DOI:
10.1039/C9FO02029B
(Paper)
Food Funct., 2020,
11, 606-616
Stability and in vitro digestion study of curcumin-encapsulated in different milled cellulose particle stabilized Pickering emulsions
Received
1st September 2019
, Accepted 12th December 2019
First published on 13th December 2019
Abstract
The stability, in vitro digestion profile and phase behavior of Pickering emulsions stabilized by milled cellulose were evaluated to investigate their feasibility as food-grade formulations for encapsulation and delivery of lipophilic bioactive compounds. Curcumin encapsulated in Pickering emulsions exhibited good stability with less than 50% degraded after 30 days’ storage. The digestion profiles of emulsions were markedly influenced by lipid type used and digestion buffer employed in simulated small intestinal experiments. The rate and extent of lipolysis of emulsions with medium chain triglycerides were greater than emulsions with long chain triglycerides (soy bean oil and canola oil), reaching complete hydrolysis under both fed and fasted conditions. For comparison, the digestion behaviors of curcumin encapsulated in conventional emulsions were also evaluated. Although the initial digestion rate of Pickering emulsions with long chain triglycerides was slower than the corresponding conventional emulsions stabilized by Tween/Span 80, their total extent of lipolysis was higher than that of conventional emulsions. The bioaccessibility of curcumin encapsulated in Pickering emulsions was higher than in corresponding small molecular weight surfactant stabilized conventional emulsions.
1. Introduction
Although the biological benefits of a variety of lipophilic compounds have been identified, their dose efficacies of oral administration are often limited since they have low stability against degradation and low bioavailability.1–5 By affecting gastric transit, intestinal permeability or metabolism, lipid-based oral delivery systems are perhaps the most widely used strategy to improve the bioavailability of poorly water-soluble, lipophilic compounds through enhancing their solubilization and dissolution in the gastrointestinal tract.6,7 Encapsulation of these lipophilic functional compounds using emulsion system is a commonly used formulation approach in food, cosmetic and pharmaceutical industries.3,4 Extensive studies have been conducted to evaluate the encapsulation, oxidative stability and release of bioactive compounds in conventional emulsions stabilized by small molecular weight surfactants or biopolymers. Nowadays solid particle stabilized emulsions, which is the so-called Pickering emulsions, have exhibited good stability against coalescence and Ostwald ripening.8,9 Although the unique physicochemical and mechanical properties of Pickering emulsions have been extensively investigated, characterization of stability and release of functional lipophilic compounds encapsulated in these emulsions is still limited. Moreover, the comparative study on digestion behavior of Pickering emulsions and small molecular surfactant stabilized conventional emulsions has been scarcely investigated. Some studies have studied the role of silica stabilized Pickering emulsions in digestion behavior under simulated digestion conditions, delivery and release of bioactive compounds.10–12 However, oral consumption of formulations containing silica nanoparticles has gained attention due to its potential deleterious effects to human health. Silica with particles sizes of 5–50 and 50–500 nm have been reported to be likely to result in enhanced exposure of intestinal epithelia to these particles, which could have ramifications to health.13
Recently, various particles derived from cellulose have been fabricated to stabilize Pickering emulsions with superior physical and chemical stability.14,15 These particles include microfibrillated cellulose, moderately degraded microfibrils,16 cellulose nanocrystals, well-define nanocrystalline particles from acid hydrolysis process of cellulose17 and hydrophobically modified cellulosic material, such as acetylated nanofibers,18 carboxymethylated cellulose,19 or silylated microfibrils.20 Media milling process is a simple physical method, which has been used to produce various nano/submicrometer particles.21–23 And the particle size of cellulose has been proved to be effectively reduced by a media mill.24,25 Coming from natural biomass-based materials under physical modification treatment, milled cellulose particles could avoid the presence of hazardous contaminations when chemical methods were applied. Hence, milled cellulose stabilized Pickering emulsions have the high potential as edible delivery systems to encapsulate, protect and release bioactive lipophilic compounds with application in food and pharmaceutical industries. To the best of authors’ knowledge, there is no reported study that reveals the encapsulation ability and in vitro digestion aspects of milled cellulose stabilized Pickering emulsions.
As a bioactive lipophilic regent, curcumin has exhibited significant anti-cancer and anti-inflammatory properties.26 However, with low water solubility and stability, the systemic bioavailability and pharmacokinetics of curcumin were extremely low.27–30 Hence, emulsion-based delivery systems have been developed to improve the effectiveness of oral delivery of curcumin.31–33 In this work, milled cellulose particles were prepared via a media milling process, which then were used to stabilize a Pickering emulsion delivery system.25 To evaluate the encapsulation, protection and release capacity of milled cellulose stabilized Pickering emulsion delivery system, curcumin was encapsulated in the emulsion as a model lipophilic compound. The structure of emulsions, the stability of encapsulated curcumin as well as the emulsions responsiveness to the simulated small intestinal digestion environments were characterized. For enhanced understanding of the stability of the emulsions under simulated digestion conditions, the structures of emulsions were analyzed using microscopic and fluorescence imaging, and the distribution of curcumin between pellet and supernatants was analyzed. Moreover, the digestion behavior of curcumin encapsulated in conventional emulsions was characterized as a control delivery system to milled cellulose stabilized Pickering emulsions.
2. Materials and methods
2.1 Materials
Sigmacell cellulose type 20 was purchased from Sigma-Aldrich (St Louis, MO). The soy bean and canola oil was purchased from a local supermarket. MCT (Medium chain triglyceride, Neobee@1053) was from Stepan Company (Northfield, IL). Curcumin was a gift from Sabinsa Corporation (Piscataway, NJ), which contains 85% curcumin, 11% demethoxycurcumin and 4% bisdemethoxycurcumin.32 Sodium taurodeoxycholate (NaTDC) was purchased from CalBiochem (La Jolla, CA). Phosphatidylcholine (PC75 rapeseed lecithin) was a gift from the American Lecithin Co. (Oxford, CT). Lipase type II from porcine pancreas, Tween 80, Span 80, potassium dihydrogen phosphate, calcium chloride, sodium chloride, and bile salt were purchased from Sigma-Aldrich (St Louis, MO).
2.2 Media-milling treatment
The milling process was conducted using the same procedure as in previous study.25 Briefly, cellulose suspended in water was filled into a MiniSeries media mill (Netzsch LLC, PA, USA), where cellulose was milled under milling speed of 3000 rpm and temperature below 30 °C. Milled cellulose suspension with milling time of 15 h was used to prepare Pickering emulsion. And the accurate weight of milled cellulose in the final suspension was determined using a moisture test by measuring the weight of milled cellulose suspension before and after drying at 105 °C for 8 h.
2.3 Preparation of curcumin-loaded Pickering emulsions
Curcumin was firstly added into MCT, soy bean oil, canola oil at 8 mg mL−1 and heated at 120 °C under magnetic stirring for around 30 min to reach a transparent solution. Then the oil phase containing dissolved curcumin was mixed with fresh prepared milled cellulose suspension at a water/oil ratio of 1
:
1 (v/v) and the curcumin-loaded Pickering emulsion was formed using an IKA Ultra-Turrax T25 homogenizer operating at 10
000 rpm for 2 min. The cellulose concentration was expressed as weight content relative to the water phase based on the prior moisture test. Control emulsion samples were freshly prepared by mixing aqueous phase containing 1% Tween 80 and 1% Span 80 with oil phase containing dissolved curcumin at oil/water ratio of 1
:
4 (v/v) using an IKA Ultra-Turrax T25 homogenizer operating at 14
000 rpm for 3 min.
2.4 Microscopy characterization of curcumin-loaded Pickering emulsions
Sample microstructures were assessed using a Nikon Eclipse TE2000-U (Japan) microscope equipped with a 1392 × 1040 resolution CCD camera (Retiga EXi, QImaging). The emulsions were placed on a glass microscopic plate for microscopic observation. Fluorescence images were captured using blue filter under fluorescence mode. The volume mean droplet diameter of emulsions was analyzed using an ImageJ program by statistically calculating over two hundred droplets from at least three different microscopic images.
2.5 Curcumin concentration and its storage stability in emulsions
The concentration of curcumin encapsulated in the Pickering emulsion was determined using the following method. 200 μL of emulsion was thoroughly mixed with 9 mL of methanol. The mixture was then centrifuged at 14
000 rpm for 10 min. The concentration of curcumin in the supernatant was further diluted to an appropriate concentration using methanol and analyzed by HPLC. The storage stability of curcumin in the emulsions was determined as a function of storage time. Emulsion samples were stored at room temperature in dark for 30 days. And curcumin concentration in the samples at different storage periods were measured using the same method described above.
2.6
In vitro lipolysis digestion of emulsions
The in vitro lipolysis digestion of emulsions was evaluated using a pH-stat lipolysis model, which is a widely used method to simulate the digestion of emulsions and predict the in vivo solubilization of lipophilic compounds in small intestine.34,35 With the digestion of lipids, the lipolysis products and the bile salts, endogenous phospholipids in the digestion media would form micelles and mixed micelles, which would solubilize the lipophilic compounds and make them possible to be absorbed.35 Since the concentration of bile salts and phospholipids in the small intestine under fed state would be higher than under fasted state due to the response to the consumption of food, two lipolysis buffers were prepared to simulate the different digestion environments in the fasted and fed states (Table 1).34,36
Table 1 Formulation of lipolysis buffers in the fasted and fed states
|
Fasted state |
Fed state |
Tris maleate (mM) |
50 |
50 |
NaCl (mM) |
150 |
150 |
CaCl2·2H2O (mM) |
5 |
5 |
NaTDC (mM) |
5 |
20 |
Phosphatidylcholine (mM) |
1.25 |
5 |
The pH-stat lipolysis model was conducted according to the following procedure. Pancreatin extract solution was prepared by adding 1 g of pancreatin into 5 mL of lipolysis buffer and being stirred for 15 min. The mixture was centrifuged at 2000 rpm for 15 min and the supernatant was collected, stored on ice. The pancreatin extract used was freshly prepared every day. Emulsion samples containing 250 mg of oil phase (around 500 μL of Pickering emulsion or 1250 μL of conventional emulsion) was mixed with 9 mL of lipolyis buffer and kept at 37 °C for 10 min under continuously stirring. Then the pH of resultant mixture was adjusted to 7.5 using 0.25 M NaOH solution. Finally, 1 mL of ice-chilled pancreatin solution, which contained extract of pancreatin coming from 200 mg of pancreatin powder, was added in to initiate the lipolysis. During the digestion process of 2 h, the pH of digestion media was maintained at 7.5 ± 0.1 by adding 0.25 M NaOH and the volume of added NaOH solution was recorded as function of time. During the digestion process, 50 μL of digestion media were pipetted out and observed under optical and fluorescent microscope at digestion time of 0, 30, 120 min. And the digestion of emulsions stabilized by 1% Tween 80 and Span 80 was also conducted as control samples.
The amount of NaOH added through the lipolysis experiment was assumed to equal to the amount of free fatty acids (FFAs) generated by lipolysis of triacylglycerols. The %FFA released was calculated based on a previously method assuming that all the triacylglycerols were converted to two free fatty acids during titration.37
|  | (1) |
where
CNaOH is the concentration of NaOH solution used during titration,
Vblank and
Vlipid is the volume of NaOH titrated during digestion of blank and emulsion sample,
Mw,lipid is the average molecular weight of the lipid (g per mol), and
mlipid is the total mass of lipid in the emulsion sample (g).
Upon completion of the lipolysis digestion, the lipolysis media were subjected to ultracentrifugation for 40 min at 4 °C and 40
000 rpm, which would be separated into a precipitated pellet on the bottom, an aqueous phase in the middle and sometimes an oily phase on the top. The amount of curcumin precipitated in the pellet was analyzed by suspending the pellet in 10 mL methanol, centrifuging at 4500 rpm for 15 min. The supernatants were diluted into suitable concentrations and injected into HPLC. The middle aqueous phase containing formulated curcumin micelles was collected and the volume was recorded. The concentration of curcumin in the micelle phase was analyzed using HPLC. The bioaccessibility (%) of curcumin was calculated based on the equation below:
|  | (2) |
2.7 High-performance liquid chromatography (HPLC) analysis
The UltiMate 3000 HPLC system equipped with an auto sampler and a 25D UV-VIS absorption detector (Dionex) was connected to a Nova-Pak C18 column, 3.9 × 150 mm (Waters). The detection wavelength used was 420 nm. Injection volume was set at 20 μL and the flow rate was 1 mL min−1. The detection of curcumin was using mobile phases of water with 2% acetic acid and acetonitrile. The total elution time was 23 min, where the mobile phase started from 35% acetonitrile in 2 min, then linearly increased to 55% in 15 min, and was kept at 55% for the next 5 min, and was then linearly reduced back to 35% for the final minute. The identified peak areas from HPLC-analysis were converted into concentrations using standard curves.
2.8 Statistical analysis
Experiments were conducted at least in duplicate, and results were expressed as the mean ± standard deviation (SD). Significant differences of mean values (p < 0.05) were measured by Duncan's test using the SPSS 17.0 package.
3. Results and discussion
3.1 Characterization of curcumin-loaded Pickering emulsion
Pickering emulsions with different lipid phases were stabilized by milled cellulose from a media milling process. Based on the moisture test conducted after the milling process, the cellulose concentration in the aqueous dispersion was 3.7% (w/w). The mean particle size of milled cellulose was 463 nm.25 The oil/water ratio of the emulsions used was 1
:
1. The microscopy and fluorescence images of Pickering emulsions containing MCT, soy bean and canola oil are presented in Fig. 1. The droplets of Pickering emulsion with MCT, canola and soy bean oil had a volume mean diameter of 46 ± 12, 27 ± 8 and 41 ± 11 μm, respectively (Table 2). Emulsions of MCT had a similar droplet size as emulsions with soy bean oil, which was higher than that of emulsions formed by canola oil. These results indicated that the formation of emulsions stabilized by milled cellulose could be impacted by the type of oil phase used. The influence of oil phase on the droplet size of resulting emulsions might be attributed to the differences in the fatty acid composition, density and polarity of different oils leading to differences in surface tension between oil phase and water. The brighter regions in the fluorescence images of emulsions represented the oil droplets with dissolved curcumin owing to the emission of fluorescence from curcumin under excitation. It was notable that no apparent needle-like crystals were detected in all the emulsions, which suggested that curcumin did not precipitate after encapsulated in these emulsions. The emulsions stabilized by milled cellulose possessed good stability without precipitation of curcumin during long-term storage.
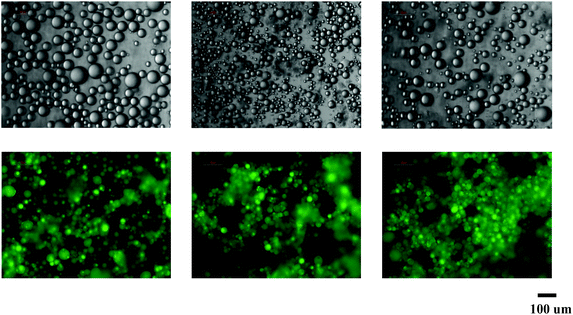 |
| Fig. 1 Microscopic images of curcumin encapsulated emulsions stabilized by milled cellulose particles. From left to right: MCT, canola oil and soy bean oil. | |
Table 2 Droplet size of Pickering and conventional emulsions with different oil phases
Lipid |
Diameter (μm) |
Pickering emulsion |
Convention emulsion |
MCT |
46 ± 12 |
19 ± 7 |
Canola oil |
27 ± 8 |
16 ± 6 |
Soy bean oil |
41 ± 11 |
23 ± 9 |
3.2 Stability of curcumin in milled cellulose stabilized Pickering emulsion
Curcumin has a high sensitivity to environmental conditions, which is unstable in various solvent conditions. In PBS of pH 7.2, more than 85% of free curcumin was found to degrade within 30 min of incubation.38 And around 80% of curcumin degraded in 90 min when being dissolved in water with 3% v/v of methanol (pH 5.7).10 The retention of curcumin in the milled cellulose stabilized Pickering emulsions as a function of time is depicted in Fig. 2. Curcumin in Pickering emulsions with different oil phases exhibited similar degradation patterns. And the degradation rate was not significantly different for emulsions containing different oil phases. Curcumin encapsulated in these Pickering emulsions exhibited improved stability and high retentions. Through encapsulation in emulsions with MCT, canola and soy bean oil, 81.5 ± 3.5%, 80.1 ± 2.6%, and 82.5 ± 2.5% of original curcumin were retained in the emulsions after 30 days’ storage. It is notable that the stability of curcumin is much higher in these Pickering emulsions than in other encapsulation systems. Curcumin in Pickering emulsion stabilized by starch granule with heat-treatment also exhibited good encapsulation stability of around 78% during storage of 24 h.39 However, studies showed that approximately 40% of curcumin was degraded within 20 h and in less than 200 min when be encapsulated in sodium dodecyl sulfate micelles and liposomes, respectively.40,41 The predominant enhancement in the curcumin stability within this Pickering emulsion system indicated its high potential as a lipophilic functional ingredient encapsulation system for application in food, cosmetic, and pharmaceutical industries.
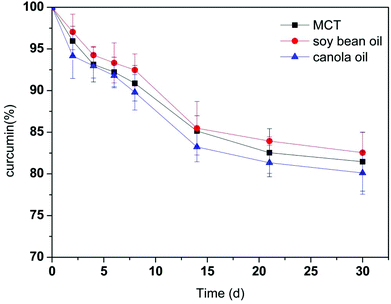 |
| Fig. 2 Stability of curcumin in Pickering emulsion with different oil phases. | |
3.3 Influence of oil type on in vitro lipid digestion
Curcumin encapsulated in milled cellulose stabilized Pickering emulsions were produced with different types of lipids. The influence of carrier oil type on rate and extent of lipid digestion was quantified using a pH-stat lipolysis model by monitoring the amount of free fatty acids (FFAs) released over time (Fig. 3). The changes in the structure of emulsions during digestion process are presented in Fig. 4. The nature of carrier oil exhibited a major influence on the kinetics of emulsion digestion. MCT is mainly made of saturated medium chain fatty acids, caprylic (C8:0) and capric (C10:0) triglycerides. Soy bean and canola oil majorly consist of unsaturated long chain fatty acids, including oleic acid (C18:1) and linoleic acid (C18:2). Compared to emulsions with soy bean oil and canola oil, the released of FFAs for emulsions with MCT was much faster and higher, with more than 85% of MCT being converted to monoglyceride and fatty acids after 40 min of digestion under fed-state digestion. This is consistent with results in previous study, which found that digestion rate of medium chain triacylglycerols was much higher than that of long chain triacylglycerols.42 While the digestion of emulsions with soy bean oil and canola oil was only 63% and 46% at the end of 2 h digestion process. The susceptibility of MCT to digestion could be attributed to several factors including solubility, hydrophilicity and phase behavior. At physiological pH, medium chain fatty acids (apparent pKa 6.843) could be ionized to a larger extent than long chain fatty acids (apparent pKa 8–8.543), thus enhancing solubility and amphiphilicity. As a result, the aqueous solubility of medium chain lipolytic digestion products is much higher than that of long chain lipolytic digestion products, which could dissociate from the digesting interface rapidly and incorporate into digestion buffer easily to either be incorporated into micellar structure or may precipitate as calcium or sodium soaps. This is in contrast to digestion of lipid with long chain fatty acids, where concentration of bile salt and solubilization capacity of digestion media are crucial to remove long chain FFAs. The lower extent of lipolysis for emulsions with soy bean and canola oil might be due to shortage of calcium or bile salts available to remove the long chain FFAs produced during digestion, which would accumulate at lipid droplet surface to inhibit the function of lipase.34 During the lipolysis process of emulsions with MCT, a significant amount of needle-like crystals was observed to precipitate out (Fig. 4), which might be the precipitation of calcium or sodium soaps. But these crystals did not exist in digestion media of emulsions with soy bean and canola oil. The microscopic images also revealed the disappearance of oil droplets in the digestion buffer of emulsions with MCT, indicating the complete digestion of MCT during digestion. While a large amount of oil droplets still present in digestion buffer for emulsions with soy bean and canola oil after 120 min of digestion.
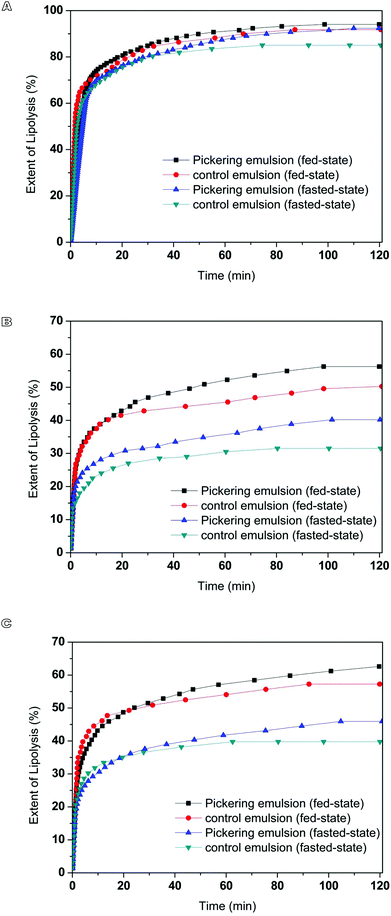 |
| Fig. 3 Release kinetics of fatty acids from Pickering emulsion and control emulsion containing different oil phases during in vitro fed- and fasted-state lipolysis. (A) MCT, (B) canola oil, and (C) soy bean oil. | |
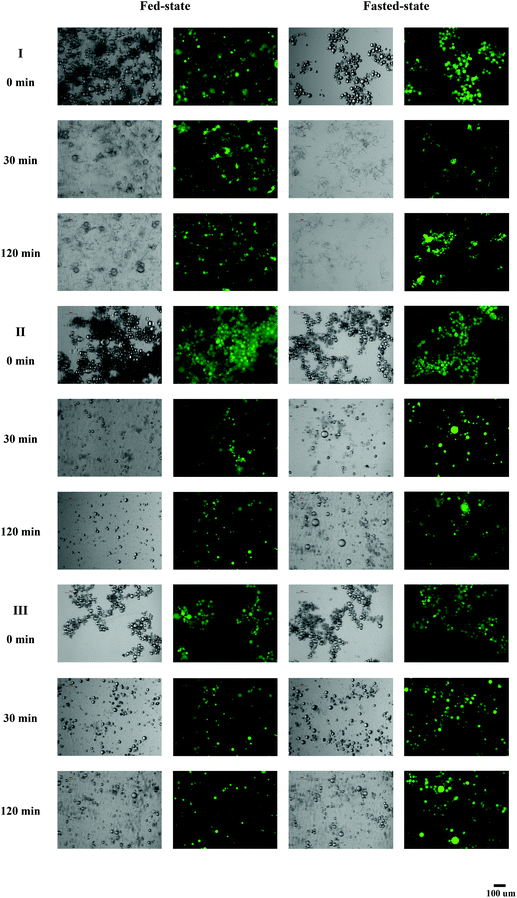 |
| Fig. 4 Microscopic and fluorescence images of Pickering emulsion containing different oil phases incubated in in vitro lipolysis at different time. (I) MCT, (II) soy bean oil, and (III) canola oil. | |
3.4 Influence of digestion buffer on in vitro lipid digestion
The influence of digestion buffer on digestion of Pickering emulsions with different oil phases (MCT, soy bean and canola oil) was characterized by monitoring the release of FFAs over time under simulated fasted and fed conditions (Fig. 3). At the beginning of digestion of Pickering emulsions containing canola and soy bean oil, a continuous increase in the release of fatty acids was observed as lipolysis proceeded. After 10 min of digestion, the rate of lipolysis decreased, which might result from the saturation of phospholipid/bile salt micelles with lipolytic products. At the end of 2 h digestion process, the extent of FFAs released in Pickering emulsion with canola and soy bean oil under fed-state conditions was 56 ± 5% and 63 ± 3%, which was approximately 1.5-fold higher than that observed under fasted-state conditions (canola oil: 40 ± 3%, soy bean oil: 46 ± 2%). In both cases, long chain lipid was partially digested over 2 h of digestion under fasted- and fed-state conditions. In consistent with results obtained in this work, the increased rate and extent digestion of long chain lipid under higher bile salt concentration were also observed in previous studies.34,44 Bile salts could improve the digestion of long chain lipids by enhancing the removal of poorly water-soluble lipolytic compounds from the oil–water interface through solubilisation.44 The effect of phospholipids on the activity of pancreatic lipase/colipase is affected by a number of factors, such as concentration of substrates, each amphiphile, the ratio of colipase and inhibitors presence.45 In the present study, the net effect of bile salt/phospholipid addition at a fixed ratio was found to stimulate long chain lipids digestion. In contrast to digestion of emulsions with long chain lipid, the digestion profiles of emulsions with medium chain lipids under fasted-state were similar to fed-state conditions. As described previously, medium chain lipid lipolysis products could rapidly dissociate from the digesting interface through either being incorporated into micellar structure or precipitating as fatty acid soaps. The dispersion of medium chain lipid digestion products into the digestion medium could happen even in the absence of bile salt.34 Therefore, the differences in the concentration of bile salts between fasted- and fed-state digestion medium did not exhibit much influence on the digestion profiles of emulsions with MCT.
3.5 Influence of emulsion type on in vitro digestion
The in vitro digestion of curcumin encapsulated in milled cellulose stabilized Pickering emulsions and control emulsions containing same amount of curcumin were studied using a pH-stat lipolysis model. The volume mean droplet size of conventional emulsions with MCT, canola and soy bean oil stabilized by Tween 80 and Span 80 were around 19 ± 7, 16 ± 6, 23 ± 9 μm (Table 2, Fig. 5), which were smaller than that of Pickering emulsions. The initial surface area of conventional emulsions with MCT, canola oil and soy bean oil for lipolysis was 2.4, 1.7 and 1.8 times their counterparts stabilized by milled cellulose. The digestion of conventional emulsions showed a more rapid increase in the hydrolysis of triglyceride in the first 10 min followed by a slower increase than the corresponding Pickering emulsions (Fig. 3). The faster initial digestion rate of emulsions stabilized by surfactants than the corresponding Pickering emulsion samples could be attributed to the increase in the surface area of lipid phase exposed to the lipase.46 The total extent of lipolysis of Pickering emulsions stabilized by milled cellulose was higher compared to conventional emulsions stabilized by small molecular surfactant with the same lipid phase, except for emulsions with MCT, which reached complete hydrolysis for both emulsions. The deviations in the digestion profiles of Pickering and conventional emulsions might be due to the differences in the ability of emulsifiers in hindering the access of lipase to oil droplets. The rate of lipolysis would rely on access of lipase to the oil droplets interface as well as the removal of surface active molecules from the interface, which originally existed in the system or formed during lipolysis. When Span/Tween 80 stabilized emulsions were incubated with lipolysis buffer, there appeared to be some coalesced droplets present (Fig. 5), which could be due to the displacement of some Span/Tween 80 molecules from the droplet surfaces by bile salts in the buffer, thus enhancing the binding between lipase and partially bile salts coated droplets. This could explain the rapid digestion of Span/Tween 80 stabilized emulsion during the first few minutes of lipolysis. With the prolonging of lipolysis process, the digestion rate reached a fairly constant value after certain time even though not all lipids had been digested. This phenomenon might be attributed to the inhibition effect of Span/Tween 80 molecules since non-ionic surfactants have been reported be more surface-active than lipase, which could inhibit the binding of lipase to oil droplet surface.47 Also, the released fatty acids during lipolysis would reduce the lipase activity.48 As surface active molecules, fatty acids would compete for the oil–water interface with lipases, which limited the contact between lipase and emulsified lipids. At the late stage of lipolysis, there was a reduced concentration of available bile salts to remove accumulated fatty acids and promote binding between lipase to the surface of oil droplets. Therefore, lower extent of lipoysis was observed in the late stage lipolysis of Span/Tween 80 stabilized emulsions than in corresponding Pickering emulsions. The results in the current study could suggest that large surface area could promote the digestion of lipid phase in Span/Tween 80 stabilized emulsions at the beginning of the lipolysis, and the inhibition effect from the non-ionic surfactants could diminish the total extent of lipolysis of Span/Tween 80 stabilized emulsions compared to milled cellulose stabilized Pickering emulsions with similar droplet size. However, for emulsions with smaller droplet size, like nanoemulsions, the inhibition effect of non-ionic surfactants on the lipolysis process might be different due to the dramatically large surface area of these emulsions.
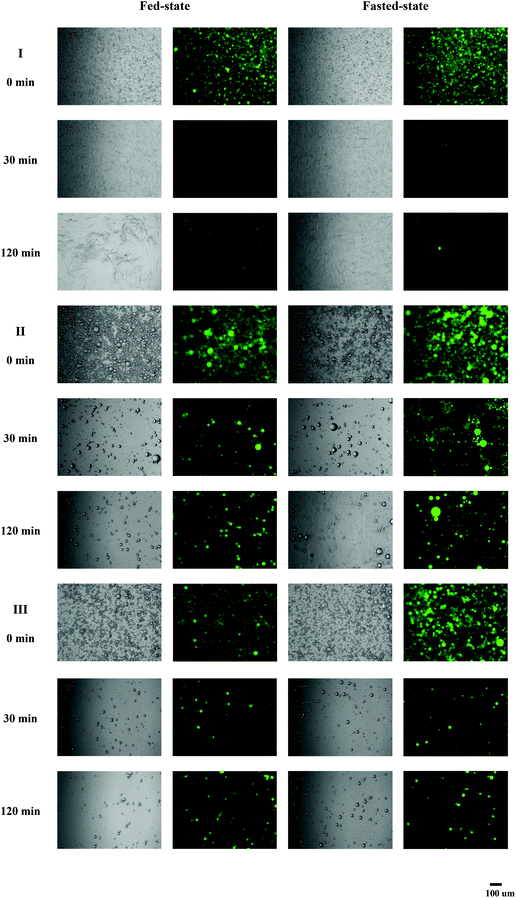 |
| Fig. 5 Microscopic and fluorescence images of control emulsion containing different oil phases incubated in in vitro lipolysis at different time. (I) MCT, (II) soy bean oil, and (III) canola oil. | |
3.6 Influence of oil type, digestion buffer & emulsion type on in vitro bioaccessibility
After ultracentrifugation, the digests of emulsions containing long chain lipids were separated into an upper oily phase, an aqueous phase and a precipitated pellet phase. And the aqueous phase was separated into two layers: an opaque paste-like layer and a clear layer in digests of emulsions containing canola and soy bean oil under fed conditions (Fig. 6B and C). As digestion of emulsions with MCT was effectively complete and independent of bile salt concentration, the lipolytic products were separated into an aqueous phase and a pellet fraction, without an oily phase, post ultracentrifugation (Fig. 6A). The pellets principally contained milled cellulose particles, insoluble crystalline calcium soaps and bile salts. The oil phase mainly contained undigested triglyceride and diglyceride. With the interaction with surface active bile salts, the lipolytic materials (mainly monoglycerides and fatty acids) would produce multilamellar liquid crystalline phases, which could gradually detach from the surface of the oil droplets to form multilamellar vesicles. These multilamellar vesicles then underwent a transformation initially to first unilamellar vesicles and later to mixed micelles upon the bile salt interaction.49 The aqueous phase obtained after in vitro digestion contained both mixed micelles and unilamellar liquid-crystalline vesicles, which were composed of bile salts, fatty acids, monoglycerides and phospholipids.50 However, the solubilization capacity of micellar and vesicular species were significantly affected by the lipid species and buffer conditions. The overall solubility of curcumin in the aqueous phase under different conditions were quantified and characterized later in this study.
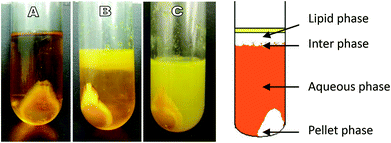 |
| Fig. 6 Different phases present after ultracentrifugation of lipolysis samples. (A) Pickering emulsion containing MCT oil under fed-state lipolysis, (B) Pickering emulsion containing soy bean oil under fed-state lipolysis, (C) Pickering emulsion containing soy bean oil under fasted-state lipolysis. | |
The solubility and dissolution rate of lipophilic compounds could be significantly improved after being administrated in a lipid formulation compared to a simple suspension formulation. During lipid digestion, lipophilic compounds are trafficked from digesting triglyceride droplets, via lipolytic products, monoglycerides and diglycerides existing on droplet surface, to the dispersed vesicular and micellar phases from where absorption is thought to occur.51 The fraction of curcumin released in the vesicular and micellar phases available for absorption is defined as bioaccessibility. Summary of extent of lipolysis and bioaccessbility of curcumin in Pickering emulsion and control emulsion are presented in Fig. 7. The distribution of curcumin in different phases of centrifuged lipolytic buffer (oil phase, aqueous phase and precipitated pellets) is displayed in Table 3. The curcumin in the aqueous phase reflected the amount of curcumin incorporated into the vesicular and micellar system, which represented the bioaccessibility of curcumin after digestion. The digestion of Pickering emulsion with MCT was complete. However, the digests of MCT provided poor solubilizing conditions for curcumin. With the absence of residual oil phase to maintain curcumin in lipid phase, precipitation of curcumin from the digestion buffer was observed, leading to a significant proportion of curcumin being recovered in the pellet phase. The digestion of emulsions with long chain triglycerides (canola and soy bean oil) was incomplete and an undigested oil phase existed under current experimental conditions, resulting in retention of curcumin in the oil phase and reduced curcumin diffusion into the aqueous phase. The bioaccessibility of curcumin in Pickering emulsions with long chain triglycerides was much lower than that of Pickering emulsions with MCT. The concentration of bile salts/phospholipids had a major influence on curcumin solubilization in the Pickering emulsion digests. The ratio of curcumin attained in the aqueous phase post-digestion was higher in digests conducted under fed state when compared to under fasted state. And the precipitation of curcumin during lipolysis in fasted state was more prominent than in fed state, which might be due to the high concentration of bile salts/phospholipids in the lipolysis buffer of fed state enhancing the incorporation of curcumin into the micellar phase.
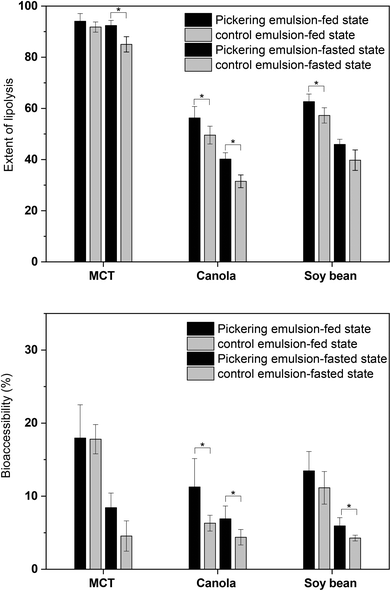 |
| Fig. 7 Extent of lipolysis and bioaccessbility of curcumin in Pickering emulsion and control emulsion. * indicates that the difference is significant (p < 0.05). | |
Table 3 The distribution of curcumin in different phases of lipolysis samples after ultracentrifugation
Lipid |
Fed state |
Fasted state |
% in oil |
% in APa |
% in pellet |
% in oil |
% in AP |
% in pellet |
AP: Aqueous phase. Different characters (a–c) represent significant difference at p < 0.05 level among the same column. |
Pickering emulsion |
MCT |
None |
78.0 ± 4.5d |
17.5 ± 3.5b |
None |
61.4 ± 2.0c |
34.8 ± 2.0b |
Soy bean oil |
62.4 ± 5.8ab |
13.5 ± 2.7bc |
24.1 ± 3.1bc |
44.0 ± 3.9a |
5.9 ± 1.1a |
50.1 ± 2.8c |
Canola oil |
53.5 ± 6.3a |
11.3 ± 3.9b |
35.2 ± 2.4cd |
46.5 ± 4.3a |
6.9 ± 1.7a |
46.6 ± 2.6c |
Convention emulsion |
MCT |
None |
67.8 ± 2.0c |
27.8 ± 2.7c |
None |
56.6 ± 2.1b |
38.3 ± 2.5b |
Soy bean oil |
72.2 ± 5.3b |
11.1 ± 2.2b |
16.7 ± 3.1ab |
80.4 ± 4.2b |
4.3 ± 0.5a |
14.3 ± 3.7a |
Canola oil |
81.3 ± 4.0bc |
6.3 ± 1.1a |
12.4 ± 2.9a |
86.0 ± 2.7b |
4.4 ± 1.1a |
9.6 ± 1.6a |
The transporting of curcumin from the Pickering emulsions was related to the extent of lipolysis as the percentage of curcumin existing in the aqueous phase was well correlated with the extent of lipolysis. However, compared with Pickering emulsions, the ratio of curcumin contained in the pellet was low, and a large portion of curcumin was concentrated in the remaining undigested oil phase during the digestion of conventional emulsions with the corresponding oil type. The low bioaccessibility of curcumin in conventional emulsions might be attributed to two factors, the lower extent of lipolysis and the existence of polar compounds. With less amount of oil droplets were hydrolysis during lipolysis, less curcumin was released into the aqueous phase. Moreover, since the addition of more polar compounds (Span 20, Span 40, Span 60, Span 80 and monoolein), was found to enhance the solubility of curcumin in the oil phase,52 the present of Span 80 in the conventional emulsions might promote the solubilization of released curcumin into the undigested oil phase. The influence of Span 80 on curcumin solubility might also explain the low concentration of curcumin in the pellets of digested conventional emulsions.
These data suggested that the relative solubilization capacity of digested emulsions was highly dependent on the type of lipid, concentration of bile salts/phospholipids used, and that the emulsifiers utilized in the formulations was critical to effective delivery of the bioactive compounds. Milled cellulose stabilized Pickering emulsions might be a more effective approach for oral delivery of lipophilic bioactive compounds than conventional emulsions stabilized by non-ionic surfactant with similar droplet size. However, the influence of different emulsifiers used on the formation of colloidal species and the solubilization behavior of encapsulated lipophilic compounds during the digestion process still needs to be revealed by further studies.
4. Conclusion
The current study demonstrates the potential of utilizing milled cellulose stabilized Pickering emulsions as a novel food-grade formulation for the encapsulation and delivery of lipophilic bioactive compounds. Great stability of curcumin in the Pickering emulsions with different lipid phases was observed in a storage period of about a month. The in vitro digestion of Pickering emulsions was highly dependent on the type of lipids and buffer conditions used. Pickering emulsions with MCT reached complete digestion under both low and high bile salts/phospholipid conditions. However, a significant amount of curcumin precipitated during the digestion process. Digestion of Pickering emulsions with long chain triglycerides (soy bean and canola oil) was incomplete and more dependent on bile salt/phospholipid concentration. Although the ratio of precipitated curcumin decreased, the bioaccessibility was lower than that of emulsions with MCT. Due to the inhibition effect from surface active non-ionic surfactants, the extent of lipolysis of Pickering emulsions was higher than the corresponding conventional emulsions stabilized by Span/Tween 80 despite their higher initial digestion speed. And the bioaccessibility of curcumin encapsulated in Pickering emulsions was higher than in conventional emulsions. These results suggest that Pickering emulsion stabilized by milled cellulose might be a great encapsulation formulation for oral delivery of lipophilic bioactive compounds. Further studies on investigating the correlation between in vitro bioaccessibility and in vivo bioavailability of the encapsulated active ingredient in this system would be vital to reveal the delivery capacity of the Pickering emulsions in vivo.
Conflicts of interest
We have no conflict of interest in this research.
References
- S. M. Loveday and H. Singh, Trends Food Sci. Technol., 2008, 19, 657–668 CrossRef CAS.
- B. M. Folmer, D. Barron, E. Hughes, L. Miguet, B. Sanchez, O. Heudi, M. Rouvet, L. Sagalowicz, P. Callier and M. Michel, Biochem. Pharmacol., 2009, 78, 1464–1474 CrossRef CAS.
- D. J. McClements and Y. Li, Adv. Colloid Interface Sci., 2010, 159, 213–228 CrossRef CAS.
- D. J. McClements, Annu. Rev. Food Sci. Technol., 2010, 1, 241–269 CrossRef CAS PubMed.
- D. Tamayo-Esquivel, A. Ganem-Quintanar, A. Martinez, M. Navarrete-Rodriguez, S. Rodriguez-Romo and D. Quintanar-Guerrero, J. Nanosci. Nanotechnol., 2006, 6, 3134–3138 CrossRef CAS PubMed.
- W. N. Charman, C. J. H. Porter, S. Mithani and J. B. Dressman, J. Pharm. Sci., 1997, 86, 269–282 CrossRef CAS PubMed.
- A. J. Humberstone and W. N. Charman, Adv. Drug Delivery Rev., 1997, 25, 103–128 CrossRef CAS.
- R. Aveyard, B. P. Binks and J. H. Clint, Adv. Colloid Interface Sci., 2003, 100, 503–546 CrossRef.
- B. P. Binks, Curr. Opin. Colloid Interface Sci., 2002, 7, 21–41 CrossRef CAS.
- R. V. Tikekar, Y. J. Pan and N. Nitin, Food Res. Int., 2013, 51, 370–377 CrossRef CAS.
- P. E. Ruiz-Rodriguez, D. Meshulam and U. Lesmes, Food Biophys., 2014, 9, 406–415 CrossRef.
- S. Simovic, H. Hui, Y. M. Song, A. K. Davey, T. Rades and C. A. Prestidge, J. Controlled Release, 2010, 143, 367–373 CrossRef CAS.
- R. Peters, E. Kramer, A. G. Oomen, Z. E. H. Rivera, G. Oegema, P. C. Tromp, R. Fokkink, A. Rietveld, H. J. P. Marvin, S. Weigel, A. A. C. M. Peijnenburg and H. Bouwmeester, ACS Nano, 2012, 6, 2441–2451 CrossRef CAS.
- H. A. Wege, S. Kim, V. N. Paunov, Q. X. Zhong and O. D. Velev, Langmuir, 2008, 24, 9245–9253 CrossRef CAS.
- I. Kalashnikova, H. Bizot, P. Bertoncini, B. Cathala and I. Capron, Soft Matter, 2013, 9, 952–959 RSC.
- H. Ougiya, K. Watanabe, Y. Morinaga and F. Yoshinaga, Biosci., Biotechnol., Biochem., 1997, 61, 1541–1545 CrossRef CAS.
- S. Elazzouzi-Hafraoui, Y. Nishiyama, J. L. Putaux, L. Heux, F. Dubreuil and C. Rochas, Biomacromolecules, 2008, 9, 57–65 CrossRef CAS PubMed.
- J. J. Blaker, K. Y. Lee, X. X. Li, A. Menner and A. Bismarck, Green Chem., 2009, 11, 1321–1326 RSC.
- L. Wagberg, G. Decher, M. Norgren, T. Lindstrom, M. Ankerfors and K. Axnas, Langmuir, 2008, 24, 784–795 CrossRef.
- M. Andresen and P. Stenius, J. Dispersion Sci. Technol., 2007, 28, 837–844 CrossRef CAS.
- H. Y. Kuo, S. H. Chen and A. I. Yeh, J. Agric. Food Chem., 2014, 62, 742–749 CrossRef CAS PubMed.
- L. H. Chiang, S. H. Chen and A. I. Yeh, J. Agric. Food Chem., 2012, 60, 12332–12340 CrossRef CAS PubMed.
- X. Lu, Y. Wang, Y. Li and Q. Huang, Food Hydrocolloids, 2018, 84, 47–57 CrossRef CAS.
- A. I. Yeh, Y. C. Huang and S. H. Chen, Carbohydr. Polym., 2010, 79, 192–199 CrossRef CAS.
- X. Lu, H. Zhang, Y. Li and Q. Huang, Food Hydrocolloids, 2018, 77, 427–435 CrossRef CAS.
- A. B. Kunnumakkara, P. Anand and B. B. Aggarwal, Cancer Lett., 2008, 269, 199–225 CrossRef CAS PubMed.
- C. Ireson, S. Orr, D. J. L. Jones, R. Verschoyle, C. K. Lim, J. L. Luo, L. Howells, S. Plummer, R. Jukes, M. Williams, W. P. Steward and A. Gescher, Cancer Res., 2001, 61, 1058–1064 CAS.
- C. R. Ireson, D. J. Jones, S. Orr, M. W. Coughtrie, D. J. Boocock, M. L. Williams, P. B. Farmer, W. P. Steward and A. J. Gescher, Cancer Epidemiol., Biomarkers Prev., 2002, 11, 105–111 CAS.
- Y. Kaminaga, A. Nagatsu, T. Akiyama, N. Sugimoto, T. Yamazaki, T. Maitani and H. Mizukami, FEBS Lett., 2003, 555, 311–316 CrossRef CAS.
- R. A. Sharma, A. J. Gescher and W. P. Steward, Eur. J. Cancer, 2005, 41, 1955–1968 CrossRef CAS.
- A. Mukerjee and J. K. Vishwanatha, Formulation, Anticancer Res., 2009, 29, 3867–3875 CAS.
- X. Y. Wang, Y. Jiang, Y. W. Wang, M. T. Huang, C. T. Ho and Q. R. Huang, Food Chem., 2008, 108, 419–424 CrossRef CAS PubMed.
- H. L. Yu and Q. R. Huang, Food Chem., 2010, 119, 669–674 CrossRef CAS.
- L. Sek, C. J. H. Porter, A. M. Kaukonen and W. N. Charman, J. Pharm. Pharmacol., 2002, 54, 29–41 CrossRef CAS PubMed.
- C. J. H. Porter, N. L. Trevaskis and W. N. Charman, Nat. Rev. Drug Discovery, 2007, 6, 231–248 CrossRef CAS PubMed.
- G. A. Kossena, B. J. Boyd, C. J. H. Porter and W. N. Charman, J. Pharm. Sci., 2003, 92, 634–648 CrossRef CAS PubMed.
- Y. W. Ting, Y. K. Jian, Y. Q. Lan, C. X. Xia, Z. Y. Lin, M. A. Rogers and Q. R. Huang, Mol. Pharmaceutics, 2015, 12, 2229–2236 CrossRef CAS PubMed.
- Y. J. Wang, M. H. Pan, A. L. Cheng, L. I. Lin, Y. S. Ho, C. Y. Hsieh and J. K. Lin, J. Pharm. Biomed., 1997, 15, 1867–1876 CrossRef CAS PubMed.
- A. Marefati, M. Bertrand, M. Sjöö, P. Dejmek and M. Rayner, Food Hydrocolloids, 2017, 63, 309–320 CrossRef CAS.
- M. H. Leung, H. Colangelo and T. W. Kee, Langmuir, 2008, 24, 5672–5675 CrossRef CAS PubMed.
- C. Chen, T. D. Johnston, H. Jeon, R. Gedaly, P. P. McHugh, T. G. Burke and D. Ranjan, Int. J. Pharm., 2009, 366, 133–139 CrossRef CAS PubMed.
- K. Ahmed, Y. Li, D. J. McClements and H. Xiao, Food Chem., 2012, 132, 799–807 CrossRef CAS.
- D. P. Cistola, J. A. Hamilton, D. Jackson and D. M. Small, Biochemistry, 1988, 27, 1881–1888 CrossRef CAS PubMed.
- K. J. MacGregor, J. K. Embleton, J. E. Lacy, E. A. Perry, L. J. Solomon, H. Seager and C. W. Pouton, Adv. Drug Delivery Rev., 1997, 25, 33–46 CrossRef CAS.
- A. Lykidis, A. Avranas and P. Arzoglou, Comp. Biochem. Physiol., Part B: Biochem. Mol. Biol., 1997, 116, 51–55 CrossRef CAS.
- Y. Li and D. J. McClements, J. Agric. Food Chem., 2010, 58, 8085–8092 CrossRef CAS.
- Y. Gargouri, R. Julien, A. G. Bois, R. Verger and L. Sarda, J. Lipid Res., 1983, 24, 1336–1342 CAS.
- Y. Pafumi, D. Lairon, P. L. de la Porte, C. Juhel, J. Storch, M. Hamosh and M. Armand, J. Biol. Chem., 2002, 277, 28070–28079 CrossRef CAS PubMed.
- O. Hernell, J. E. Staggers and M. C. Carey, Biochemistry, 1990, 29, 2041–2056 CrossRef CAS PubMed.
- G. A. Kossena, B. J. Boyd, C. J. H. Porter and W. N. Charman, J. Pharm. Sci., 2003, 92, 634–648 CrossRef CAS PubMed.
- C. J. H. Porter, A. M. Kaukonen, A. Taillardat-Bertschinger, B. J. Boyd, J. M. O'Connor, G. A. Edwards and W. N. Charman, J. Pharm. Sci., 2004, 93, 1110–1121 CrossRef CAS PubMed.
- H. L. Yu, K. Shi, D. Liu and Q. R. Huang, Food Chem., 2012, 131, 48–54 CrossRef CAS.
|
This journal is © The Royal Society of Chemistry 2020 |
Click here to see how this site uses Cookies. View our privacy policy here.