of FASN in the liver. G: Densitometry analysis of pAMPK/AMPK in the liver. H: Densitometry analysis of phospho-SREBP1/β-actin in the liver. I: Densitometry analysis of pACC/β-actin in the liver. NM, Normal mice administered 0.5% CMC-Na; MD, NAFLD mice administered 0.5% CMC-Na; LB, NAFLD mice administered 50 mg kg−1 d−1 baicalein in 0.5% CMC-Na; HB, NAFLD mice administered 200 mg kg−1 d−1 baicalein in 0.5% CMC-Na. The values are shown as the means ± standard errors, n = 8 for each group. Significant differences (*P < 0.05, **P < 0.01 and ***P < 0.001) were analyzed using one-way ANOVA followed by LSD test for multiple comparisons. | |
4. Discussion
As a dietary food supplement in Eastern and Western cultures, baicalein has been widely used for many diseases, including hepatic disease. We and other researchers have found that baicalein can improve lipid metabolism in diabetic animals. However, IR plays an important role in the occurrence and development of NAFLD, and baicalein can ameliorate IR.20 Therefore, the hepatic fat-lowering effect and the anti-NAFLD effect of baicalein on a non-IR animal needed to be directly evaluated. Moreover, the mechanism through which baicalein reduced fat accumulation also needed to be elucidated in detail. The results of the present study demonstrated that baicalein increased the phosphorylation of AMPK, thus consequently inhibiting the transcriptional activity of SREBP1 and inhibiting the synthesis of hepatic fat to exert hepatic fat-lowering effects in oleic acid-induced fatty HepG2 cells and in HFD-induced non-IR NAFLD mice.
NAFLD is characterized by fat or TG accumulation, and directly limiting fat accumulation in the liver is a fundamental approach for NAFLD prevention and treatment. Fatty acids are the primary components of fat, and FASN and ACC are the rate-limiting enzymes of fatty acid synthesis.21 Reducing the abundance of FASN and ACC is a main approach to reducing fat accumulation. SREBP1 is a nuclear transcription factor that has been reported to widely regulate key enzymes that synthesize fatty acids, including FASN and ACC.22 Moreover, the phosphorylation of AMPK suppressed the cleavage and nuclear translocation of SREBP1 and then further repressed the expression of the SREBP1-mediated target genes in hepatic cells when the cells were treated with oleic acid, leading to a reduction in lipid accumulation.23 Additionally, the phosphorylation state of AMPK was found to be influenced by fluctuations in the levels of peripheral blood glucose and lipids.24 Therefore, we used mice with similar blood glucose and lipid profiles in immunoblot analysis to avoid false positive results caused by fluctuations in peripheral blood glucose and lipids. Our data suggested that baicalein increased the phosphorylation of AMPK and SREBP1, further suppressed the cleavage of SREBP1, and thus consequently inhibited the transcriptional activity of SREBP1 and the synthesis of hepatic fat both in vitro and in vivo. Notably, we used a reporter gene to assay the transcriptional activity of SREBP1, which a more direct measurement.25 These results were further confirmed by blocking the phosphorylation of AMPK with compound C. According to Fig. 3, compared with baicalein alone, the combination of baicalein and compound C increased the transcriptional activity of SREBP1, the abundance of ACC and FASN, and the levels of intracellular TG, suggesting that the AMPK phosphorylation inhibitor inhibited the effect of baicalein on SREBP1. Furthermore, many researchers have found that AMPK can inhibit the activity of ACC by increasing its phosphorylation level.26 In our study, baicalein also increased the phosphorylation of ACC, and this increase was blocked by the AMPK phosphorylation inhibitor. Therefore, baicalein reduced hepatic lipid accumulation by activating AMPK. Furthermore, since plasma-adipose-liver tissue exchange free fatty acids (FFA), baicalein could also reduce the plasma TG.27 Taking these results into consideration, increasing the phosphorylation of AMPK and inhibiting the transcriptional activity of SREBP1 might be one of the main mechanisms through which baicalein reduces hepatic fat accumulation and plasma lipid profiles, especially TG levels.
NAFLD is also characterized by the accumulation of TC or free cholesterol, and reducing TC accumulation is important for NAFLD prevention and treatment.28 Our results suggested that baicalein could reduce hepatic and plasma TC. The key enzymes in cholesterol biosynthesis are 3-hydroxy-3-methylglutaryl-CoA reductase (HMGCR), and HMGCR could be regulated by the AMPK-SREBP2 signaling pathway.29 Our results indicated that baicalein increased the phosphorylation of AMPK and that this might activate the phosphorylation of SREBP2, suppress the cleavage and nuclear translocation of SREBP2, and then further repress the expression of SREBP2-mediated target genes, for example HMGCR. Thus, the regulation of cholesterol biosynthesis by baicalein via AMPK/SREBP2 might be a mechanism to reduce hepatic TC, and because of the exchange of cholesterol between plasma-adipose-liver tissue, this might also be the mechanism to reduce plasma TC.30
The liver damage caused by lipid peroxidation and inflammation leads to hepatic dysfunction, hepatitis, fibrosis, cirrhosis and even carcinoma.31,32 In the present study and our previous studies, baicalein protected the liver from oxidative and inflammatory injury.33 These therapeutic effects were also beneficial for NAFLD prevention and treatment. For antioxidative activity, nuclear factor (erythroid-derived 2)-like 2 (Nrf2) is a master regulator of cellular detoxification responses and redox status that induces the expression of multiple antioxidant genes.34 Recent studies demonstrated that the AMPK/Akt2/Nrf2 pathway is an important regulator of antioxidative activity in the liver.35 Our data indicated that increased AMPK phosphorylation might activate the nuclear transcriptional activity of Nrf2 and enhance the antioxidant activity of the liver. Baicalein and associated glycosides were also reported to upregulate the gene expression of antioxidant enzymes.36 In addition, our previous antioxidant capacity study demonstrated that baicalein was a natural antioxidant and could eliminate free radicals directly in vivo.37 For the anti-inflammatory effect, many researchers have shown that baicalein exhibited anti-inflammatory activity through the suppression of NF-κB transactivation by inhibiting the Trx system.38 Moreover, our previous research indicated that baicalein could increase fecal SCFA content and improve gut barrier function by improving gut microbiota to reduce systemic inflammation.33 Based on the above results, baicalein has antioxidant activity and anti-inflammatory activity, and these activities are beneficial for NAFLD prevention and treatment.
Our data also indicated that baicalein could increase the level of HDLC and reduce the level of LDLC. Additionally, our previous studies and the previous work of many others also confirmed these therapeutic effects.16,17,33 These therapeutic effects are also beneficial for NAFLD prevention and treatment. However, the mechanism of these therapeutic effects is not clear and needs to be elucidated. We are trying to elucidate these mechanisms in vivo and in vitro.
5. Conclusion
In summary, baicalein reduced hepatic fat accumulation in vitro and in vivo by increasing the activation of AMPK and suppressing the cleavage of SREBP1, thus consequently inhibiting the transcriptional activity of SREBP1 and the synthesis of hepatic fat. Moreover, baicalein also showed TC-lowering, HDLC-raising, LDLC-lowering, antioxidant, and anti-inflammatory activities. Therefore, acting on multiple targets and pathways might be the mechanism through which baicalein impacts NAFLD prevention and treatment, and these results further support the use of baicalein as a dietary supplement in Eastern and Western cultures. In addition, this study will also be beneficial for understanding the anti-NAFLD mechanism of many other flavonoids.
Abbreviations
2h-PG | 2 h-postprandial glucose |
ACC | Acetyl coenzyme a carboxylase |
ALT | Alanine transaminase |
AMPK | 5′-Adenosine monophosphate (AMP)-activated protein kinase |
AST | Aspartate transaminase |
CMC-Na | Sodium carboxymethylcellulose |
DMEM | Dulbecco's modified Eagle's medium |
FASN | Fatty acid synthase |
FFA | Free fatty acid |
FINS | Fasting insulin |
FPG | Fasting plasma glucose |
GPx | Glutathione peroxidase |
HDLC | High-density lipoprotein cholesterol |
HFD | High-fat diet |
HMGCR | 3-Hydroxy-3-methylglutaryl-CoA reductase |
HOMA-IR | Homeostasis model assessment-IR |
IR | Insulin resistance |
LDLC | Low-density lipoprotein cholesterol |
LSD | Least significant difference |
mSREBP1 | Mature SREBP1 |
NAFLD | Nonalcoholic fatty liver diseases |
Nrf2 | Nuclear factor (erythroid-derived 2)-like 2 |
pSREBP1 | Precursor SREBP1 |
PVDF | Polyvinylidene fluoride |
SDS-PAGE | SDS–polyacrylamide gel electrophoresis |
SOD | Superoxide dismutase |
SREBP1 | Sterol regulatory element-binding protein 1 |
T-AOC | Total antioxidative capacity |
TBST | Tris-buffered saline with Tween-20 |
TC | Total cholesterol |
TG | Total triglyceride |
Conflicts of interest
The authors declare no conflict of interest.
Acknowledgements
The research was supported by the fund for the National Natural Science Foundation of China (Grant No. 81903878) and the Natural Science Foundation of Shandong Province, China (Grant No. ZR2019BH049).
References
- M. Ekstedt, P. Nasr and S. Kechagias, Natural History of NAFLD/NASH, Curr. Hepatol. Rep., 2017, 16(4), 391–397 CrossRef PubMed.
- Z. Younossi, Q. M. Anstee, M. Mariett, T. Hardy, L. Henry, M. Eslam, J. George and E. Bugianesi, Global burden of NAFLD and NASH: trends, predictions, risk factors and prevention, Nat. Rev. Gastroenterol. Hepatol., 2018, 15(1), 11–20 CrossRef PubMed.
- G. A. Michelotti, M. V. Machado and A. M. Diehl, NAFLD, NASH and liver cancer, Nat. Rev. Gastroenterol. Hepatol, 2013, 10(11), 656–665 CrossRef CAS PubMed.
- E. Buzzetti, M. Pinzani and E. A. Tsochatzis, The multiple-hit pathogenesis of non-alcoholic fatty liver disease (NAFLD), Metabolism, 2016, 65(8), 1038–1048 CrossRef CAS PubMed.
- Q. Liu, S. Bengmark and S. Qu, The role of hepatic fat accumulation in pathogenesis of non-alcoholic fatty liver disease (NAFLD), Lipids Health Dis., 2010, 9, 42 CrossRef PubMed.
- K. L. Donnelly, C. I. Smith, S. J. Schwarzenberg, J. Jessurun, M. D. Boldt and E. J. Parks, Sources of fatty acids stored in liver and secreted via lipoproteins in patients with nonalcoholic fatty liver disease, J. Clin. Invest., 2005, 115(5), 1343–1351 CrossRef CAS PubMed.
- A. L. Menezes, M. P. Pereira, S. L. Buzelle, M. P. Dos Santos, S. A. de França, A. M. Baviera, C. M. Andrade, M. A. Garófalo, I. C. Kettelhut, V. E. Chaves and N. H. Kawashita, A low-protein, high-carbohydrate diet increases de novo fatty acid synthesis from glycerol and glycerokinase content in the liver of growing rats, Nutr. Res., 2013, 33(6), 494–502 CrossRef CAS PubMed.
- D. M. Torres and S. A. Harrison, Diagnosis and therapy of nonalcoholic steatohepatitis, Gastroenterology, 2008, 134(6), 1682–1698 CrossRef CAS PubMed.
- E. Reynoso and J. E. Lavine, NAFLD: The role of exercise in treating NAFLD, Nat. Rev. Gastroenterol. Hepatol., 2012, 9(7), 368–370 CrossRef PubMed.
- C. Faggio, A. Sureda, S. Morabito, A. Sanches-Silva, A. Mocan, S. F. Nabavi and S. M. Nabavih, Flavonoids and platelet aggregation: A brief review, Eur. J. Pharmacol., 2017, 807, 91–101 CrossRef CAS PubMed.
- H. Trinh, Y. Yoo, K. H. Won, H. T. T. Ngo, J. E. Yang, J. G. Cho, S. W. Lee, K. Y. Kim and T. H. Yi, Evaluation of in vitro antimicrobial activity of Artemisia & apiacea H. and Scutellaria baicalensis G. extracts, J. Med. Microbiol., 2018, 67(4), 489–495 CrossRef CAS PubMed.
- C. S. Cheng, J. Chen, H. Y. Tan, N. Wang, Z. Chen and Y. Feng, Scutellaria baicalensis and Cancer Treatment: Recent Progress and Perspectives in Biomedical and Clinical Studies, Am. J. Chin. Med., 2018, 46(1), 25–54 CrossRef CAS PubMed.
- S. Salini, T. Chubicka, N. Sasidharan, E. R. Sindhu and T. D. Babu, Cytotoxic and antioxidant properties of selected Scutellaria species from the Western Ghats of Peninsular India, Pharm. Biol., 2013, 51(2), 152–159 CrossRef CAS PubMed.
- S. Havermann, R. Rohrig, Y. Chovolou, H. U. Humpf and W. Watjen, Molecular effects of baicalein in Hct116 cells and Caenorhabditis elegans : activation of the Nrf2 signaling pathway and prolongation of lifespan, J. Agric. Food Chem., 2013, 61(9), 2158–2164 CrossRef CAS PubMed.
- M. Li, A. Shi, H. Pang, W. Xue, Y. Li, G. Cao, B. Yan, F. Dong, K. Li, W. Xiao, G. He, G. Du and X. Hu, Safety, tolerability, and pharmacokinetics of a single ascending dose of baicalein chewable tablets in healthy subjects, J. Ethnopharmacol., 2014, 156, 210–215 CrossRef CAS PubMed.
- P. Pu, X. A. Wang, M. Salim, L. H. Zhu, L. Wang, K. J. Chen, J. F. Xiao, W. Deng, H. W. Shi, H. Jiang and H. L. Li, Baicalein, a natural product, selectively activating AMPKα2 and ameliorates metabolic disorder in diet-induced mice, Mol. Cell. Endocrinol., 2012, 362(1–2), 128–138 CrossRef CAS PubMed.
- W. Sun, J. Sun, B. Zhang, Y. Xing, X. Yu, X. Li, Z. Xiu and Y. Dong, Baicalein improves insulin resistance via regulating SOCS3 and enhances the effect of acarbose on diabetes prevention, J. Funct. Foods, 2017, 37, 339–353 CrossRef CAS.
- Y. Mi, D. Tan, Y. He, X. Zhou, Q. Zhou and S. Ji, Melatonin Modulates lipid Metabolism in HepG2 Cells Cultured in High Concentrations of Oleic Acid: AMPK Pathway Activation may Play an Important Role, Cell Biochem. Biophys., 2018, 76(4), 463–470 CrossRef CAS PubMed.
- Z. G. Zheng, Y. P. Zhou, X. Zhang, P. M. Thu, Z. S. Xie, C. Lu, T. Pang, B. Xue, D. Q. Xu, Y. Chen, X. W. Chen, H. J. Li and X. Xu, Anhydroicaritin improves diet-induced obesity and hyperlipidemia and alleviates insulin resistance by suppressing SREBPs activation, Biochem. Pharmacol., 2016, 122, 42–61 CrossRef CAS PubMed.
- F. Angelico, M. D. Ben, R. Conti, S. Francioso, K. Feole, S. Fiorello, M. Burattin, M. Mohanna, B. Zalunardo and F. Lirussi, Insulin resistance (IR) but not reduced insulin secretion (IS) is associated to non alcoholic fatty liver disease (NAFLD), J. Hepatol., 2003, 38(2), 190 CrossRef.
- J. D. Horton, J. L. Goldstein and M. S. Brown, SREBPs: activators of the complete program of cholesterol and fatty acid synthesis in the liver, J. Clin. Invest., 2002, 109(9), 1125–1131 CrossRef CAS PubMed.
- L. F. Wang, X. N. Wang, C. C. Huang, L. Hu, Y. F. Xiao, X. H. Guan, Y. S. Qian, K. Y. Deng and H. B. Xin, Inhibition of NAMPT aggravates high fat diet-induced hepatic steatosis in mice through regulating Sirt1/AMPKalpha/SREBP1 signaling pathway, Lipids Health Dis., 2017, 16(1), 82 CrossRef PubMed.
- Y. Li, S. Xu, M. M. Mihaylova, B. Zheng, X. Hou, B. Jiang, O. Park, Z. Luo, E. Lefai, J. Y. Shyy, B. Gao, M. Wierzbicki, T. J. Verbeuren, R. J. Shaw, R. A. Cohen and M. Zang, AMPK phosphorylates and inhibits SREBP activity to attenuate hepatic steatosis and atherosclerosis in diet-induced insulin-resistant mice, Cell Metab., 2011, 13(4), 376–388 CrossRef CAS PubMed.
- Z. Wan, C. Durrer, D. Mah, S. Simtchouk, E. Robinson and J. P. Little, Reduction of AMPK activity and altered MAPKs signalling in peripheral blood mononuclear cells in response to acute glucose ingestion following a short-term high fat diet in young healthy men, Metabolism, 2014, 63(9), 1209–1216 CrossRef CAS PubMed.
- G. Welch, R. Damoiseaux and L. Miraglia, Making It All Work: Functional Genomics and Reporter Gene Assays, Methods Mol. Biol., 2018, 1755, 89–105 CrossRef CAS PubMed.
- Z. Zheng, Y. Zhou, X. Zhang, P. M. Thu, Z. Xie, C. Lu, T. Pang, B. Xue, D. Xu, Y. Chen, X. Chen, H. Li and X. Xu, Anhydroicaritin improves diet-induced obesity and hyperlipidemia and alleviates insulin resistance by suppressing SREBPs activation, Biochem. Pharmacol., 2016, 122, 42–61 CrossRef CAS PubMed.
- N. D. Oakes, P. G. Thale'n, S. M. Jacinto and B. Ljung, Thiazolidinediones Increase Plasma-Adipose Tissue FFA Exchange Capacity and Enhance Insulin-Mediated
Control of Systemic FFA Availability, Diabetes, 2001, 50(5), 1158 CrossRef CAS PubMed.
- K. Ray, NAFLD-HCC: target cholesterol, Nat. Rev. Gastroenterol. Hepatol., 2018, 15(7), 390 CrossRef PubMed.
- H. Tang, R. Yu, S. Liu, B. Huwatibieke, Z. Li and W. Zhang, Irisin Inhibits Hepatic Cholesterol Synthesis via AMPK-SREBP2 Signaling, EBioMedicine, 2016, 6, 139–148 CrossRef PubMed.
- K. Ren, T. Jiang and G. J. Zhao, Quercetin induces the selective uptake of HDL-cholesterol via promoting SR-BI expression and the activation of the PPARγ/LXRα pathway, Food Funct., 2018, 9(1), 624–635 RSC.
- M. M. Rahman, A. Y. Muse, D. Khan, I. H. Ahmed, N. Subhan, H. M. Reza, M. A. Alam, L. Nahar and S. D. Sarker, Apocynin prevented inflammation and oxidative stress in carbon tetra chloride induced hepatic dysfunction in rats, Biomed. Pharmacother., 2017, 92, 421–428 CrossRef CAS PubMed.
- G. C. Farrell, F. Haczeyni and S. Chitturi, Pathogenesis of NASH: How Metabolic Complications of Overnutrition Favour Lipotoxicity and Pro-Inflammatory Fatty Liver Disease, Adv. Exp. Med. Biol., 2018, 1061, 19–44 CrossRef CAS PubMed.
- B. Zhang, W. Sun, N. Yu, J. Sun, X. Yu, X. Li, Y. Xing, D. Yan, Q. Ding, Z. Xiu, B. Ma, L. Yu and Y. Dong, Anti-diabetic effect of baicalein is associated with the modulation of gut microbiota in streptozotocin and high-fat-diet induced diabetic rats, J. Funct. Foods, 2018, 46, 256–267 CrossRef CAS.
- I. Bellezza, I. Giambanco, A. Minelli and R. Donato, Nrf2-Keap1 signaling in oxidative and reductive stress, Biochim. Biophys. Acta, Mol. Cell Res., 2018, 1865(5), 721–733 CrossRef CAS PubMed.
- L. Wang, S. Zhang, H. Cheng, H. Lv, G. Cheng and X. Ci, Nrf2-mediated liver protection by esculentoside A against acetaminophen toxicity through the AMPK/Akt/GSK3beta pathway, Free Radicals Biol. Med., 2016, 101, 401–412 CrossRef CAS PubMed.
- V. Y. Waisundara, S. Y. Siu, A. Hsu, D. Huang and B. K. Tan, Baicalin upregulates the genetic expression of antioxidant enzymes in Type-2 diabetic Goto-Kakizaki rats, Life Sci., 2011, 88(23–24), 1016–1025 CrossRef CAS PubMed.
- W. Sun, Y. Sang, B. Zhang, X. Yu, Q. Xu, Z. Xiu and Y. Dong, Synergistic effects of acarbose and an Oroxylum indicum seed extract in streptozotocin and high-fat-diet induced prediabetic mice, Biomed. Pharmacother., 2017, 87, 160–170 CrossRef CAS PubMed.
- R. S. Patwardhan, D. Sharma, M. Thoh, R. Checker and S. K. Sandur, Baicalein exhibits anti-inflammatory effects via inhibition of NF-kappaB transactivation, Biochem. Pharmacol., 2016, 108, 75–89 CrossRef CAS PubMed.
|
This journal is © The Royal Society of Chemistry 2020 |