DOI:
10.1039/C9FO01740B
(Paper)
Food Funct., 2020,
11, 153-162
Chronic oral exposure to glycated whey proteins increases survival of aged male NOD mice with autoimmune prostatitis by regulating the gut microbiome and anti-inflammatory responses†
Received
31st July 2019
, Accepted 22nd November 2019
First published on 26th November 2019
Abstract
Glycated whey proteins have been shown to be protective against type 1 diabetes in our previous studies, suggesting their potential application as medical food. To determine if the protection could be extended to other autoimmune diseases, aged male non-obese diabetic (NOD) mice that develop a wide spectrum of autoimmune pathologies, including spontaneous autoimmune prostatitis, were used. After a 6-month oral exposure to whey protein-derived early glycation products (EGPs), EGP-treated NOD mice had an increased survival rate, decreased macrophage infiltration in the anterior lobe and decreased inflammation in the prostate when compared to the mice that received non-reacted controls. The systemic immunity was regulated towards anti-inflammation, evidenced by an increase in serum IL-10 level and decreases in total splenocytes, splenic M1 macrophages, CD4+ T cells, CD8+ T cells and B cells. Consistent with an overall anti-inflammatory status, the gut microbiome was altered in abundance but not diversity, with increased Allobaculum, Anaerostipes, Bacteroides, Parabacteroides and Prevotella and decreased Adlercreutzia and Roseburia at the genus level. Moreover, increased Bacteroides acidifaciens correlated with most of the immune parameters measured. Collectively, chronic oral exposure to EGPs produced an anti-inflammatory effect in aged male NOD mice, which might contribute to the protective effects against spontaneous autoimmune prostatitis and/or other organ specific autoimmune diseases.
Introduction
Chronic prostatitis/chronic pelvic pain syndrome (CP/CPPS), category III prostatitis classified by NIH, has a prevalence of over 90% among symptomatic patients with prostatitis,1 and it can be further divided into IIIA and IIIB. IIIA CP/CPPS is an inflammatory subtype characterized by the presence of leukocytes in expressed prostatic secretions, post prostatic massage urine or semen.1 The autoimmune process and local pro-inflammation were proposed as two important mechanisms for CP/CPPS, although other reasons (e.g., endocrine dysfunctions) also matter.2,3 Traditional nonsteroidal anti-inflammatory drugs are effective in inhibiting the inflammatory process and managing pain for CP/CPPS patients,4 but anti-inflammatory therapies are not recommended as monotherapy for treating prostatitis, and a multimodal therapeutic regimen may be used.5 Herein, dietary supplements with anti-inflammatory properties are gaining popularity in the treatment of CP/CPPS.4
Glycated food proteins or dietary early glycation products (EGPs) are Amadori or Heyns compounds produced during rearrangement in the Maillard reaction/glycation. They have enhanced functional properties, such as increased solubility and thermostability.6 Along with their well-established physiochemical properties, their immunoregulatory effects have been under investigation. Our previous studies showed that whey protein derived early glycation products were able to polarize macrophages toward anti-inflammatory M2 both in vitro7 and in vivo.8 Sub-chronic oral exposure to these EGPs reduced type 1 diabetes (T1D) incidence in female non-obese diabetic (NOD) mice, and the mechanisms were through increasing anti-inflammatory responses and decreasing autoantibody generation.9 These findings suggest that EGPs are anti-inflammatory and may have potential as a functional food for patients with chronic prostatitis caused by inflammation.
The animal model employed in this study was male NOD mouse. This murine strain spontaneously develops autoimmune T1D at an early age and shares similarities with human T1D development in early adolescence. However, aged male NOD mice exhibit a wide spectrum of autoimmune pathologies distributed through a variety of organs.10 Intra-prostatic inflammatory infiltration is well established by about 20 weeks of age.11 In a comparison of NOD to other five stains (i.e., BALB/c, B10, NZB, MRL, and SWR) regarding prostatic inflammation, NOD males exhibit the highest inflammation score spontaneously.12 In this study, non-diabetic NOD males (>4 months) were orally exposed to EGPs or non-reacted (NR) controls for up to 6 months to determine their effects on prostatic inflammation and associated mechanisms (e.g., cytokines/chemokines and gut microbiome).
Materials and methods
EGP preparation
The whey protein derived EGPs were prepared as described before.7 In brief, whey protein isolate (WPI, Fonterra (USA) Inc, Rosemont, IL) and glucose (Sigma-Aldrich, St Louis, MO) were dissolved in distilled water at a molar ratio of free amino groups to reducing ends of 1
:
2. The solution was freeze-dried and incubated in a desiccator containing saturated aqueous Mg(NO3)2 solution at 55 °C for 8 h. LC-MS showed that all the whey proteins were modified by glucose, and the reaction had not progressed to later stages.9
Mouse model of autoimmune prostatitis and the treatment regimen
NOD males were obtained from Taconic Biosciences (Rensselaer, NY) and housed in the Coverdell Vivarium at the University of Georgia (UGA). The room was maintained on a 12 h dark/light cycle at 21–24 °C with 20–60% relative humidity. The mice had access to food (Diet 5053, PicoLab® Rodent Diet 20, LabDiet, MO) and filtered tap water ad libitum. Blood glucose levels were measured from sampling caudal venous tail blood with a Bayer's CONTOUR blood glucose meter (Bayer Health Care LLC, Mishawaka, IN). Diabetic mice were identified as those with a blood glucose level >200 mg dL−1 for two consecutive weeks. At the study termination, the mice were euthanized by CO2 inhalation followed by cervical dislocation. The animal experiments were performed in accordance with the Public Health Service (PHS) policy on humane care and use of laboratory animals and the guide for the care and use of laboratory animals, and approved by UGA Institutional Animal Care and Use Committee (IACUC).
Age-matched non-diabetic NOD males (approximately 4-month-old) were randomly separated into 2 groups and gavaged daily with NR and EGPs at 600 mg per kg body weight (BW) for up to 6 months. This dose was physiologically relevant as discussed previously,7 and EGPs at this dose protected NOD mice from developing T1D.9 Mice were euthanized at months 3 and 6 following the first dosing. Aged NOD males had decreased incidences of diabetes, and none of them developed T1D during the experimental period. However, for the survival study, some mice had to be euthanized during the study when the thresholds of humane endpoints were reached due to other autoimmune diseases and cancers. They were recorded as moribund events.
Histological assessment of the prostatic inflammation
The urogenital organs without seminal vesicles and partial anterior prostates were dissected and placed in 10% buffered formalin. Longitudinal cuts at every 1–2 mm were made, and the sections were processed for hematoxylin and eosin (H&E) staining by the Histology Laboratory (College of Veterinary Medicine, UGA). The inflammation in 4 prostatic lobes (dorsal, lateral, anterior and ventral) was scored by a board-certified veterinary pathologist. The scoring system was described in detail by Haverkamp et al.: 0, no inflammation; 1, mild inflammation; 2, moderate inflammation; 3, severe inflammation.13 The mouse prostatic lobes were identified according to the histological guidelines introduced by Oliveira et al.14
Flow cytometric analysis
The preparation of single-cell suspensions was similar to the procedures described before.9 A section was dissected from the anterior prostate (AP), placed into PBS, and digested by 0.05 mg ml−1 collagenase (Sigma-Aldrich) and 5 U ml−1 DNase (Sigma-Aldrich) at 37 °C for 30–45 min. The mixture was centrifuged at 300g at 4 °C for 8 min, and the pellet was resuspended in PBS and passed through a 40-μm cell strainer with a fresh tube placed underneath to collect the single-cell suspension. The spleen was mashed using the frosted ends of two microscope slides, with the red blood cell lysed by adding ACK lysing buffer (Gibco by Life Technologies, Orand Island, NY) for 5 min.
Splenocytes and AP cells were stained for the following surface markers conjugated with fluorophores: CD3 (145-2C11, PerCP-Cy5.5, BD Pharmingen), CD4 (RM4-5, V450, BD Horizon), CD8 (53-6.7, APC-H7, BD Pharmingen), F4/80 (BM8, FITC, eBioscience), CD80 (16-10A1, PE-CD594, BD Horizon), CD209 (LWC06, Alexa Fluor, Novus Biologicals), and B220 (RA3-6B2, FITC, eBioscience). After adding the antibodies, cells were incubated at 4 °C in the dark for 30 min. The cells were washed and acquired on a Becton Dickinson LSRII Flow Cytometer (BD Biosciences). The flow cytometric data were analyzed using FlowJo v10 (FlowJo, LLC, Ashland OR). Leukocytes were characterized as CD4+ T cells (CD3+CD4+CD8−), CD8+ T cells (CD3+CD4−CD8+), macrophages (F4/80+), M1 (F4/80+CD80+CD209−), M2 (F4/80+CD80−CD209+), and B cells (B220+).
Hematology
Hematology was conducted after 6-month treatment. A volume of ∼200 μl venous blood sample was drawn from a tail nick, collected in tubes with K2EDTA (BD Microtainer, Franklin Lakes, NJ), and kept on ice. The hematology analyses with differentials were performed on the same day in the Veterinary Diagnostic Laboratory at the UGA Veterinary Teaching Hospital using a HESKA Element HT5 Veterinary Analyzer (Loveland, Co).
Cytokine/chemokine quantitation
Following EGP treatment for 6 months, blood was collected from the orbital sinus of mice after being deeply anesthetized. The sera were separated and stored at −80 °C prior to use. Thirty-one cytokines/chemokines in the sera were detected using a MILLIPLEX MAP mouse cytokine/chemokine kit (Millipore, Billerica, MA) following the manufacturer's instructions, and the data were collected and analysed using Bio-Plex Manager 6.1.1. The working range was 12.8–40
000 pg ml−1 for IL-13 and 3.2–10
000 pg ml−1 for the rest, with IL-15 being out of range.
16S rRNA gene sequencing and bioinformatics analysis
The feces were collected from individual mice 6 months after initial dosing, transferred to 500 μL Eppendorf tubes and kept in a −80 °C freezer. DNA was extracted using QIAamp DNA stool mini kits (Qiagen, Valencia, CA) following the manufacturer's protocols. For library preparation, DNA was normalized to 20 ng μL−1 at Georgia Genomic Facility (GGF) and the V3-V4 region of 16S rRNA was targeted using locus-specific primers for the first round of PCR. Illumina-specific iTru_R1_5′_fusion and iTru_R2_5′_fusion Read 1 and Read 2 sequencing primers (forward: S-D-Bact-0564-a-S-15 and reverse: S-D-Bact-0785-a-A-21) were used with 20 internal tags (8 forward fusion primers and 12 reverse fusion primers) ranging from 5 nucleotides (NT) to 8 NTs long.15 The PCR mix was from Kapa Biosystems, Inc. (Boston, MA). Next, the PCR amplicon aliquot was purified and quantified using AMPure beads. The second round PCR was run using Illumina i5 and i7 primers, and sequencing was done on an Illumina Miseq (Illumina Inc., San Diego, US). Bioinformatics analysis was performed as previously described.16 The subsequent analysis was performed using Quantitative Insights IntoMicrobial Ecology (QIIME) version 1.9.0, a pipeline that worked with high-throughput 16S rRNA sequencing data.16 Linear discriminant analysis (LDA) effect size (LEfSe) analysis was used to identify significantly different taxa following the conditions on the website http://huttenhower.sph.harvard.edu/galaxy. Features that had an LDA score >2 were plotted.
Statistical analysis
The survival curve was compared using the Gehan–Breslow–Wilcoxon test, and all the immune parameters were tested using Student's t-test using GraphPad Prism 6 (GraphPad Software, San Diego, CA). The non-parametric t-test was used to test the statistical significance for α diversity, and Analysis of Similarities (ANOSIM) for β diversity. Correlation analysis was performed using XLSTAT (Addinsoft Inc., Long Island City, NY) using Spearman's correlation test.
Results
Chronic exposure to EGPs increases the survival rate and moderately reduces the prostatic inflammation
The survival rate of mice was recorded to indicate the overall effects of NR/EGPs. As shown in Fig. 1, chronic oral consumption of EGPs had an overall beneficial effect on the mice when compared to NR treatment: after 6 months of dosing, EGP treatment maintained the survival rate of the male NOD mice at ∼80%, while those dosed with NR only had a survival rate below 40%. No animals developed diabetes during the dosing period (data not shown).
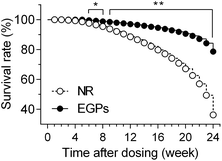 |
| Fig. 1 Survival rate of male NOD mice. The mice were orally gavaged with NR or EGPs at a dose of 600 mg per kg BW per day for 6 months. Moribund events were recorded when the mice reached the humane endpoints (n = 15–13). *, p < 0.05; **, p < 0.01. | |
To evaluate the effect of EGPs on intraprostatic inflammation, aged NOD males (>4 months) were euthanized after dosing for 3 or 6 months. Organs including the AP were harvested, and none of them showed a significant difference in weight between the NR and EGP treatment groups (ESI Table 1†). Longitudinal sections of the urogenital organs containing 4 prostatic lobes were H&E stained, and the inflammations associated with the 4 lobes were scored. Immune infiltration was detected in both dorsal and lateral prostates (Fig. 2A and B, black arrows). After 3 months of dosing, EGP treatment limited the inflammation in the dorsal prostate at the mild stage for all mice, while 2/6 mice treated with NR developed moderate inflammation. After 6 months of dosing, EGPs kept the inflammation in the dorsal prostate mostly at the mild stage (5 mild and 1 moderate), whereas the NR group had half of the mice at the mild stage and the other half at the moderate stage (Fig. 2A, last panel). For the lateral prostate (Fig. 2B), one animal had mild inflammation after 3 months of EGP treatment, which would most likely be due to the immune infiltration prior to the experiment, which could have occurred as early as 7 weeks of age.11 Prolonged treatment with EGPs for up to 6 months protected the lateral prostate from developing inflammation: no mice in the EGP group developed inflammation, while 1 mild and 1 moderate inflammatory incidence occurred in the NR group.
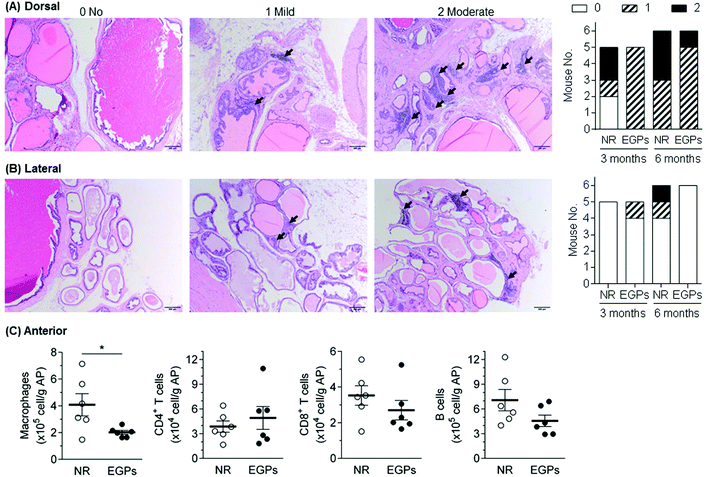 |
| Fig. 2 Chronic exposure to EGPs reduces prostatic inflammation. After dosing for 3 or 6 months, the mice were euthanized and the urogenital organs were harvested. Longitudinal sections were H&E stained, and the inflammations associated with the 4 lobes were scored. Representative images for each score are shown: black arrows indicate scattered or aggregated leukocyte infiltrates. The inflammatory statuses of NR and EGP groups at months 3 and 6 are summarized in the last panels. Immune cell infiltration was only detected for (A) dorsal and (B) lateral prostates. In addition to histological assessment, (C) the inflammatory infiltrates (i.e., macrophages, CD4+ T cells, CD8+ T cells, and B cells) in the anterior prostate (AP) after 6-month treatment were quantitated by flow cytometry (mean ± SEM, n = 5–6). *, p < 0.05. | |
No inflammation was observed for anterior and ventral prostates using histopathological analysis (data not shown). In our previous studies, however, H&E staining was shown to be less sensitive than flow cytometric analysis,9 evidenced by the subtle differences in the immune infiltration of the pancreas being successfully detected using the latter, but not the former. Therefore, the infiltrating immune cells in the anterior prostate, after being treated for 6 months, were analyzed by flow cytometry (Fig. 2C). Macrophages, CD4+ T cells, CD8+ T cells and B cells were analyzed because they were frequently reported to be associated with inflamed prostates in NOD mice.11,13 Among the analyzed cells, macrophages were significantly decreased by EGPs, while CD8+ T cells and B cells were numerically decreased. Taken together with the histopathological analysis, oral exposure to EGPs generated beneficial effects by controlling or slowing down the inflammatory progression in dorsal, lateral and anterior prostates. The inflammatory status within the ventral prostate had not been further studied due to technical issues (e.g., unable to clearly separate from other lobes). However, a previous study comparing the prostatic inflammation upon ovalbumin-specific CD8+ T cell transfer showed that the anterior, dorsal and ventral prostates responded similarly.13
EGPs alter the systemic immunity
The WBC number in the blood was slightly increased from 6.4 to 7.3 (×103 μl−1) by EGP treatment for 6 months. Neutrophil and monocyte numbers were only numerically increased, while the eosinophil number was significantly increased. On the other hand, exposure to EGPs slightly decreased the number of lymphocytes, with more differences detected for the percentage (NR 48.3% vs. EGPs 40.8% of WBCs, Fig. 3).
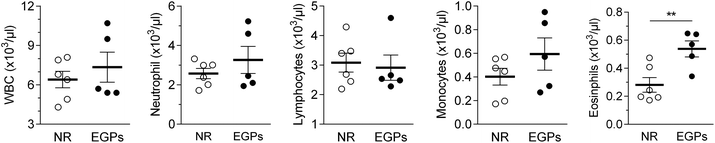 |
| Fig. 3 EGPs alter the white blood cells (WBC) in the circulation. Hematology analysis with differentials was conducted, and the numbers of WBCs, neutrophils, lymphocytes, monocytes and eosinophils were shown (mean ± SEM, n = 5–6). **, p < 0.01. One blood sample in the EGP group had clots, so the analysis of this sample was not performed. | |
The spleen weights were not significantly different between the two groups (Fig. 4A), but total splenocytes and all the measured immune populations (i.e., macrophages, M1, M2, CD4+ T cells, CD8+ T cells, and B cells) were decreased in the EGP group (Fig. 4B–E) with significant changes observed for total splenocytes, M1, CD4+ T cells, CD8+ T cells and B cells. However, the M2/M1 ratio was not significantly altered by EGP treatment (Fig. 4C).
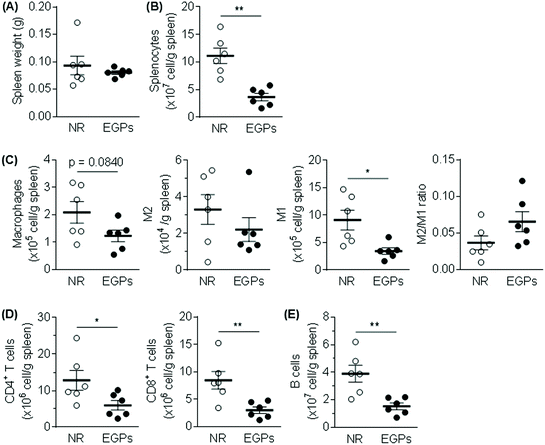 |
| Fig. 4 EGPs decrease the numbers of splenic leukocytes without altering the spleen weight. (A) The spleens were harvested and weighted. (B) The splenic leukocyte number was significantly decreased by EGPs. The analysis of macrophages, CD4+ T cells, CD8+ T cells and B cells is summarized in (C–E) (mean ± SEM, n = 6). *, p < 0.05; **, p < 0.01. | |
To further determine if EGPs generated anti-inflammatory effects, the cytokines/chemokines in sera were measured (Fig. 5). The up-regulated ones among the 31 cytokines/chemokines examined were IL-10, G-CSF, IL-3, IL-5, IL-6 and IL-17, with IL-10 showing statistically significant increases (5678.85 ± 979.60 (NR) versus 9644.43 ± 764.86 (EGPs), p = 0.0096, ESI Table 2†). IL-17 was increased 1.664 fold by EGPs, and the mean ± SE was 72.34 ± 15.24 (NR) versus 192.68 ± 114.38 (EGPs, ESI Table 2†).
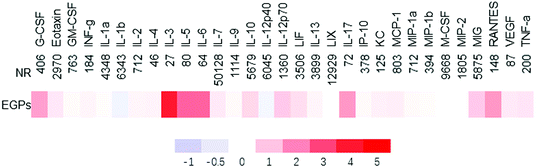 |
| Fig. 5 Heatmap of cytokines/chemokines regulated by NR and EGPs in male NOD mice treated for 6 months. The average concentrations of cytokines/chemokines of NR groups are shown (pg ml−1), and those of EGP groups are expressed as %change of NR. All averages and SEM are shown in ESI Table 2.† | |
EGPs modulate the gut microbiome
Correlations have been found between symptom scores and disease severity of CP/CPPS and the degree of dysbiosis in the gut microbiome.17 16S rRNA sequencing was used to characterize the changes in the microbiome community composition. Principal coordinate analysis of the β diversity metrics showed that the gut bacterial communities in the NR and EGP groups were well separated when taking the difference in abundance into account using weighted UniFrac (Fig. 6A). The weighted UniFrac result was supported by ANOSIM (p < 0.05 with 999 permutation), which suggested that the difference in the gut microbiome induced by EGP exposure was readily observable and well differentiated. However, EGP treatment did not show a clear pattern on the unweighted Unifrac, an index of β diversity indicating presence/absence (data not shown). Both the PD whole tree and chao1, indexes of α diversity that reflected the genetic diversity of the communities under study,18 were not significantly altered by EGP treatment (data not shown).
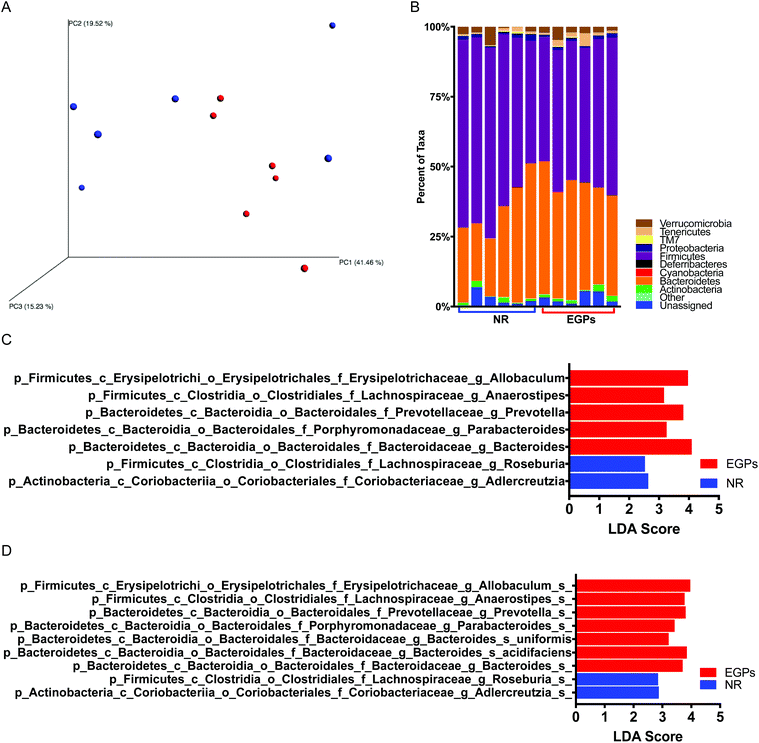 |
| Fig. 6 The composition of the gut microbiome based on 16S rRNA sequencing in NOD males treated with EGPs or NR for 6 months. (A) Weighted UniFrac beta diversity difference between NR (blue) and EGPs (red). (B) Taxonomy of the gut microbiome shown at the phylum level. Linear discriminant analysis effective size (LEfse) results for the genus level (C) and species level (D) are shown. N = 6. | |
The taxonomic profiles of the EGP versus the NR treated mice were then compared by assigning taxonomy to OTUs and subsequently splitting the OTU table into the phylogenetic level. The relative abundances of taxonomy for each treatment at the phylum level are shown in Fig. 6B, with each color representing an individual bacterial phylum. Phylum Firmicutes represented 60.0% of the total bacteria in the NR group, while in the EGP group, it represented 50.3% of the total taxa, which was significantly different from the NR group. The Firmicutes/Bacteroidetes (F/B) ratio was decreased following EGP treatment (2.19 ± 0.41 vs. 1.30 ± 0.10); however, it did not reach the level of statistical significance. When the microbial taxa at the class level were compared, EGP treatment increased Erysipelotrichia and decreased Coriobacteriia in terms of relative abundance. When the microbial taxa at the order level were compared, EGP treatment increased Erysipelotrichales and decreased Coriobacteriales in terms of relative abundance. When the microbial taxa at the family level were compared, EGP treatment increased Porphyromonadaceae, Prevotellaceae, Erysipelotrichaceae and Bacteroidaceae and decreased Coriobacteriaceae in terms of relative abundance (data not shown). When the microbial taxa at the genus level were compared, EGP treatment increased Anaerostipes, Parabacteroides, Prevotella, Allobaculum and Bacteroides and decreased Adlercreutzia and Roseburia in terms of relative abundance (Fig. 6C). When the microbial taxa at the species level were compared, EGP treatment increased Allobaculum sp., Anaerostipes sp., Prevotella sp., uniformis sp., acidifaciens sp. and Bacteroides sp. and decreased Adlercreutzia sp. and Roseburia sp. in terms of relative abundance (Fig. 6D).
Correlation between immunity and the gut microbiome
Fig. 7 illustrates the relationships between pairs of the variables that were significantly altered following EGP treatment. Spearman's correlation analysis revealed that the number of prostatic macrophages positively correlated with total splenocytes, splenic M1 macrophages, CD4+ T cells, CD8+ T cells and B cells and negatively correlated with blood eosinophils, serum IL-10 and Bacteroides at the genus level (Fig. 7A). When Bacteroides at the species level was further analyzed, the number of prostatic macrophages negatively correlated with Bacteroides acidifaciens and another unidentified Bacteroides species (Fig. 7B). Blood eosinophils positively correlated with serum IL-10, Prevotella and Anaerostipes at the genus level and Bacteroides uniformis at the species level and negatively correlated with total splenocytes, splenic M1 macrophages, CD4+ T cells, CD8+ T cells and B cells (Fig. 7A and B). The number of total splenocytes positively correlated with splenic M1 macrophages, CD4+ T cells, CD8+ T cells and B cells and negatively correlated with serum IL-10 and Bacteroides, Parabacteroides, Prevotella and Anaerostipes at the genus level and Bacteroides uniformis and acidifaciens at the species level (Fig. 7A and B). Splenic M1 macrophages positively correlated with splenic CD4+ T cells, CD8+ T cells and B cells and negatively correlated with serum IL-10, Bacteroides and Parabacteroides at the genus level and Bacteroides uniformis and acidifaciens at the species level (Fig. 7A and B). Splenic CD4+ T cells positively correlated with splenic CD8+ T cells and B cells and negatively correlated with serum IL-10 and Bacteroides at the genus level and Bacteroides acidifaciens at the species level (Fig. 7A & B). Bacteroides positively correlated with Prevotella at the genus level (Fig. 7A). Parabacteroides positively correlated with Prevotella and Allobaculum at the genus level (Fig. 7A). Prevotella positively correlated with Anaerostipes at the genus level (Fig. 7A). Taken together, the correlation analysis showed that all the significantly altered immune parameters were correlated to each other, and their further correlation with the gut microbe suggested an overall anti-inflammatory feature.
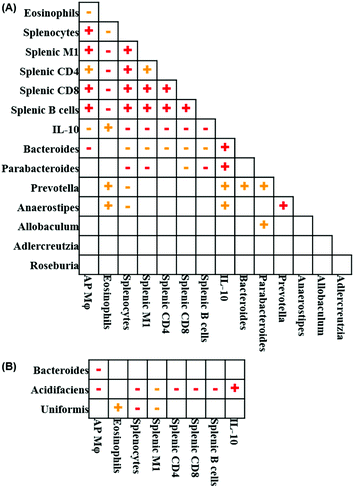 |
| Fig. 7 Correlation analysis using Spearman's correlation test. (A) Significantly regulated immune endpoints and microbes at the genus level were correlated. (B) Significantly regulated Bacteroides sp. were correlated with immune endpoints. Positive correlation, (p < 0.05), (p < 0.01); negative correlation, (p < 0.05), (p < 0.01). | |
Discussion
In this work, the chronic effects of EGPs were studied in aged non-diabetic NOD males; the survival rate of these NOD mice was significantly increased by EGP treatment (Fig. 1), which might be related to a systemic anti-inflammatory effect generated by EGPs. EGPs decreased total splenocytes, splenic M1 macrophages, CD4+ T cells, CD8+ T cells and B cells (Fig. 4), while increasing the serum IL-10 level (Fig. 5). Upregulation of anti-inflammatory cytokine IL-10 by EGPs was previously observed in human macrophage culture7 as well as in NOD females9 and C57BL/6 males subcutaneously transplanted with TRAMP-C2 prostate cancer cells.8 Interestingly, IL-10 has been shown to prevent aging-associated inflammation and insulin resistance19 and aging-induced endothelial dysfunction.20 Moreover, IL-10 might mediate its anti-inflammatory effect by metabolically reprogramming macrophages,21 and decreased IL-10 could result in enhanced mortality from Gram-negative sepsis.22 However, the Th2 response is also characterized by the production of IL-10, which may contribute to the immediate hypersensitivity response and eosinophil influx.23 It has been shown that certain Prevotella species promote the differentiation of Th17 cells, which synergizes with eosinophils to accelerate multiple myeloma progression.24 Further studies should be conducted to determine the effects of EGP-induced increases in blood eosinophils and IL-17.
Aged male NOD mice develop a wide spectrum of organ specific autoimmune diseases,10 such as autoimmune prostatitis, which was characterized by leukocyte infiltration in the prostate gland.11 We have found that EGP treatment moderately decreased prostatic inflammation (Fig. 2). In addition, flow cytometric analysis suggested that the infiltrating immune cells, especially macrophages, in the anterior prostate were decreased by EGPs. The number of prostatic macrophages positively correlated with total splenocytes, splenic M1 macrophages, CD4+ T cells, CD8+ T cells and B cells and negatively correlated with the levels of serum IL-10 and gut Bacteroides (Genus). Specifically, the number of prostatic macrophages negatively correlated with Bacteroides acidifaciens and another unidentified Bacteroides species. Bacteroides uniformis, which was also increased by EGPs, could induce higher IL-10 production from Raw264.7 macrophages than other Bacteroides strains.25 However, the function of IL-10 in prostatitis is still being debated. Although several cytokines and chemokines including IL-10 were significantly increased in the seminal plasmas of patients with CP/CPPS,26,27 peripheral IL-10 was not significantly changed in these patients.28 Further studies by measuring cytokines in the seminal fluid following EGP treatment are warranted.
Only 3–10% protein-bound Amadori products were absorbed in the intestine after ingestion.29 After eating a single meal containing fructoselysine, the human fecal secretion of fructoselysine was 2.6–5.6%.30 Thus, it could be assumed that about 90% of orally consumed EGPs were decomposed by the gut microbes. In this study, EGP treatment altered the microbiome profile (β diversity) without decreasing the genetic diversity of the communities (α diversity). In contrast, advanced glycation end-products (AGEs), the homologous products of EGPs, were inflammatory and decreased the species richness and α diversity of gut microbiota in Sprague-Dawley rats31 and C57BL/6 mice.32 For CP/CPPS patients, significantly decreased α diversity was observed compared to controls who were asymptomatic or just had urinary tract symptoms.33 However, gut microorganism alteration in clustering but not diversity was reported for T1D patients.34
Sequencing of 16S rRNA-encoding gene has identified Bacteroidetes and Firmicutes as the most abundant phyla in human gut microbiota35 and in fecal samples from NOD mice.36 The F/B ratio has been extensively examined and correlated with various diseases. It has been reported that the F/B ratio was higher in obese subjects and overweight subjects.37 In this study, the F/B ratio was numerically decreased by EGPs, and these NOD mice had fewer prostatic immune infiltrates and higher survival rates. Similar phenomena were observed in our previous genistein studies, in which genistein prevented hyperglycemia and numerically decreased the F/B ratio in NOD mice.36 However, a significantly lower F/B ratio was also observed in patients with systemic lupus erythematosus,38 and these patients have higher IL-10 levels.39 In contrast, it is also reported that aging-dependent decline of IL-10-producing B cells coincides with production of antinuclear antibodies.40 Therefore, it is important to determine if EGP consumption has any adverse effects in lupus patients.
EGP treatment increased Erysipelotrichia at the class level, Erysipelotrichales at the order level and Erysipelotrichaceae at the family level. The presence of a core microbial ecology including Erysipelotrichaceae is essential for a successful therapeutic fecal microbiota transplantation, which controls intestinal inflammation through inducing IL-10 secretion by immune cells.41Porphyromonadaceae at the family level and Parabacteroides at the genus level, which were also upregulated by EGPs, were positively correlated with IL-10 in healthy human stools42 and in mice,43 respectively. In addition, EGPs significantly upregulated Allobaculum, Bacteroides, and Prevotella and downregulated Roseburia at the genus level. Other significantly regulated genera included increased Anaerostipes and decreased Adlercreutzia. The SCFA-producing bacteria Anaerostipes can improve insulin sensitivity by generating butyrate.44 On the other hand, the genus Adlercreutzia was elevated in old vs. young wild type mice.45 Overall, EGP-treated NOD males exhibited a healthier gut microbiome than the NR controls.
Among these significantly regulated genera, Bacteroides, which was predominantly contributed by Bacteroides acidifaciens (Fig. 7B), correlated with all the immune parameters except for the eosinophils (Fig. 7A). The expansion of gut Bacteroides acidifaciens was identified in mice with Atg7 conditional knockout in dendritic cells, which showed a lean phenotype with improved insulin resistance, lower body weight and fat mass.46 In our previous study, decreased Prevotella and Anaerostipes were detected in hyperglycemic male CD-1 mice (multiple low dose streptozotocin-induced) following chronic 2,3,7,8-tetrachlorodibenzo-p-dioxin (TCDD) exposure and correlated with the liver weight, one of the indexes for liver toxicity.16 In CP/CPPS patients, underrepresented Prevotella was detected compared to controls.33 In this study, EGP-mediated increases in Prevotella and Anaerostipes correlated with the changes in eosinophils and IL-10 positively and splenocytes negatively (Fig. 7A). It should be noted that eosinophils can contribute to the inhibition of prostate cancer cell growth47 and resolution of lung-allergic responses following repeated allergen challenge by producing IL-10.48
Conclusions
In summary, this work demonstrated that chronic exposure to glycated whey proteins alleviated autoimmune prostatitis and increased the survival rate in aged male NOD mice, which extended the potential application of EGPs from T1D9 to a broader spectrum of autoimmune diseases. Anti-inflammation and modulated gut microbiome profiles were suggested to be the underlying mechanisms by which EGPs produced their protective effects. To our knowledge, this is the first animal study exploring the effects of EGPs on the gut microbiome. Direct evidence has been presented that EGPs could modulate gut microbes in mice, and the data suggest that microbiome alterations correlate with immunity changes. Admittedly, the cause–effect relationship between the immune system and the gut microbiome following EGP exposure has not been heavily focused on within this work. Future approaches, including fecal transfer and the application of immunodeficient mice models, should be carried out to address the knowledge gap.
Author contributions
Y.C. and T.L.G. designed the study. Y.C. performed the animal experiments and analyzed the data; T.N. performed the histological assessments; T.L.G. conducted flow cytometry; K.M.G. and T.L.G. performed the microbiome analysis. Y.C. and T.L.G. wrote the manuscript.
Conflicts of interest
There are no conflicts to declare.
Acknowledgements
The authors thank Fonterra (USA) Inc (Rosemont, IL) for supplying WPI samples. The authors would like to thank CVM Cytometry Core Facility (the College of Veterinary Medicine, UGA) for assisting with flow cytometric analysis. This study was supported by NIH R41DK121553 (TL Guo) and in part by NIH R21ES024487 (TL Guo), NIH R41AT009523 (TL Guo) and Interdisciplinary Toxicology Program at the UGA.
Notes and references
- J. N. Krieger, L. Nyberg Jr. and J. C. Nickel, J. Am. Med. Assoc., 1999, 282, 236–237 CrossRef CAS PubMed.
- M. A. Pontari and M. R. Ruggieri, J. Urol., 2004, 172, 839–845 CrossRef PubMed.
- A. Gorski, E. Jonczyk-Matysiak, M. Lusiak-Szelachowska, R. Miedzybrodzki, B. Weber-Dabrowska, J. Borysowski, S. Letkiewicz, N. Baginska and K. S. Sfanos, Front. Microbiol., 2018, 9, 1434 CrossRef PubMed.
- W. W. Hochreiter, Eur. Urol., Suppl., 2003, 2, 30–33 CrossRef CAS.
- J. C. Nickel, Can. Urol. Assoc. J., 2011, 5, 306–315 CrossRef.
- Y. Chen, X. X. Chen, T. L. Guo and P. Zhou, Food Res. Int., 2015, 69, 106–113 CrossRef CAS.
- Y. Chen, N. M. Filipov and T. L. Guo, Mol. Nutr. Food Res., 2018, 62, 1700641 CrossRef PubMed.
- Y. Chen and T. L. Guo, Mol. Nutr. Food Res., 2019, 63, e1800885 Search PubMed.
- Y. Chen, T. Nagy and T. L. Guo, J. Funct. Foods, 2019, 56, 171–181 CrossRef CAS.
- E. H. Leiter, ILAR J., 1993, 35, 4–14 CrossRef.
- G. Penna, S. Amuchastegui, C. Cossetti, F. Aquilano, R. Mariani, N. Giarratana, E. De Carli, B. Fibbi and L. Adorini, J. Immunol., 2007, 179, 1559–1567 CrossRef CAS PubMed.
- C. M. Jackson, D. B. Flies, C. A. Mosse, A. Parwani, E. L. Hipkiss and C. G. Drake, Prostate, 2013, 73, 651–656 CrossRef CAS PubMed.
- J. M. Haverkamp, B. Charbonneau, S. A. Crist, D. K. Meyerholz, M. B. Cohen, P. W. Snyder, R. U. Svensson, M. D. Henry, H. H. Wang and T. L. Ratliff, Prostate, 2011, 71, 1139–1150 CrossRef CAS.
- D. S. Oliveira, S. Dzinic, A. I. Bonfil, A. D. Saliganan, S. Sheng and R. D. Bonfil, Bosnian J. Basic Med. Sci., 2016, 16, 8–13 CAS.
- T. C. Glenn, R. A. Nilsen, T. J. Kieran, J. W. Finger, T. W. Pierson, K. E. Bentley, S. L. Hoffberg, S. Louha, F. J. García-De León, M. A. del Río Portilla, K. D. Reed, J. L. Anderson, J. K. Meece, S. E. Aggrey, R. Rekaya, M. Alabady, M. Bélanger, K. Winker and B. C. Faircloth, bioRxiv, 2016, 049114 Search PubMed.
- D. E. Lefever, J. Xu, Y. Chen, G. Huang, N. Tamas and T. L. Guo, Toxicol. Appl. Pharmacol., 2016, 304, 48–58 CrossRef CAS PubMed.
- H. C. Arora, C. Eng and D. A. Shoskes, Ann. Transl. Med., 2017, 5, 30 CrossRef PubMed.
- C. A. Lozupone and R. Knight, FEMS Microbiol. Rev., 2008, 32, 557–578 CrossRef CAS PubMed.
- S. Dagdeviren, D. Y. Jung, R. H. Friedline, H. L. Noh, J. H. Kim, P. R. Patel, N. Tsitsilianos, K. Inashima, D. A. Tran, X. Hu, M. M. Loubato, S. M. Craige, J. Y. Kwon, K. W. Lee and J. K. Kim, FASEB J., 2017, 31, 701–710 CrossRef CAS PubMed.
- D. A. Kinzenbaw, Y. Chu, R. A. Peña Silva, S. P. Didion and F. M. Faraci, Physiol. Rep., 2013, 1, e00149 CrossRef PubMed.
- W. K. E. Ip, N. Hoshi, D. S. Shouval, S. Snapper and R. Medzhitov, Science, 2017, 356, 513–519 CrossRef CAS PubMed.
- M. J. Noto, K. W. Becker, K. L. Boyd, A. M. Schmidt and E. P. Skaar, Infect. Immun., 2017, 85, e00954-16 CrossRef PubMed.
- E. V. Vykhovanets, M. I. Resnick, G. T. MacLennan and S. Gupta, Prostate Cancer Prostatic Dis., 2007, 10, 15–29 CrossRef CAS PubMed.
- A. Calcinotto, A. Brevi, M. Chesi, R. Ferrarese, L. Garcia Perez, M. Grioni, S. Kumar, V. M. Garbitt, M. E. Sharik, K. J. Henderson, G. Tonon, M. Tomura, Y. Miwa, E. Esplugues, R. A. Flavell, S. Huber, F. Canducci, V. S. Rajkumar, P. L. Bergsagel and M. Bellone, Nat. Commun., 2018, 9, 4832 CrossRef PubMed.
- P. Gauffin Cano, A. Santacruz, A. Moya and Y. Sanz, PLoS One, 2012, 7, e41079 CrossRef PubMed.
- L. J. Miller, K. A. Fischer, S. J. Goralnick, M. Litt, J. A. Burleson, P. Albertsen and D. L. Kreutzer, J. Urol., 2002, 167, 753–756 CrossRef CAS PubMed.
- G. Penna, N. Mondaini, S. Amuchastegui, S. Degli Innocenti, M. Carini, G. Giubilei, B. Fibbi, E. Colli, M. Maggi and L. Adorini, Eur. Urol., 2007, 51, 524–533 CrossRef CAS PubMed ; discussion 533.
- C. Hu, H. Yang, Y. Zhao, X. Chen, Y. Dong, L. Li, Y. Dong, J. Cui, T. Zhu, P. Zheng, C. S. Lin and J. Dai, Sci. Rep., 2016, 6, 28608 CrossRef PubMed.
- M. Corzo-Martinez, M. Avila, F. J. Moreno, T. Requena and M. Villamiel, Int. J. Food Microbiol., 2012, 153, 420–427 CrossRef CAS.
- H. F. Erbersdobler, M. Lohmann and K. Buhl, Adv. Exp. Med. Biol., 1991, 289, 363–370 CrossRef CAS PubMed.
- W. Qu, X. Yuan, J. Zhao, Y. Zhang, J. Hu, J. Wang and J. Li, Mol. Nutr. Food Res., 2017, 61, 1700118 CrossRef.
- W. Qu, C. Nie, J. Zhao, X. Ou, Y. Zhang, S. Yang, X. Bai, Y. Wang, J. Wang and J. Li, J. Agric. Food Chem., 2018, 66, 8864–8875 CrossRef CAS PubMed.
- D. A. Shoskes, H. Wang, A. S. Polackwich, B. Tucky, J. Altemus and C. Eng, J. Urol., 2016, 196, 435–441 CrossRef PubMed.
- M. E. Mejia-Leon, J. F. Petrosino, N. J. Ajami, M. G. Dominguez-Bello and A. M. de la Barca, Sci. Rep., 2014, 4, 3814 CrossRef CAS PubMed.
- J. M. Blander, R. S. Longman, I. D. Iliev, G. F. Sonnenberg and D. Artis, Nat. Immunol., 2017, 18, 851–860 CrossRef CAS.
- G. Huang, J. Xu, D. E. Lefever, T. C. Glenn, T. Nagy and T. L. Guo, Toxicol. Appl. Pharmacol., 2017, 332, 138–148 CrossRef CAS PubMed.
- O. Castaner, A. Goday, Y. M. Park, S. H. Lee, F. Magkos, S. T. E. Shiow and H. Schroder, Int. J. Endocrinol., 2018, 2018, 4095789 Search PubMed.
- A. Hevia, C. Milani, P. Lopez, A. Cuervo, S. Arboleya, S. Duranti, F. Turroni, S. Gonzalez, A. Suarez, M. Gueimonde, M. Ventura, B. Sanchez and A. Margolles, mBio, 2014, 5, e01548–e01514 CrossRef CAS PubMed.
- J. Godsell, I. Rudloff, R. Kandane-Rathnayake, A. Hoi, M. F. Nold, E. F. Morand and J. Harris, Sci. Rep., 2016, 6, 34604 CrossRef CAS PubMed.
- K. S. M. van der Geest, P. G. Lorencetti, W. H. Abdulahad, G. Horst, M. Huitema, C. Roozendaal, B. J. Kroesen, E. Brouwer and A. M. H. Boots, Exp. Gerontol., 2016, 75, 24–29 CrossRef CAS PubMed.
- C. Burrello, F. Garavaglia, F. M. Cribiu, G. Ercoli, G. Lopez, J. Troisi, A. Colucci, S. Guglietta, S. Carloni, S. Guglielmetti, V. Taverniti, G. Nizzoli, S. Bosari, F. Caprioli, M. Rescigno and F. Facciotti, Nat. Commun., 2018, 9, 5184 CrossRef PubMed.
- J. S. Bajaj, N. S. Betrapally, P. B. Hylemon, D. M. Heuman, K. Daita, M. B. White, A. Unser, L. R. Thacker, A. J. Sanyal, D. J. Kang, M. Sikaroodi and P. M. Gillevet, Hepatology, 2015, 62, 1260–1271 CrossRef CAS.
- E. Cekanaviciute, B. B. Yoo, T. F. Runia, J. W. Debelius, S. Singh, C. A. Nelson, R. Kanner, Y. Bencosme, Y. K. Lee, S. L. Hauser, E. Crabtree-Hartman, I. K. Sand, M. Gacias, Y. Zhu, P. Casaccia, B. A. C. Cree, R. Knight, S. K. Mazmanian and S. E. Baranzini, Proc. Natl. Acad. Sci. U. S. A., 2017, 114, 10713–10718 CrossRef CAS PubMed.
- S. Khan and G. Jena, Chem.-Biol. Interact., 2016, 254, 124–134 CrossRef CAS.
- N. Thevaranjan, A. Puchta, C. Schulz, A. Naidoo, J. C. Szamosi, C. P. Verschoor, D. Loukov, L. P. Schenck, J. Jury, K. P. Foley, J. D. Schertzer, M. J. Larché, D. J. Davidson, E. F. Verdú, M. G. Surette and D. M. E. Bowdish, Cell Host Microbe, 2017, 21, 455–466 CrossRef CAS.
- J. Y. Yang, Y. S. Lee, Y. Kim, S. H. Lee, S. Ryu, S. Fukuda, K. Hase, C. S. Yang, H. S. Lim, M. S. Kim, H. M. Kim, S. H. Ahn, B. E. Kwon, H. J. Ko and M. N. Kweon, Mucosal Immunol., 2017, 10, 104–116 CrossRef CAS PubMed.
- P. Furbert-Harris, D. Parish-Gause, I. Laniyan, K. A. Hunter, J. Okomo-Awich, T. R. Vaughn, K. C. Forrest, C. Howland, A. Abdelnaby and O. A. Oredipe, Prostate, 2003, 57, 165–175 CrossRef CAS PubMed.
- K. Takeda, Y. Shiraishi, S. Ashino, J. Han, Y. Jia, M. Wang, N. A. Lee, J. J. Lee and E. W. Gelfand, J. Allergy Clin. Immunol., 2015, 135, 451–460 CrossRef CAS PubMed.
Footnote |
† Electronic supplementary information (ESI) available. See DOI: 10.1039/c9fo01740b |
|
This journal is © The Royal Society of Chemistry 2020 |