DOI:
10.1039/C9FO02202C
(Paper)
Food Funct., 2020,
11, 596-605
Propacin, a coumarinolignoid isolated from durian, inhibits the lipopolysaccharide-induced inflammatory response in macrophages through the MAPK and NF-κB pathways
Received
21st September 2019
, Accepted 11th December 2019
First published on 12th December 2019
Abstract
Durian, known as the king of fruits, is rich in nutrients and bioactive phytochemicals. Propacin is a bioactive coumarinolignoid isolated from durian. In this study, we demonstrated its anti-inflammatory effect on lipopolysaccharide (LPS)-induced RAW264.7 cells and explored the underlying mechanism. Propacin suppressed nitric oxide (NO) and prostaglandin E2 (PGE2) production in LPS-stimulated macrophages significantly by downregulating the mRNA and protein expression of nitric oxide synthase (iNOS) and cyclooxygenase-2 (COX-2). Moreover, propacin decreased the overexpression of the LPS-induced reactive oxygen species (ROS) and maintained the mitochondrial integrity in active macrophages. Furthermore, propacin inhibited the translocation of the nuclear factor-κB (NF-κB) p65 subunit into the nucleus and the phosphorylation of mitogen-activated protein kinases (MAPKs), especially JNK and ERK. Collectively, these data indicated that propacin may have the potential to be developed as a novel therapeutic agent for inflammatory-related diseases.
Introduction
Inflammation is a vitally important process that serves to protect the body from harmful stimuli and pathogens.1 However, chronic inflammation is related to many serious diseases such as arthritis, atherosclerosis, obesity, migraines and so on due to the overproduction of inflammation mediators. Inflammatory diseases have become an important threat to human health worldwide.
Bacterial lipopolysaccharide (LPS) is a component of the outer membrane of Gram-negative bacteria, which can stimulate the release of inflammatory mediators such as nitric oxide (NO) and prostaglandin E2 (PGE2) from macrophages, as well as pro-inflammatory cytokines like tumor necrosis factor alpha (TNF-α), interleukin-1 beta (IL-1β), and interleukin-6 (IL-6).2 All these inflammatory cytokines are strategically positioned throughout the body tissues and orchestrate inflammatory processes.3 Pro-inflammatory cytokines such as TNF-α and IL-1β can lead to inflammation, fever, and tissue destruction when they are administered to humans.4 Mitogen-activated protein kinases (MAPKs) include four well-characterized subfamilies of kinases: extracellular signal-regulated kinases (ERK1/2), c-Jun NH2-terminal kinases (JNK-1/2/3), p38 (p38α/β/γ/δ) and ERK5.5 They are critical regulators of pro-inflammatory cytokine production. Therefore, feedback control of MAPK activation is necessary.6
Nuclear factor-κB (NF-κB) is clearly one of the most important regulators of pro-inflammatory gene expression, which has been considered to be a prototypical pro-inflammatory signaling pathway.7,8 Transcription of pro-inflammatory cytokines, mitochondrial membrane potential, nitric oxide synthase (iNOS) and cyclooxygenase-2 (COX-2) can be induced when NF-κB was highly activated. Synthesis of pro-inflammatory cytokines, such as TNF-α, IL-1β, and IL-6, is also mediated by NF-κB translocation.8
Anti-inflammatory drugs are widely used in the clinic, second only to anti-infective drugs; however the side effects of toxicity are undesirable. It is still urgent to develop low toxicity and high efficiency anti-inflammatory agents. Recently, natural products have become the focus of drug development due to their low side effects.9 Previous studies have shown that Durio species exhibited anti-inflammatory and antioxidant activities. Durian (Durio zibethinus Murr.) is cultivated in large quantities in Southeast Asia and China. Known as the king of fruits, durian is rich in nutrients such as carbohydrates, proteins, and vitamins B and C. Propacin (Fig. 1) is a phenolic extracted from the peels of durian.10 Our previous studies have shown the anti-inflammatory activity of propacin. However, the anti-inflammatory effect and mechanism of propacin have not been fully clarified yet. In this study, we attempted to explore the potential anti-inflammatory mechanism of propacin in the RAW264.7 cell model stimulated by LPS.
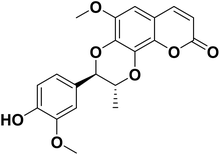 |
| Fig. 1 Chemical structure of propacin. | |
Materials and methods
Chemicals and reagents
Dulbecco's Modified Eagle's Medium (DMEM), fetal bovine serum (FBS), and penicillin–streptomycin solution were purchased from Thermo Scientific (Waltham, MA, USA). 3-(4,5-Dimethylthiazol-2-yl)-2,5-diphenyl tetrazolium bromide (MTT), Griess reagent, lipopolysaccharide and minocycline were procured from Sigma-Aldrich Chemicals (St Louis, MO, USA). The AxyPrep Multisource RNA Miniprep Kit was obtained from Corning (NY, USA). UltraSYBR Mixture was obtained from CWBIO (Beijing, China). RIPA cell lysis buffer, BCA protein assay kit and Nuclear and Cytoplasmic Protein Extraction Kit were purchased from Beyotime Biotechnology (Shanghai, China). Primary antibodies (p38, p-p38, JNK, p-JNK, ERK, p-ERK, p-IκBα, IκBα, and p-NF-κB) and the corresponding secondary antibodies for western blot analysis were purchased from Cell Signaling Technology (Danvers, MA, USA). The primary antibody NF-κB p65 was purchased from Santa Cruz Biotechnology (Dallas, TX, USA). The Super Sensitive ECL Luminescence Reagent was obtained from Dalian Meilun Biotechnology (Dalian, China). The propacin we used was isolated and purified in our lab, according to our previous work; the purity of propacin is 98.8%.10
Cell culture and cell viability
The murine macrophage cell line RAW264.7 was purchased from the Institute of Basic Medical Sciences of the Chinese Academy of Medical Sciences (China). The RAW264.7 cells were incubated in a humidified environment of 5% CO2 at 37 °C and maintained in DMEM containing 10% FBS and 1% penicillin–streptomycin solution (100 U mL−1 and 100 mg mL−1).
The viability of RAW264.7 cells in the presence of propacin was measured by MTT assay. The cells were treated in 96-well plates (2 × 104 cells per well) at 37 °C for 24 h. Various concentrations of propacin were added to the cell plates with or without LPS for another 24 h. After 4 h of incubation with MTT (0.5 mg mL−1), formazan crystals were formed and these were dissolved in 100 μL DMSO. The optical density was monitored using a micro-plate reader (Molecular Devices, LLC., San Jose, CA, USA) at 490 nm.
Measurement of NO production
The beginning of the procedure was the same as that described in the previous section. Equal volumes of cell culture medium and the Griess reagent (1% sulfanilamide and 0.1% N-(1-naphthyl) ethylenediamide dihydrochloride in 2.5% phosphoric acid) were mixed for 10 min at room temperature in the dark. The absorbance was measured using a micro-plate reader at 540 nm. A sodium nitrite standard calibration curve was used to determine the NO concentration.
Isolation of total RNA and quantitative reverse-transcription PCR (qRT-PCR) analysis
The AxyPrep Multisource RNA Miniprep Kit was used to isolate total RNA from the samples. Reverse transcription was performed with 1 μg RNA to produce cDNA using PrimeScript RT Master Mix (TaKaRa, Beijing, China). Quantitative real-time PCR was conducted over 40 cycles using UltraSYBR Mixture (CWBIO, Beijing, China). The primers for iNOS were 5′-AGCCAAGCCCTCACCTACTT-3′ (F) and 5′-GCCT CCAATCTCTGCCTATC-3′ (R); those for COX-2 were 5′-TGAGTACCGCAAACGCTTC TC-3′ (F) and 5′-TGGACGAGGTTTTTCCACCAG-3′ (R); those for IL-1β were 5′-GTTG ACGGACCCCAAAAGAT-3′ (F) and 5′-CCTCATCCTGGAAGGTCCAC-3 (R); those for IL-6 were 5′-CACGGCCTTCCCTACTTCAC-3′ (F) and 5′-TGCAAGTGCATCATC GTTGT-3′ (R); those for TNF-α were 5′-TATGGCTCAGGGTCCAACTC-3′ (F) and 5′-GGAAAGCCCATTTGAGTCCT-3′ (R); and those for β-actin were 5′-ATGTGGATCAGC AAGCAGGA-3′ (F) and 5′-AAGGGTGTAAAACGCAGCTCA-3′ (R). qRT-PCR was performed on a Light Cycler 96 system (Roche Diagnostics, Mannheim, Germany) and the results were analyzed using LightCycler software version 1.1.0.1320.
ELISA assay
RAW264.7 cells cultured in 24-well plates (6 × 105 cells per well) were treated with propacin and LPS (1 μg mL−1) for 24 h. The supernatant of each well was harvested; the PGE2 content was measured using the mouse PGE2 ELISA kit (Shanghai Enzyme-linked Biotechnology Co., Ltd, Shanghai, China), and the IL-6 and TNF-α contents were determined using the mouse IL-6 ELISA kit and mouse TNF-α ELISA kit (Elabscience, Wuhan, China) according to the manufacturer's instructions. The samples were tested three times in three independent experiments.
Western blot analysis
RAW264.7 cells were incubated overnight in 6-well plates at a density of 1 × 106 cells per well, and then treated with different concentrations of propacin and LPS (1 μg mL−1) for 2 h. Total cell protein was extracted using RIPA cell lysis buffer. For the preparation of nuclear and cytoplasmic protein samples, the cells were lysed using the Nuclear and Cytoplasmic Protein Extraction Kit. Protein concentrations were measured using the BCA protein assay kit. The protein samples were boiled with loading buffer for 5 min, resolved by 10% SDS-polyacrylamide gel electrophoresis, and transferred to polyvinylidene difluoride (PVDF) membranes (Millipore, Billerica, MA, USA). They were blocked with 5% BSA for 1 h at room temperature and then incubated with the first antibody overnight at 4 °C. After washing three times, the membranes were incubated with secondary antibodies at room temperature for 2 h. ECL was used for detecting the antibody interactions using FluorChem system E (Proteinsimple, Shanghai, China).
Determination of the mitochondrial membrane potential
A JC-1 fluorescent probe was widely used for the determination of the mitochondrial membrane potential. When the mitochondrial membrane potential is high, JC-1 accumulates and forms a polymer and emits red fluorescence. In contrast, JC-1 monomers can't aggregate to form a polymer in the mitochondrial matrix, and emit green fluorescence. Briefly, RAW264.7 cells were treated with LPS (1 μg mL−1) and propacin (20 μM) for 4 h and fixed with 4% formaldehyde for 15 min. Then the cells were incubated with JC-1 staining solution for 20 min at 37 °C. The images were captured using a fluorescence microscope (Zeiss GmbH, Jena, Germany).
Determination of the intracellular reactive oxygen species
DCFH-DA was used to determine the intracellular ROS. The cells were seeded in a 96-well plate at a density of 3 × 104 cells per well overnight. Then the cells were stimulated with LPS alone or together with different concentrations of propacin for 4 h. DCFH-DA at a final concentration of 20 μM was added to the medium at 37 °C for 30 min. The fluorescence was measured using a fluorescent microplate reader at 485 nm excitation and 530 nm emission wavelengths.
NF-κB nuclear translocation by immunofluorescence staining
RAW264.7 cells (4 × 104 cells per well) were incubated in 24-well plates overnight and treated with LPS and propacin for 6 h. Then the cells were fixed with 4% formaldehyde for 20 min at room temperature. Subsequently, the cells were permeabilized with 0.1% (v/v) Triton-X100 for 20 min and blocked with 0.5% (w/v) BSA dissolved in PBS for 1 h at room temperature, followed by incubation with anti-NF-κB overnight at 4 °C. After incubation with Chicken anti-Mouse IgG (H + L) Cross-Adsorbed Secondary Antibody and Alexa Fluor 488 conjugate (1
:
500 dilution) at room temperature for 2 h, the cells were washed with PBS three times. Following incubation, the cells were stained using 10 μg mL−1 Hoechst 33258 (Thermo Fisher Scientific, Waltham, MA, USA) for 15 min at room temperature. The cells were observed using a fluorescence microscope.
Statistical analysis
All data were expressed as the means ± SD from three independent experiments and were analyzed using GraphPad Prism 5.0 software (GraphPad Software, San Diego, CA, USA). Statistical analyses were performed by one-way ANOVA with Tukey's post-hoc test, and p < 0.05 was considered as a significant difference.
Results
Effect of propacin on RAW264.7 cell viability
To determine the cytotoxic effects of propacin on RAW264.7 cells, the MTT assay was performed at 24 h after treatment with propacin at a concentration of up to 40 μM in the presence of 1 μg mL−1 LPS. As shown in Fig. 2A, no significant differences in the cytotoxicity of propacin were observed on RAW264.7 cells. Based on these results, we chose to use concentrations of propacin at or below 40 μM for the following studies.
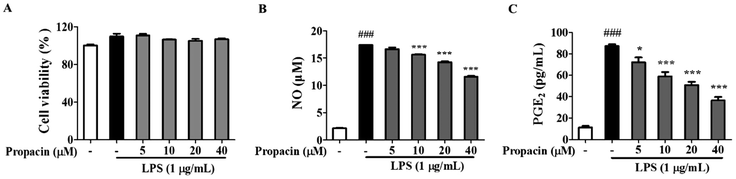 |
| Fig. 2 Effects of propacin on the viability and production of NO and PGE2 in RAW264.7 cells. RAW264.7 cells were treated with 0–40 μM propacin and 1 μg mL−1 LPS for 24 h. (A) The cell viability was measured by MTT assay. (B) NO production was determined using the Griess reagent. (C) The amount of PEG2 was determined using the ELISA kit. Values are presented as means ± SD (n = 3). | |
Effect of propacin on PGE2 and NO production in LPS-stimulated RAW264.7 cell lines
Once activated by LPS, NO and PGE2 are generated by macrophages at the inflammatory site. Therefore, as an initial indicator of the anti-inflammatory effects of propacin on LPS-stimulated RAW264.7 cells, the inflammatory mediator was assessed at non-cytotoxic concentrations. The level of NO and PGE2 in cell supernatants was determined using the Griess reagent and ELISA kit separately. Fig. 2B and C show that the NO and PGE2 levels were significantly increased when the cells were treated with LPS for 24 h; however, propacin treatment inhibited LPS-stimulated NO and PGE2 production in a concentration-dependent manner.
Effects of propacin on the expression level of iNOS and COX-2 activation in LPS-stimulated RAW264.7 cells
The observation that propacin inhibited NO and PEG2 production led us to further investigate the expression of iNOS and COX-2 on mRNA and protein levels. RAW264.7 cells were treated with different concentrations of propacin and 1 μg mL−1 LPS. The iNOS and COX-2 mRNA level and protein level were determined by qRT-PCR and western blot. As shown in Fig. 3A, the mRNA levels of iNOS and COX-2 were significantly increased in LPS treated cells compared to those in the control. However, propacin treatment significantly downregulated the expression of iNOS and COX-2 induced by LPS stimulation. Moreover, western blot analysis showed that the levels of iNOS and COX-2 protein significantly increased after LPS treatment (Fig. 3B). As expected, propacin treatment downregulated LPS-induced iNOS and COX-2 levels dose-dependently. These data demonstrate that propacin can reduce the production of NO and PGE2 by downregulating the expression of iNOS and COX-2, respectively, to achieve the effect of suppressing inflammation.
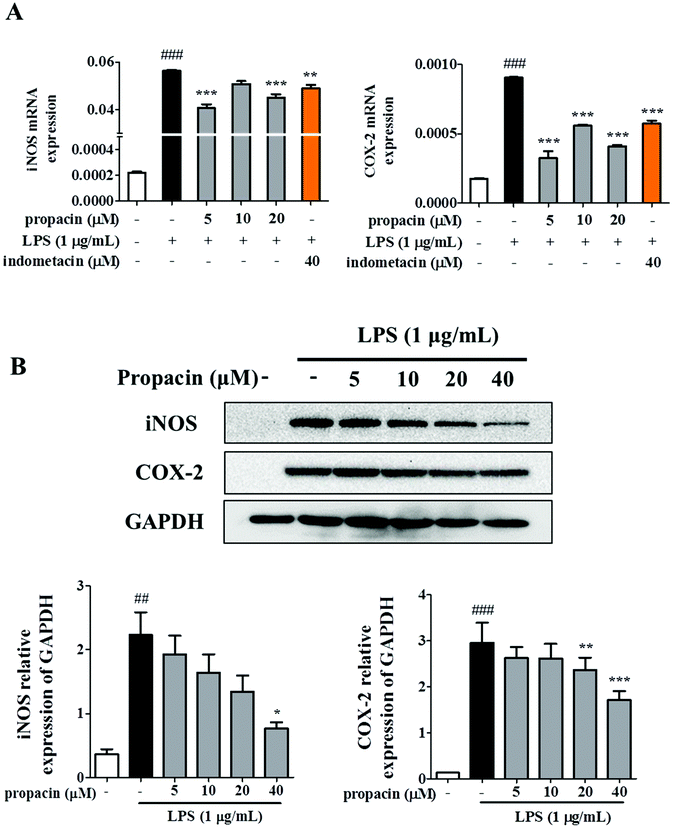 |
| Fig. 3 Effect of propacin on iNOS and COX2 against LPS in RAW264.7 cells. (A) Cells were treated with propacin in the absence and presence of LPS for 12 h. The iNOS and COX-2 mRNA levels were measured by RT-PCR; (B) cells were treated with different concentrations of propacin and 1 μg mL−1 LPS for 12 h. The expressions of iNOS and COX-2 protein were measured by western blot analysis. Values are presented as means ± SD (n = 3). ###p < 0.001 vs. control group; ##p < 0.01 vs. control group; *p < 0.05 vs. LPS group; **p < 0.01 vs. LPS group; and ***p < 0.001 vs. LPS group. | |
Effect of propacin on LPS-stimulated TNF-α, IL-6, and IL-1β production in RAW264.7 cells
The levels of pro-inflammatory cytokines such as IL-1β, TNF-α and IL-6 are elevated during the development of inflammatory diseases. Therefore, the mRNA expressions of TNF-α and IL-1β were estimated by qRT-PCR analysis after the cells were stimulated with LPS for 12 h. Fig. 4A, B and C showed that propacin dose-dependently reduced the mRNA levels of TNF-α, IL-1β, and IL-6. In addition, we also measured the secretion of TNF-α and IL-6 using ELISA assay. Fig. 4D and E showed that propacin dose-dependently reduced the secretion of TNF-α and IL-6. All these results suggest that propacin inhibits the LPS-stimulated expression of TNF-α, IL-1β, and IL-6 in macrophages.
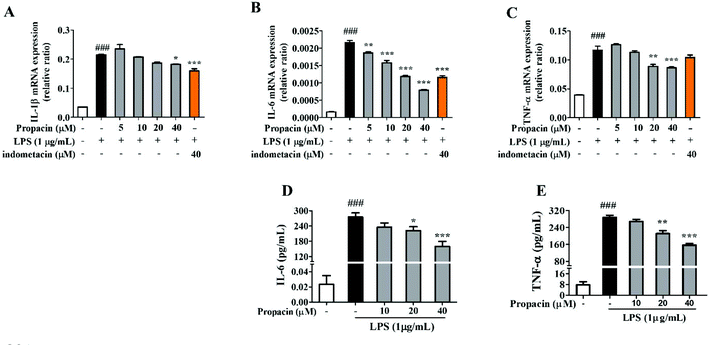 |
| Fig. 4 Effect of propacin on cytokine generation in RAW264.7 macrophages. Cells were treated with various concentrations of propacin along with LPS (1 μg mL−1) stimulation. (A–C) The expressions of IL-1β, IL-6 and TNF-α mRNA in cells were analyzed by qRT-PCR. (D, E) The secretions of IL-6 and TNF-α in cells were analyzed using the ELISA kit. Values are presented as means ± SD (n = 3). ###p < 0.001 vs. control group; ##p < 0.01 vs. control group; *p < 0.05 vs. LPS group; **p < 0.01 vs. LPS group; and ***p < 0.001 vs. LPS group. | |
Effect of propacin on LPS-induced NF-κB activation in RAW264.7 cells
The activation of NF-κB may regulate inflammatory mediators, such as iNOS, COX-2, IL-1β, IL-6, and TNF-α. Since NF-κB activation led to the nuclear translocation of RelA/p65, the effect of propacin on the p65 protein in the cytoplasm and nucleus was examined. The results of western blot showed that LPS led to p65 translocation from the cytoplasm to the nucleus, and propacin diminished the translocation (Fig. 5A).
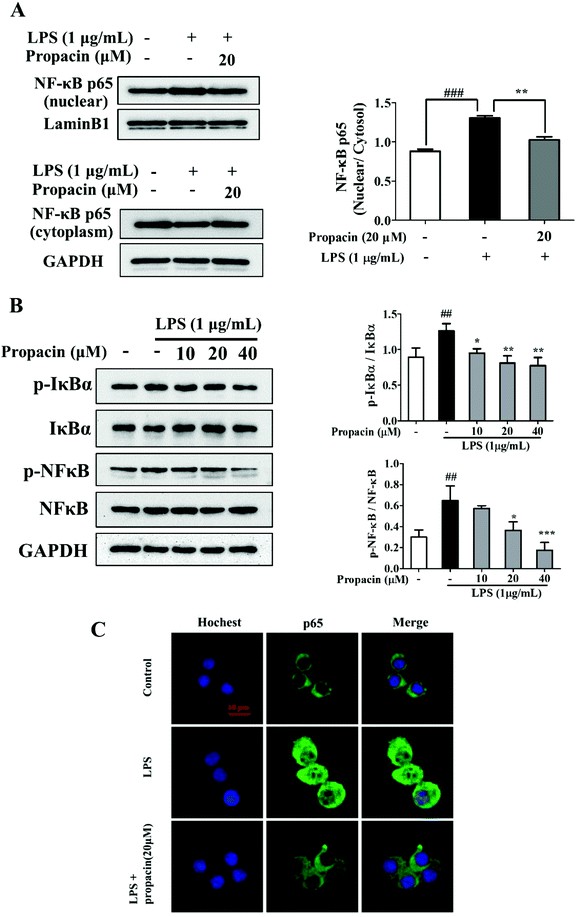 |
| Fig. 5 Effect of propacin on the NF-κB pathway in RAW264.7 macrophages. RAW264.7 cells were seeded into 6-well culture plates and incubated overnight, and treated with propacin along with LPS (1 μg mL−1) for 4 h. (A) Cytoplasmic and nuclear proteins were extracted to perform western blot analysis. (B) The effect of propacin on the phosphorylation of IκBα and NF-κB p65 was determined by western blot. (C) Immunofluorescence staining and fluorescence microscopy were used for observing NF-κB translocation. Scale bar = 10 μm. Values were presented as means ± SD (n = 3). ###p < 0.001 vs. control group; **p < 0.01 vs. LPS group. | |
To further explore the inhibitory effect of propacin on the activation of NF-κB induced by LPS, we also investigated the effect of propacin on the phosphorylation of NF-κB and IκBα using western blot. As shown in Fig. 5B, propacin remarkably suppressed LPS-stimulated phosphorylation of NF-κB and IκBα expression.
Immunostaining results showed further confirmation on the translocation of NF-κB (Fig. 5C). All these results demonstrated that propacin reduced LPS-induced p65 activation.
Effect of propacin on LPS-induced MAPK activation in RAW264.7 macrophages
MAPKs play an important role in the regulation of various physiological processes and regulate the expression of pro-inflammatory mediators such as iNOS and COX-2 as well as the production of pro-inflammatory cytokines. Here, we determined the effect of propacin on the MAPK signaling pathway by LPS stimulation by western blot analysis. After stimulating the RAW264.7 cells with LPS (1 μg mL−1) for 2 h, the phosphorylation levels of p38, JNK, and ERK were increased significantly, whereas treatment with propacin effectively reduced the phosphorylation levels of these kinases (Fig. 6A). The effect of propacin on the phosphorylation of p38 was the least, much more pronounced for JNK phosphorylation in a dose-dependent manner and the strongest for ERK1/2 phosphorylation in a dose-dependent manner. Propacin showed no effect on p38, JNK and ERK in LPS-induced macrophages.
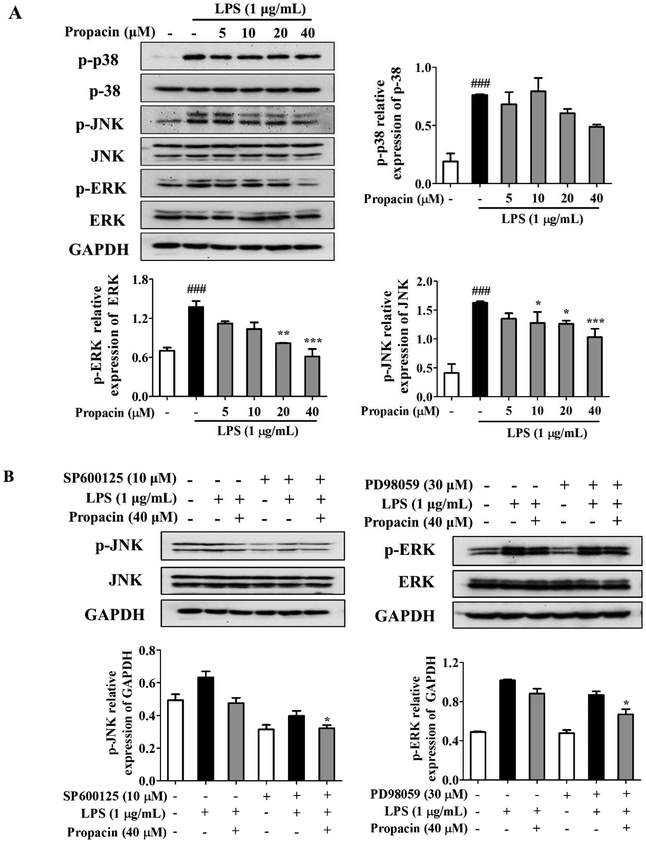 |
| Fig. 6 Effect of propacin and JNK and ERK inhibitors on the MAPK pathway in RAW264.7 macrophages. (A) RAW264.7 cells were treated with propacin in the presence of LPS (1 μg ml−1) for 2 h. Protein expression levels of the phosphorylated and total forms of ERK, JNK, and p38 were determined by western blot analysis. Values are presented as means ± SD (n = 3). ###p < 0.001 vs. control group; *p < 0.05 vs. LPS group; **p < 0.01 vs. LPS group; and ***p < 0.001 vs. LPS group. (B) Cells were pretreated with SP600125 (10 μM) or PD98059 (30 μM) alone for 2 h and then treated with propacin (40 μM) and LPS (1 μg mL−1) for 2 h. Protein expression levels of phosphorylated JNK and ERK were measured by western blot analysis. Values are presented as means ± SD (n = 3). *p < 0.05 vs. single agent treatment. | |
To further study the effect of propacin on p-JNK and p-ERK, each specific inhibitor (SP600125, JNK inhibitor; PD98059, ERK inhibitor) was employed. As shown in Fig. 6B, SP600125 and PD98059 treatment decreased the phosphorylation of JNK and ERK, respectively. The combined effect of propacin and the inhibitors augmented the suppression of the phosphorylation of JNK and ERK.
Effect of propacin on LPS-stimulated ROS production in RAW264.7 cells
ROS production is known to play an important role in inflammation. Overproduction of ROS is deleterious to cells at high concentrations.11 LPS is a well-documented inducer of ROS production. Therefore, we examined the effect of propacin on ROS production in LPS-induced RAW264.7 macrophages. As shown in Fig. 7A, the ROS level was observably increased by LPS in macrophages. However, 40 μM propacin significantly inhibited LPS induced ROS production in RAW264.7 cells.
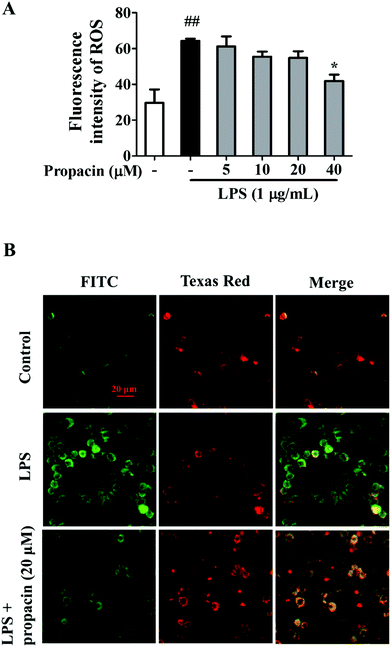 |
| Fig. 7 Effect of propacin on ROS production and mitochondrial membrane potential in LPS-stimulated RAW264.7 cells. RAW264.7 cells were treated with LPS (1 μg mL−1) at different concentrations of propacin for 4 h. (A) ROS was measured using a DCFH-DA probe and a fluorescent microplate reader. Values are presented as means ± SD (n = 3). (B) The mitochondrial membrane potential was determined using a JC-1 fluorescent probe and a fluorescence microscope. Scale bar = 20 μm. *p < 0.05 vs. LPS group. | |
Effect of propacin on the mitochondrial membrane potential in LPS-stimulated RAW264.7 cells
Increased ROS and MAPK activation may damage the integrity of the mitochondrial membrane systems.12 To study the impact of LPS and propacin on the mitochondrial membrane potential, fluorescent mitochondrial dye JC-1 and fluorescence microscopy were used. After incubation with LPS alone, the red component of JC-1 fluorescence disappeared and the green component of JC-1 fluorescence was enhanced compared to the normal group, indicating that the mitochondrial membrane was depolarized. However, this tendency was attenuated by propacin treatment (Fig. 7B).
Discussion
Inflammation has a protective effect against the aggression of injurious stimuli.13 However, it can become detrimental if dysregulated. Chronic inflammation may lead to many diseases, such as neurodegenerative diseases, arteriosclerosis, diabetes, obesity, and cancer.14 Macrophages play an important role in inflammation because of their phagocytic functions and production of cytokines and mediators, such as NO, PGE2, TNF-α and IL-1β.15 Recently, natural products have attracted a lot of attention because of their safety and availability. Polyphenols are ubiquitously produced by plants and exhibit numerous biological activities, like anti-inflammatory, antioxidant and cardiovascular protective activities.16 In our previous studies, propacin, the phenolic isolated from durian, was found to exhibit anti-inflammatory activity in LPS-induced RAW264.7 cells. Here, in this research, the underlying molecular mechanisms in the macrophages of propacin have been studied.
NO is a messenger gas molecule that is released at inflammatory sites, promoting the release of inflammatory cytokines. PGE2 was excessively produced during chronic inflammation in activated macrophages. Inhibition of NO and PGE2 production is thought to be an ideal strategy for the reduction of inflammation. iNOS and COX-2 are coding genes for enzymes that increase the synthesis of NO and PGE2.17,18 The stimulation of inflammatory cytokines and ROS lead to the overexpression of iNOS and COX-2 and cause chronic inflammatory diseases.19 Our results showed that stimulation with LPS significantly increased the expressions of NO and PGE2 in RAW264.7 cells, while propacin decreased the secretion of NO and PGE2 at the concentration of 40 μM and showed no cytotoxicity. Furthermore, propacin decreased the expressions of iNOS and COX-2 at both transcriptional and translational levels. Our results showed that propacin induced the decrease of iNOS and COX-2 protein expressions in a dose-dependent manner; however, the mRNA levels showed no dose correlation. For this phenomenon we assumed that it is because changes of mRNA were detected before changes of the protein, 5 μM propacin significantly decreased iNOS and COX-2 mRNA expression while it showed no effect on protein levels.
Cytokines released in the early stages of inflammation are called pro-inflammatory cytokines. TNF-α is one of the early responsive pro-inflammatory cytokines directly regulated by NO, which leads to the activation of the JNK signaling pathway.9 IL-1β is rapidly expressed, followed by the activation of iNOS, after LPS treatment. Macrophages express IL-6 when they are induced by microbes and play a beneficial role in the immune response, such as the regulation of TNF-α and IL-1β.20 However, persistent IL-6 induction could result in detrimental effects on the surrounding neurons or bone and lead to poor control of autoimmune response.21 As shown by our results, LPS significantly increased the mRNA expressions of IL-1β, IL-6, and TNF-α and the secretions of IL-6 and TNF-α compared to the non-treatment group. Propacin inhibited the expression of pro-inflammatory cytokines in a dose-dependent manner. These results suggested that propacin inhibited the expressions of TNF-α, IL-1β, and IL-6, thus slowing down the inflammatory response.
Various pro-inflammatory cytokines and inflammatory mediators are regulated by transcription factors. NF-κB plays an important role in the initiation of the transcription of pro-inflammatory cytokines, chemokines, COX-2, and iNOS.11 A heterodimer consisting of a p50 or p52 subunit and p65 is the most prevalent activated form of NF-κB. Inhibitor kappa B (IκB) suppresses the NF-κB translocation. Once IκB is phosphorylated by IκB kinase (IKK) activation, the cytoplasmic NF-κB–IκB complex releases NF-κB dimers. Dimers translocate to the nucleus and then phosphorylation of NF-κB enhances the transcription of pro-inflammatory cytokines.8 Thus, blocking of NF-κB might become a therapeutic strategy aimed against inflammatory disorders. To determine the effect of propacin on NF-κB translocation, the nuclear and cytosolic fractions and total protein were extracted. The results showed that LPS induction remarkably increased the phosphorylation of IκBα and NF-κB p65, and increased the expression of nuclear NF-κB and decreased the expression of cytoplasmic NF-κB. However, these events were blocked by treatment with propacin. Immunostaining also confirmed the translocation of NF-κB. LPS treatment led to strong fluorescence intensity in the nucleus, but this was inhibited by propacin. The results demonstrated that propacin suppressed the expression of pro-inflammatory cytokines through the NF-κB pathway.
As many research studies have shown in the past, LPS is a potent component of the activation of three MAPK pathways, including p38, JNK and ERK. Apart from irritating the release of inflammatory cytokines, such as IL-1β, IL-6 and TNF-α, previous studies have demonstrated that MAPKs are involved in the regulation of NF-κB activation.22,23 We demonstrated that propacin treatment diminished the activation of MAPK pathways stimulated by LPS, especially decreasing the phosphorylation of JNK and ERK. Meanwhile, a tendency for the suppression of p38 phosphorylation was observed. Furthermore, JNK or ERK inhibitors enhanced propacin induced downregulation of phosphorylation of JNK or ERK. Therefore, these results indicated that propacin restrained inflammatory response through both MAPK and NF-κB pathways.
Excessive ROS release may cause mitochondrial dysfunction and lead to various diseases, such as autoimmune diseases, cardiovascular diseases, metabolic syndromes and cancers.24 Recent studies indicate that mitochondrial ROS was known to be connected to the Toll-like receptor (TLR) pathway, associated with the activation of MAPK and NF-κB signaling pathways.25,26 Our study showed that LPS stimulation increased the ROS level and mitochondrial membrane depolarization in RAW264.7 cells, while treatment with propacin attenuated the generation of ROS and maintained the mitochondrial integrity.
Conclusion
In summary, propacin inhibited the LPS-induced immune response in macrophages through NF-κB and MAPK signaling pathways, leading to the suppression of iNOS, COX-2 and ROS production, regulating the mitochondrial integrity. Our results show that propacin isolated from durian exhibits excellent anti-inflammatory activity and is a potential anti-inflammatory drug.
Conflicts of interest
The authors declare that there was no competing interest.
Acknowledgements
This work was financially supported by the Pearl River S&T Nova Program of Guangzhou (201806010169), the National Natural Science Foundation of China (No. 81773592), and the Guangdong Province Universities and Colleges Pearl River Scholar Funded Scheme (2017).
References
- H. S. Park, D. E. Nelson, Z. E. Taylor, J. B. Hayes, K. D. Cunningham, B. A. Arivett, R. Ghosh, L. C. Wolf, K. M. Taylor, M. B. Farone, S. T. Handy and A. L. Farone, Suppression of LPS-induced NF-κB activity in macrophages by the synthetic aurone, (Z)-2-((5-(hydroxymethyl) furan-2-yl) methylene) benzofuran-3(2H)-one, Int. Immunopharmacol., 2017, 43, 116–128 CrossRef CAS PubMed.
- E.-A. Kim, S.-Y. Kim, J. Kim, J.-Y. Oh, H.-S. Kim, W.-J. Yoon, D.-H. Kang and S.-J. Heo, Tuberatolide B isolated from Sargassum macrocarpum inhibited LPS-stimulated inflammatory response via MAPKs and NF-κB signaling pathway in RAW264.7 cells and zebrafish model, J. Funct. Foods, 2019, 52, 109–115 CrossRef CAS.
- C. Varol, A. Mildner and S. Jung, Macrophages: development and tissue specialization, Annu. Rev. Immunol., 2015, 33, 643–675 CrossRef CAS PubMed.
- C. A. Dinarello, Proinflammatory cytokines, Chest, 2000, 118, 503–508 CrossRef CAS PubMed.
- G. Huang, L. Z. Shi and H. Chi, Regulation of JNK and p38 MAPK in the immune system: signal integration, propagation and termination, Cytokine, 2009, 48, 161–169 CrossRef CAS PubMed.
- Y. Zhang, D. Y. Leung, B. N. Richers, Y. Liu, L. K. Remigio, D. W. Riches and E. Goleva, Vitamin D inhibits monocyte/macrophage proinflammatory cytokine production by targeting MAPK phosphatase-1, J. Immunol., 2012, 188, 2127–2135 CrossRef CAS PubMed.
- T. Lawrence, The Nuclear Factor NF-κB Pathway in Inflammation, Cold Spring Harbor Perspect. Biol., 2009, 1, a001651 Search PubMed.
- P. P. Tak and G. S. Firestein, NF-κB: a key role in inflammatory diseases, J. Clin. Invest., 2001, 107, 7–11 CrossRef CAS PubMed.
- J. Ren, L. Li, Y. Wang, J. Zhai, G. Chen and K. Hu, Gambogic acid induces heme oxygenase-1 through Nrf2 signaling pathway and inhibits NF-kappaB and MAPK activation to reduce inflammation in LPS-activated RAW264.7 cells, Biomed. Pharmacother., 2018, 109, 555–562 CrossRef PubMed.
- J. Feng, Y. Wang, X. Yi, W. Yang and X. He, Phenolics from Durian Exert Pronounced NO Inhibitory and Antioxidant Activities, J. Agric. Food Chem., 2016, 64, 4273–4279 CrossRef CAS PubMed.
- C. Xie, X. Li, J. Zhu, J. Wu, S. Geng and C. Zhong, Magnesium isoglycyrrhizinate suppresses LPS-induced inflammation and oxidative stress through inhibiting NF-κB and MAPK pathways in RAW264.7 cells, Bioorg. Med. Chem., 2019, 27, 516–524 CrossRef CAS PubMed.
- E. Bognar, Z. Sarszegi, A. Szabo, B. Debreceni, N. Kalman, Z. Tucsek, B. Sumegi and F. Gallyas Jr., Antioxidant and Anti-Inflammatory Effects in RAW264.7 Macrophages of Malvidin, a Major Red Wine Polyphenol, PLoS One, 2013, 8, e65355 CrossRef CAS PubMed.
- J. N. Fullerton and D. W. Gilroy, Resolution of inflammation: a new therapeutic frontier, Nat. Rev. Drug Discovery, 2016, 15, 551–567 CrossRef CAS PubMed.
- R. Medzhitov, Origin and physiological roles of inflammation, Nature, 2008, 454, 428–435 CrossRef CAS PubMed.
- D. Korns, S. C. Frasch, R. Fernandez-Boyanapalli, P. M. Henson and D. L. Bratton, Modulation of macrophage efferocytosis in inflammation, Front. Immunol., 2011, 2, 57 Search PubMed.
- N. Yahfoufi, N. Alsadi, M. Jambi and C. Matar, The Immunomodulatory and Anti-Inflammatory Role of Polyphenols, Nutrients, 2018, 10, 1618 CrossRef PubMed.
- C. A. Dinarello, Proinflammatory Cytokines, Chest, 2000, 118, 503–508 CrossRef CAS PubMed.
- J. M. Han, E. K. Lee, S. Y. Gong, J. K. Sohng, Y. J. Kang and H. J. Jung, Sparassis crispa exerts anti-inflammatory activity via suppression of TLR-mediated NF-κB and MAPK signaling pathways in LPS-induced RAW264.7 macrophage cells, J. Ethnopharmacol., 2019, 231, 10–18 CrossRef CAS PubMed.
- S. W. Moon, C. B. Ahn, Y. Oh and J. Y. Je, Lotus (Nelumbo nucifera) seed protein isolate exerts anti-inflammatory and antioxidant effects in LPS-stimulated RAW264.7 macrophages via inhibiting NF-κB and MAPK pathways, and upregulating catalase activity, Int. J. Biol. Macromol., 2019, 134, 791–797 CrossRef CAS PubMed.
- A. L. Sampaio, J. Dalli, V. Brancaleone, F. D'Acquisto, M. Perretti and C. Wheatley, Biphasic modulation of NOS expression, protein and nitrite products by hydroxocobalamin underlies its protective effect in endotoxemic shock: downstream regulation of COX-2, IL-1beta, TNF-alpha, IL-6, and HMGB1 expression, Mediators Inflammation, 2013, 2013, 741804 Search PubMed.
- T. C. Moore, K. L. Bush, L. Cody, D. M. Brown and T. M. Petro, Control of early Theiler's murine encephalomyelitis virus replication in macrophages by interleukin-6 occurs in conjunction with STAT1 activation and nitric oxide production, J. Virol., 2012, 86, 10841–10851 CrossRef CAS PubMed.
- M. Huang, S. Zeng, Y. Zou, M. Shi, Q. Qiu, Y. Xiao, G. Chen, X. Yang, L. Liang and H. Xu, The suppression of bromodomain and extra-terminal domain inhibits vascular inflammation by blocking NF-kappaB and MAPK activation, Br. J. Pharmacol., 2017, 174, 101–115 CrossRef CAS PubMed.
- M. Guha and N. Mackman, LPS induction of gene expression in human monocytes, Cell. Signalling, 2001, 13, 85–94 CrossRef CAS PubMed.
- Y. Chen, Z. Zhou and W. Min, Mitochondria, Oxidative Stress and Innate Immunity, Front. Physiol., 2018, 9, 1487 CrossRef PubMed.
- B. Banoth and S. L. Cassel, Mitochondria in innate immune signaling, Transl. Res., 2018, 202, 52–68 CrossRef CAS PubMed.
- J. Park, J. S. Min, B. Kim, U. B. Chae, J. W. Yun, M. S. Choi, I. K. Kong, K. T. Chang and D. S. Lee, Mitochondrial ROS govern the LPS-induced pro-inflammatory response in microglia cells by regulating MAPK and NF-kappaB pathways, Neurosci. Lett., 2015, 584, 191–196 CrossRef CAS PubMed.
|
This journal is © The Royal Society of Chemistry 2020 |
Click here to see how this site uses Cookies. View our privacy policy here.