Silica nanoparticles inhibit arsenic uptake into rice suspension cells via improving pectin synthesis and the mechanical force of the cell wall†
Received
12th September 2019
, Accepted 3rd November 2019
First published on 7th November 2019
Abstract
Our previous studies indicated that the foliar application of silica nanoparticles (SiO2 NPs) could obviously reduce arsenic (As) accumulation in rice. However, the mechanism underlying this effect at the single-cell level has not been reported. In this study, we investigated for the first time the effects of SiO2 NPs on inhibiting As uptake into rice using individual rice cells. The results indicated that the addition of SiO2 NPs could enhance the proportion of live cells by weakening oxidative stress upon As exposure. Compared to the treatment of cells with As only, treatment with SiO2 NPs could maintain the integrity of the cell, increase the thickness of the cell wall (77.4%) and the ratio of As in the pectin (19.6%). In addition, the pectin content, cation exchange capacity (CEC) and pectin methylesterase (PME) activity were also increased in the SiO2 NPs-pretreated cells, leading to a decreased degree of pectin methylesterification and an improved mechanical force of the cell walls. Furthermore, in the SiO2 NPs-pretreated rice cells, the expression of the OsLis1 and OsLis2 genes was lower, whereas the expression of the OsNIP1;1 and OsNIP3;3 genes was higher than that of the As-only group. This finding provides new insights into the mechanism of how the addition of SiO2 NPs inhibits As uptake into rice at the single-cell level and lays the foundation for its application in As-contaminated paddy soil.
Environmental significance
Recently, soil pollution from heavy metal ions has been a worldwide concern. Therein, trivalent arsenic (As(III)), one of the most toxic heavy metal elements, has caused many environmental problems because of its high toxicity and carcinogenicity to human beings. In this study, we explored the effects of SiO2 NPs on inhibiting As uptake into rice using individual rice cells. A mechanism was proposed that SiO2 NPs could reduce obviously the As accumulation in rice at the single-cell level. This finding provides new insights into the understanding of the SiO2 NPs inhibition of the uptake of As in rice and lays the foundation for its application in As-contaminated paddy soil.
|
1. Introduction
Arsenic (As) is a toxic element that is a non-threshold carcinogen and is distributed widely in the environment.1 It has been reported that the pollution of paddy soil with As by industrial activities, irrigation with As-polluted groundwater, and the use of arsenic-based fertilizer and pesticide resulted in a further increase in As concentrations in rice.2,3 Rice is a staple food crop worldwide, especially in Southeast Asian countries.4 When rice is cultivated in As-contaminated paddy soil, As can be easily taken up by rice, leading to the inhibition of plant growth, nutrient imbalances and the reduction of yield.5 The accumulation of As in human bodies through the food chain may pose detrimental effects to human health, including denaturation of proteins and enzymes,6 various types of cancer and impairment of the immune response.7 Thus, methods to reduce As accumulation in rice grains is an important public concern.
As the second most abundant element in the Earth's crust, silicon (Si) is beneficial for the development of rice. It was reported that the application of Si fertilizer can promote structural stability, improve the quality of grain and yield, and enhance the resistance of rice under As stress.8–10 For example, Wu et al. suggested that exogenous Si could decrease the shoot inorganic As concentrations in ‘XFY-9’ and ‘XWX-12’ rice by 31% and 25%, respectively.11 Tripathi et al. demonstrated that the addition of Si reduced As accumulation by elevating the antioxidant defence system in rice.12 A study from our research group also found that the addition of bioavailable Si contributed largely to As accumulation in rice grains and played a crucial role in mitigating As stress in rice by random forest analysis.13 One reason for this effect is that silicon acid shares the same transporter with arsenite.14 Thus, exogenous Si could regulate the expression of the Si transporter and thus decrease As accumulation in rice. The application of Si fertilizer is regarded as an economical and efficient method to reduce As uptake and accumulation in rice.15,16
In recent years, the application of nanomaterials in the fields of agriculture and environmental protection has attracted more attention due to the unique chemical and physical properties of the materials. Traditional Si fertilizers are barely utilized directly by plants due to their low solubility and bioavailability.17 Compared with conventional Si fertilizer, silica nanoparticles (SiO2 NPs) with high bioavailability can easily penetrate the leaf and are absorbed rapidly onto the leaf surface. Suriyaprabha et al. indicated that SiO2 NPs promote seed coat resistance and improve the silica availability to maize plants compared with other bulk Si sources.18,19 It is well known that rice is a silica-accumulating plant that takes up amorphous silica, depositing it onto the cell walls and preventing the uptake of heavy metals into the cell.20,21 Zhang et al. proposed that silica deposition in rice could play important roles in defending against abiotic stress, such as heavy metal toxicity.22 Tripathi et al. found that the addition of SiO2 NPs could be more effectively decreased accumulation of arsenate and oxidative stress in the maize cultivar than the bulk of Si under arsenate stress, which is due to its greater availability to seedlings.23 Our previous study also demonstrated that the foliar application of SiO2 NPs in As-contaminated paddy soil increased the dry weight of rice, whereas it obviously reduced the As accumulation in the grain and shoot.24 Therefore, the application of SiO2 NPs is an important measure for obtaining high yield and reducing As accumulation in rice grains.
Previous studies suggested that Si could effectively reduce the uptake of As at the whole-plant level, and the underlying mechanisms at the single-cell level have yet to be investigated. Recently, He et al. demonstrated that hemicellulose could be the major ligand bound to Si by the formation of [Si-hemicellulose matrix] at the single-cell level.25 Furthermore, Maria et al. found that silicate effectively decreased Cd uptake by regulating Cd and phytochelatin-related genes at the cellular level.26 Our previous studies have also indicated that most SiO2 NPs accumulate in the rice suspension cell walls.27 In the present study, our aim was to investigate how SiO2 NPs inhibit the cellular uptake and transport of As using individual rice cells. This was conducted by investigating the effect of SiO2 NPs on As uptake into rice cells using a series of molecular biological and physical techniques, including inductively coupled plasma mass spectrometry (ICP-MS), energy-dispersive X-ray spectroscopy (EDX), atomic force microscopy (AFM) and polymerase chain reaction (PCR). We demonstrate that the addition of SiO2 NPs significantly increases the pectin content and improves the mechanical force of the cell wall, leading to decreased As uptake into rice cells. Moreover, the supply of SiO2 NPs regulated the expression of the genes encoding the As-related transporters.
2. Materials and methods
2.1 Materials
NaAsO2 was purchased from Anpel Laboratory Technologies Company (purity > 99%, Shanghai, China). Other chemicals were provided by Guangzhou Chemical Reagent Company (Guangzhou, China). All the chemicals were of analytical grade without any further purification. Milli-Q water-supplying device Milli-Q water was used in all experiments. The ultrapure water (18 MΩ.cm) was from the purification device (Milli-Q, Billerica, MA, USA). The detailed methods for the synthesis and characterization of SiO2 NPs are described in the ESI† (S1.1 and S2.1 and Fig. S1).
2.2 Cell viability assay
Rice suspension cell lines (Oryza sativa L. japonica) were cultivated following previously reported methods.28,29 Briefly, rice seeds were washed three times with ultrapure water and sterilized using ethanol (75%) and mercuric chloride (0.1%) for 10 min. After sterilization, the seeds were incubated at 28 °C for 1 month in the dark to obtain callus. Subsequently, the calli were transferred into a glass flask containing modified N6 medium (Table S1†) and further cultured for 48 h at 28 °C in the dark. The detailed methods for the cell viability, reactive oxygen species (ROS) and mitochondrial transmembrane potential (MMP) assays are described in the ESI† (S1.2 and S1.3).
2.3 Cell morphology
The rice cells were harvested by centrifugation (1700×g) and thoroughly washed with buffer to remove the cell medium. Subsequently, the samples were pre-fixed in 2.5% glutaraldehyde for 5 h, thoroughly rinsed with 0.1 M phosphate buffer solution (PBS, pH = 7.2) and dehydrated in an ascending ethanol series (30, 50, 70, 90, 95 and 100% v/v). Then, the dehydrated cells were embedded in spur resin and cut into ultrathin sections using a microtome (Leica, Germany). Finally, the obtained samples were observed using Transmission Electron Microscope (TEM) equipped with an Oxford INCA Energy TEM 200 EDX system (TECNAI 10, Philips, Netherlands).30 The thickness of the cell wall was analysed using ImageJ software.
2.4 Analysis of As and Si contents
The concentration of As was measured using the method in our previous study.24 Lyophilized samples (0.05 g) were weighed into Teflon bottles, and 5 mL of HNO3-H2O2 mixture was added. After overnight incubation at room temperature, the digestion solution was heated using a graphite furnace at 120 °C until the extracts became clear. The digested extracts were diluted to a volume of 25 mL with ultrapure water and then measured for total As concentration with a hydrogen generation-atomic fluorescence spectrometer (AFS-820, Beijing Titan Instruments Co., Beijing, China). The content of Si in the rice cells was measured following the reported literature.13 The obtained sample was transferred to the Teflon bottle, and then 10 mL HCl (1 M), 10 mL deionized H2O and 2 mL NH4F (1 M) were added. Subsequently, 10 mL ethyl alcohol and 15 mL ammonium molybdate (5%) was added and stirred 20 min. Finally, 10 mL of tartaric acid–oxalic acid (0.5–0.5 M) and 5 mL of ascorbic acid (2%) were added into the solution. The concentration of Si was measured by PerkinElmer spectrophotometer (Waltham, MA, USA).
2.5 Cation exchange capacity and surface potential
The cell wall was extracted as reported in the literature.31 The detailed method is described in the ESI† (S1.4). Cation exchange capacity (CEC) was determined by incubating cell wall material in 10 mL of BaCl2 (50 mM, pH = 5.0) for 30 min with stirring at 4 °C. After centrifugation at 2000 rpm, the pellet was resuspended in fresh BaCl2 solution, washed three times with ultrapure water, dried and digested in 5 mL of HNO3-H2O2 mixture. Finally, CEC were measured by inductively coupled plasma optical emission spectrometry (ICP-OES).31 The surface potential of the sample was measured using a Malvern Zetasizer Nano ZS (Worcestershire, UK).
2.6 Determination of PME activity and degree of pectin methylesterification
PME activity was measured using the previously reported method.32 Specifically, alcohol oxidase was added to the PBS solution (pH = 7.5). Then, 100 μL of pectin supernatant was added into the tube to obtain the mixture and further incubated for 30 min in a water bath at 30 °C. Then, 200 μL of Purpald was added into the mixture and continuously shaking was applied. After shaking for 30 min, the mixture was transferred into a 2 mL plastic tube containing 0.5 mL ultrapure water. The absorbance values of the obtained samples were measured at 550 nm. The pectin content was analysed as described in the ESI† (S1.5).
2.7 Atomic force microscope (AFM) imaging and measurement of mechanical properties
The Lyophilized cell wall was treated with 0.02% Poly-L-Lysine for 10 min and then suspended in ultrapure water for 1 h. Subsequently, the obtained sample was washed with ultra-high purity water, dripped onto completely clean coverslips and then allowed to air dry overnight. The coverslip was glued to a glass slide and placed on a piezoelectric scanning tube of a MultiMode VIII AFM (Bruker, Santa Barbara, CA, USA). AFM imaging was performed with a Bruker ScanAsyst-Air probe (tip radius = 10 nm, spring constant = 5 N m−1) (Bruker, Santa Barbara, CA, USA) and a silicon nitride cantilever (Fig. 6A). Before each measurement, the spring constant of the probe was calibrated. All images were obtained at a low scan rate (1 Hz). Measurement of the mechanical properties of the cell wall was performed after each imaging. The Poisson's ratio of the sample was assumed to be 0.3. The force–distance curve was continuously recorded by tapping the sample surface in a scan range of 2 × 2 μm in a grid of 16 × 16 points.34 Young's modulus was calculated using Nanoscope analysis software based on the Hertz-Sneddon model:
where E is the Young's modulus, F represents force, ν represents the Poisson ratio, R represents the tip radius, and δ represents the maximum deformation. For each sample, 3 randomly chosen fragments were detected in air at 50–60% relative humidity.
2.8 Gene expression analysis
Rice suspension cells were cultured with or without SiO2 NPs for 24 h. Different concentrations of As (0, 10, 20, or 40 μM) were added into the culture solutions 24 h before the RNA extraction. Total RNA was isolated using a Plant RNA Kit (Omega Bio-Tek, Norcross, USA) according to the manufacturer's instructions. Complementary DNA (cDNA) was prepared using a reagent kit (Takara, Kyoto, Japan). Quantitative real-time polymerase chain reaction (qRT-PCR) analysis was conducted using SYBR Premix Ex TaqTM II (Takara, Kyoto, Japan). The gene-specific primers used for qRT-PCR are described in Table S2.† The actin gene was used as an internal control. The data were calculated using the 2−ΔΔCt method.33 All experiments were conducted with three independent biological replicates.
2.9 Statistical analysis
The data were analysed by analysis of variance (ANOVA). Tukey's test (P < 0.05) was then conducted if the ANOVA indicated significance.
3. Results
3.1 Effects of SiO2 NPs pretreatment on alleviating As toxicity and oxidative stress
We first investigated the effect of SiO2 NPs on As toxicity using individual rice suspension cells. Cell viability was assessed using double staining with fluorescein diacetate/propidium iodide (FDA/PI) to distinguish live/dead cells. As shown in Fig. 1A, the amount of red rice cells increased obviously with increasing As concentration, suggesting that the cells treated with As only had low viability. Interestingly, after pretreatment with 0.1 mm SiO2 NPs for 24 h, the red cells became green (Fig. 1B). When the concentration of SiO2 NPs reached up to 1.0 mM, we found that almost all of the rice cells were green (Fig. 1C). Thus, our results indicated that pretreatment with SiO2 NPs can alleviate As toxicity in rice suspension cells. To quantify cell viability, the rice cell survival percentage was detected using flow Cytometry. The results showed that the survival percentage of the As-only treated cells decreased obviously with increasing As concentration. However, compared with treatment with As alone, the survival percentage of SiO2 NPs-pretreated cells at concentrations of 10, 20 and 40 μM As increased by 92.5%, 85.4% and 83.6%, respectively (Fig. 2A). To correlate the effect of SiO2 NPs on As toxicity, we further measured the level of ROS. The results indicated that the fluorescence intensity of SiO2 NPs-pretreated cells at concentrations of 0, 10, 20 and 40 μM As decreased by 4.5-, 6.2- and 4.8-fold, respectively, compared with treatment with As alone (Fig. 2B). Furthermore, we found that the level of MMP in the SiO2 NPs-pretreated cells was decreased drastically in comparison to that in the As-only treated cells (Fig. 2C). These results suggested that the addition of SiO2 NPs could enhance As detoxification and significantly decrease oxidative stress upon As exposure.
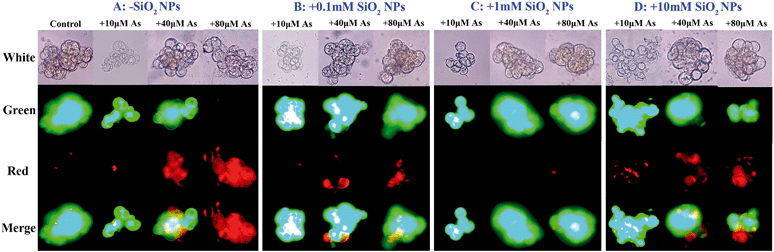 |
| Fig. 1 Optical (upper panels) and fluorescence (lower panels) images of suspension cells cultivated under the treatments without (A)/with (B and C) SiO2 NPs (0.1 and 1 mM) or As treatment (10, 40 and 80 μM). The colour green represents a viable cell; the colour red represents a dead cell. | |
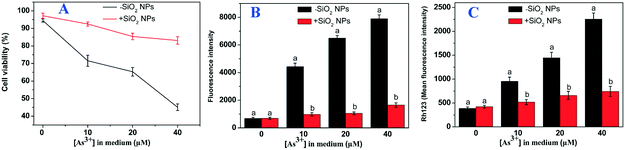 |
| Fig. 2 (A) The cell viabilities of suspension-cultured cells exposed to various As concentrations (10, 20 and 40 μM) measured in the absence and presence of SiO2 NPs; ROS (B) and MMP (C) quantitative analysis of the mean fluorescence intensity of cells after treatment. The values are the means ± SD of three independent sets of experiments. The statistical comparison was performed using one-way ANOVA followed by Tukey's test (P < 0.05). | |
3.2 Effect of SiO2 NPs pretreatment on the morphology and structure of rice cells
To investigate the effect of SiO2 NPs on alleviating the toxicity of As, TEM was used to investigate SiO2 NPs localization within the rice cells. From Fig. 3A, we can observe that the cell membrane/wall of cells incubated without As and with SiO2 NPs was intact. These cells are divided normally, forming a cluster of cells with an appropriate size range. After exposure to As for 24 h, the integrity of the rice cell was completely destroyed following the collapse of the cell structure (Fig. 3B). In contrast, in the presence of the SiO2 NPs, the cells exposed to As remained essentially intact with a normal shape (Fig. 3C). Interestingly, the SiO2 NPs-pretreated cell walls were obviously thickened compared with the As-only treated cell walls. The thicknesses of the cell walls with and without SiO2 NPs pretreatment were 168 ± 42 nm and 298 ± 54 nm under As stress, respectively (Fig. 3D and F). This result indicated that the SiO2 NPs treatment could significantly increase the thickness of the cell wall. Moreover, the chemical composition of the cell wall was determined using EDX. As shown in Fig. 3E, there were three main peaks in the EDX spectrum, which correspond to carbon, oxygen and arsenic elements, respectively. This confirms the existence of As on the cell wall. The result of EDX analysis provided direct evidence for arsenic sorption onto the cell wall with pretreatment of SiO2 NPs.
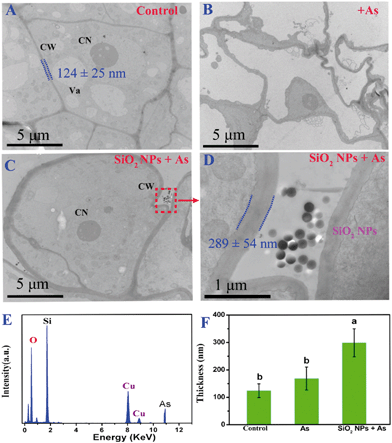 |
| Fig. 3 TEM images of the microstructures of the rice cell suspensions cultured under various conditions: (A) control, (B) + 40 μM As, (C and D) SiO2 NPs + 40 μM As, (E) energy dispersive X-ray (EDX) spectra taken from the dotted rectangle in Fig. 3C, suggesting the presence of Si and As at the cell wall surface. CW, cell wall; CN, cell nucleus; Va, vacuole; (F) the thickness of cell walls under different treatments was measured from the micrographs using ImageJ software analysis software. The data are expressed as the means ± SD. Differences in cell wall thickness under different treatments were analyzed using one-way ANOVA followed by Tukey's test (P < 0.05). | |
3.3 Effect of SiO2 NPs pretreatment on the subcellular distribution of As
To clarify the subcellular distribution of Si and As, the cell wall and pectin were isolated. The contents of Si and As were determined using ICP-MS, and the results showed that the Si concentration in the SiO2 NP-pretreated cells was significantly higher than that in the untreated cells (Fig. 4A). To our surprise, the As concentration in the rice cells did not obviously change between SiO2 NPs-pretreated cells and untreated cells (Fig. 4B). Only 27.7% of the As accumulated in the SiO2 NPs-untreated cell walls. Nevertheless, the As content in the SiO2 NP-pretreated cell wall was increased significantly compared with that of the As-only cells. Most of the As content (72.9%) accumulated in the cell walls. Compared with the cells cultured without the addition of SiO2 NPs, the percentage of As in the pectin of SiO2 NPs-pretreated cells was increased by 19.6%. The results indicated that the addition of SiO2 NPs could enhance the ratio of As in the pectin of cell walls.
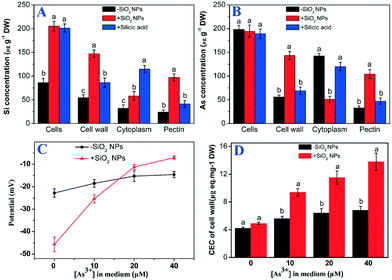 |
| Fig. 4 The content of Si (A) and As (B) in whole cells, cell walls, cytoplasm and pectin in the treatment of control,SiO2 NPs and silicic acid; CEC (C) and surface potential of cell wall (D); statistical comparisons were performed using one-way ANOVA followed by Tukey's test (P < 0.05). | |
3.4 Effect of SiO2 NPs pretreatment on the properties of cell wall
The results showed that the cation exchange capacity of the rice cell wall was improved by pretreatment with SiO2 NPs. Compared with treatment with As alone, the CEC of the SiO2 NPs-pretreated cell walls increased by 1.7-, 1.8- and 2.1-fold at As concentrations of 10, 20 and 40 μM, respectively (Fig. 4C). Similarly, the potential of the SiO2 NPs-pretreated cell walls was significantly higher than that of the As-only group (Fig. 4D). To further understand the role of the cell wall, we extracted pectin from the cell walls and determined the content and properties of the pectin. We found that the surface potential and PME activity of the pectin of SiO2 NPs-pretreated cells was drastically increased compared with that of cells treated with As alone (Fig. 5A and B). However, the degree of pectin methylesterification was decreased significantly in the SiO2 NPs-pretreated cell walls (Fig. 5C). Furthermore, compared with the As-only group, the pectin content in the SiO2 NPs-pretreated cell walls at As concentrations of 0, 10, 20 and 40 μM increased by 2.0-, 2.0-, 2.1-, 1.9-fold, respectively (Fig. 5D). These results indicated that the presence of SiO2 NPs could increase the pectin content and CEC of the cell wall and decrease the degree of pectin methylesterification.
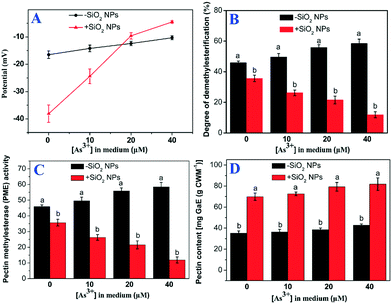 |
| Fig. 5 The surface potential (A), degree of pectin methylesterification (B), PME activity (C) and content (D) of pectin. The values are the means ± SD of three independent sets of experiments. The statistical comparison was performed using one-way ANOVA followed by Tukey's test (P < 0.05). | |
The pectin content may influence the structural and mechanical properties of the cell wall. In this study, AFM was applied to directly measure the surface morphology and Young's modulus of the rice cell wall. The three-dimensional topography clearly indicated that the SiO2 NPs-pretreated and untreated cell walls had large spatial mechanical differences. The surfaces of the cell walls treated with As alone were rough (Fig. 6D). However, the surface of the cell wall became flat with the addition of SiO2 NPs (Fig. 6E–G). Meanwhile, the mechanical properties of cell walls were measured by AFM force curves following imaging, and Young's modulus was calculated according to the Nanoscope Analysis software (Fig. 6C'–G'). The AFM-based elastic modulus mapping indicated that the mechanical strength (Young's modulus) of cell walls was 1.99 ± 0.43 GPa naturally, whereas the Young's modulus of cell walls with sole As treatment was significantly decreased to 0.26 ± 0.02 GPa. Compared with the cells cultured without the addition of SiO2 NPs, the average Young's modulus values of SiO2 NPs-pretreated cell walls under As stress at 0.1, 1 and 10 mM increased by 4.5-, 9.9- and 23.8-fold, respectively (Fig. 6B). This result suggested that the presence of SiO2 NPs significantly improved the mechanical properties of the cell wall compared with treatment solely with As.
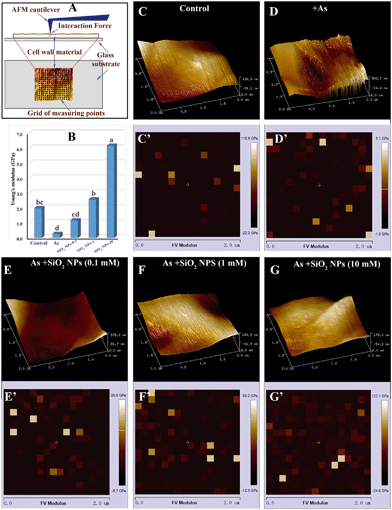 |
| Fig. 6 Schematic illustration to evaluate the mechanical properties of the cell wall using AFM (A); Young's modulus of the cell wall (B); three-dimensional topography of the cell wall pretreated with or without various concentrations of SiO2 NPs (C, D, E, F and G); elasticity topography of the cell wall pretreated with or without various concentrations of SiO2 NPs (C', D', E', F' and G'). | |
3.5 Effect of SiO2 NPs pretreatment on the expression level of As-related genes
To further investigate the influence of SiO2 NPs on the uptake of As, the relative expression levels of As-related genes were measured. Our results showed that OsLsi1 and OsLsi2 showed lower expression levels in the SiO2 NPs-pretreated rice cells than in the untreated cells (Fig. 7A and B). However, the relative expression levels of OsNIP1;1 and OsNIP3;3 in the SiO2 NPs-treated cells were significantly increased with increasing As concentration in the medium. No obvious changes in the expression levels of OsNIP1;1 and OsNIP3;3 genes were measured in cells without SiO2 NPs when the As concentration in the cell culture medium was increased (Fig. 7C and D). This result indicated that the addition of SiO2 NPs could induce the overexpression of OsNIP1;1 and OsNIP3;3 in rice cells and then prevent As from entering the cell.
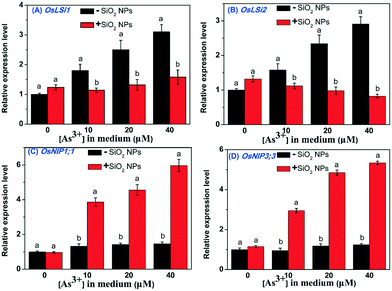 |
| Fig. 7 The relative expression levels of the OsLsi1 (A), OsLsi2 (B), OsNIP1;1 (C) and OsNIP3;3 (D) genes after treatment with As in rice suspension-cultured cells that with or without SiO2 NPs pretreatment for 24 h. the values are the means ± SD of three independent sets of experiments. The statistical comparison was performed using one-way ANOVA followed by Tukey's test (P < 0.05). | |
4. Discussion
4.1 Effect of SiO2 NPs pretreatment on alleviating As toxicity
Recent studies have demonstrated that the addition of SiO2 NPs effectively decreased As concentrations in rice shoots and grains through pot and field experiments.35 Our previous studies also observed similar results.24 Although the fact that SiO2 NPs application could reduce the uptake of As at whole-plant and tissue levels has been investigated, the underlying mechanisms at a single level remain relatively unexplored. In the present study, the results of cell viability assays indicated that the viability of As-only treated rice cells decreased obviously with increasing As concentration. Nevertheless, the addition of SiO2 NPs could effectively enhance the proportion of live rice cells under exposure to As (Fig. 2A). From these results, we concluded that pretreatment with SiO2 NPs can alleviate As toxicity in rice suspension cells. It was reported that the metalloid arsenic could promote the production of ROS, resulting in disturbances to the antioxidant system and damage to the rigidity of the cell wall.36 Consistent with these studies, our results indicated that a high concentration of As could trigger excessive production of ROS and increase relevant oxidative damage. However, SiO2 NPs treatment resulted in significantly lower ROS and MMP levels in the SiO2 NPs-pretreated group than in the As-only group (Fig. 2B and C), indicating that As toxicity is alleviated in the presence of SiO2 NPs. Our results indicated that a high concentration of As could trigger excessive production of ROS and MMP. However, the SiO2 NPs-pretreated cells was significantly lower ROS and MMP levels than that of the As-only group. It is reported that a significant amount of ROS is involved in stress-induced cell death in plant cells. Mitochondria is a major site and origin of generation of ROS responsible for cell death.37 MMP could be typically used as the indicator of the activity of the mitochondrial inner membrane in rice cells. The increase of MMP can disrupt the electron transport chain and cause directly mitochondrial dysfunction.38 The reduction of ROS and MMP in the SiO2 NPs-pretreated rice cells was likely that the presence of Si could scavenge excess ROS and reduce oxidative damage by decreasing the permeability and lipid peroxidation of the plasma membrane. Similarly, the applied Si could scavenge excess ROS and reduce oxidative damage by decreasing the permeability and lipid peroxidation of the plasma membrane.35 Our previous study also suggested that SiO2 NPs play a crucial role in maintaining the integrity of rice cells after Cd exposure.27
4.2 Effect of SiO2 NPs pretreatment on improving the mechanical force of the cell wall
As the first cell organ to contact As, it is suggested that the cell wall would be the first physiological barrier to prevent As from entering into the cell. In light of this, we also observed the morphology of rice cells, finding that the presence of SiO2 NPs could maintain the integrity of the cell, significantly increasing the thickness of the cell wall and further protecting the cells from oxidative damage under As stress (Fig. 3). The plant cell wall is mainly composed of cellulose and polysaccharides, including pectin and hemicellulose. These constituents could influence cell wall characteristics and functions, such as the permeability, extensibility, and porosity.39 The cell wall carries negative charges and is able to bind divalent and trivalent cations due to the presence of polysaccharides. The ICP-MS results further demonstrated that the total content of As in whole SiO2 NPs-pretreated and untreated cells did not obviously change. Only 28.3% of the As accumulated in the SiO2 NPs untreated cell walls. And most of the As content (71.7%) was distributed into the SiO2 NPs untreated cell. It was likely that the As binding sites in the SiO2 NPs untreated cells reached complete saturation, beyond the capability of the cell walls to exert an impact on the As uptake of cells. Therefore, we can reasonably that As molecules could enter into the cells across cell membrane. However, there was a highly significant linear correlation between the As concentrations in the cell wall and the pectin fraction, suggesting that most of the As content (73.6%) was deposited into the cell walls and that pectin is the major contributor to the increased As retention in cell walls when SiO2 NPs are applied. This was consistent with our previous findings that the foliar application of SiO2 NPs could significantly increase the proportion of As bound to the shoot cell wall.24 Similarly, Stanley et al. found that 40% of accumulated As was bound to the cell wall fraction in the leaves of As-treated rice.40 It was reported that pectin has previously been shown to be a cation adsorber.41 After binding with SiO2 NPs, the cell wall and pectin possess a more negative charge in the absence of As. However, with exposure to As, the surface potential of the SiO2 NPs-treated cell wall and pectin was obviously improved compared with that of untreated SiO2 NPs. It is likely that the negatively charged SiO2 NPs-treated cell wall and pectin were close enough to bind with As by the electrostatic adsorption model. Similar results have demonstrated that the negatively charged carboxylic groups on pectin have a high affinity for As3+, Al3+ and Cd2+.42 It was reported that pectin consists of complex polysaccharides, including homogalacturonan, rhamnogalacturonans I and II, galactans, arabinans and other polysaccharides.43
Previous studies found that the density of the negative charges on pectin is determined by its degree of demethylation, which is controlled by the activity of PME enzymes.32 In the present study, the pectin content and PME activity were obviously increased in the presence of SiO2 NPs, further confirming that pectin is the major binding site for As cations. It was reported that pectic polysaccharides crosslinked with silica are key to the physical strength of plant cells.44 To further investigate the structure and properties of SiO2 NPs-pretreated and untreated rice cells under As stress, AFM has also been recently used as a powerful tool for the evaluation of the surface topography and mechanical properties of cell wall materials.45 We found that there was a concomitant increase in the average Young's modulus of the cell wall with increasing SiO2 NPs concentration, further confirming that the supply of SiO2 NPs could improve the mechanical force of the cell wall and thus inhibit As uptake into the cell. Some previous studies also demonstrated that the presence of Si produced a similar effect, improving the mechanical properties of the cell walls by the HC-bound form of Si.25 Moreover, the binding and cross-linking of cations to the pectin matrix could influence the porosity of cell walls.46 Additionally, it has been suggested that the content of pectin in the cell walls could alter cell wall characteristics and functions. Combined with the results of ICP-MS, XPS and AFM, we demonstrate that the addition of SiO2 NPs could stimulate the synthesis of pectin, thicken the cell walls, and thus inhibit As uptake into cell by improving the mechanical force of the cell wall. Therefore, this inhibitory effect involved the physiological function of SiO2 NPs.
4.3 Effect of SiO2 NPs pretreatment on regulating the expression of as-related genes
Apart from the physical and chemical binding of As to the binding sites on the cell walls, the OsLsi1 (Low silicon 1) and OsLsi2 (Low silicon 2) genes encode the uptake of As. OsLsi1 is responsible for the entry of arsenite into rice roots, while OsLsi2 is responsible for arsenite efflux in the xylem.47,48 Both transporters control the accumulation of As in roots and shoots of rice due to the shared transport pathway. The previous study indicated that the Si supply may affect the uptake and translocation of arsenic due to the shared transport pathway by regulating the expression of Si transport genes.49 Our results indicated that the presence of SiO2 NPs could obviously inhibit the expression levels of OsLsi1 and OsLsi2. Because As(III) shares the Si transport pathway, this inhibitory effect may involve a direct competition for the transporter proteins by the addition of Si. However, in our previous study, the addition of SiO2 NPs can stimulate the expression of OsLsi1 in rice cells.27 This opposite result was likely because silicon shares the same transporter with arsenite. As a member of the NIP aquaporin family, the OsNIP1;1 and OsNIP3;3 proteins were localized at the plasma membrane and permeable to arsenite.50 In the SiO2 NPs-pretreated rice cells, the expression levels of OsNIP1;1 and OsNIP3;3 were increased several times more than in SiO2 NPs-untreated cells at different concentrations of As. Similarly, Zhao et al. suggested that the overexpression of OsNIP1;1 and OsNIP3;3 could reduce the accumulation of As in rice shoots and grain.51 The above results showed that the SiO2 NPs supply can reduce the uptake of As by regulating the expression of As-related genes. We reasoned that the cellular transporter response could be attributed to the decreased uptake of As via the improved mechanical force of the cell wall.
Based the above results and discussion, we propose the mechanism for inhibiting As uptake into rice by the supply SiO2 NPs at the single-cell level. The addition of SiO2 NPs could stimulate the synthesis of pectin and then improve the mechanical force of the cell wall. Such an enhanced mechanical force of the cell wall in turn decreases the uptake of As from the medium, thereby giving rise to regulate the expression of As-related genes and decrease the cellular ROS and MMP levels.
5. Conclusion
In this study, the data demonstrated that treatment with SiO2 NPs decreases the cellular ROS and MMP levels and increases the pectin content, CEC and PME activity of the cell wall under As stress, leading to a decreased degree of pectin methylesterification and an improved mechanical force of the cell wall. Additionally, the SiO2 NPs treatment could regulate the expression of As-related genes upon exposure to As. Thus, As was mainly deposited into the cell walls, and pectin was the major contributor to the increased As retention in the cell wall. To the best of our knowledge, this is the first study to reveal the mechanisms by which SiO2 NPs inhibit the uptake of As in rice at the single-cell level. Furthermore, this study will facilitate a better understanding of how SiO2 NPs inhibit the uptake and transport of As and may provide a new strategy for designing effective fertilizers to decrease the accumulation of As in rice.
Conflicts of interest
There are no conflicts to declare.
Acknowledgements
This work was financially supported by National Natural Science Foundation of China (No. 41877137), GDAS' Project of Science and Technology Development (2018GDASCX-0106, 2017GDASXC-0404, 2018GDASXC-0501 and 2017GDASCX-0835), National Key Research and Development Project of China (No. 2016YFD0800700), Science and Technology Planning Project of Guangzhou (201604020039), Natural Science Foundation of Guangdong Province, China (2019B151502044 and 2018A030313745).
References
- A. H. Smith, P. A. Lopipero, M. N. Bates and C. M. Steinmaus, Public health-Arsenic epidemiology and drinking water standards, Science, 2002, 296, 2145–2146 CrossRef CAS.
- Y. G. Zhu, G. X. Sun, M. Lei, M. Teng, Y. X. Liu, N. C. Chen, L. H. Wang, A. M. Carey, C. Deacon, A. Raab, A. A. Meharg and P. N. Williams, High percentage inorganic arsenic content of mining impacted and nonimpacted Chinese rice, Environ. Sci. Technol., 2008, 42, 5008–5013 CrossRef CAS.
- F. J. Zhao, J. F. Ma, A. A. Meharg and S. P. McGrath, Arsenic uptake and metabolism in plants, New Phytol., 2009, 181, 777–794 CrossRef CAS PubMed.
- A. A. Meharg, P. N. Williams, E. Adomako, Y. Y. Lawgali, C. Deacon, A. Villada, R. C. J. Cambell, G. Sun, Y. G. Zhu, J. Feldmann, A. Raab, F. J. Zhao, R. Islam, S. Hossain and J. Yanai, Geographical variation in total and inorganic arsenic content of polished (White) rice, Environ. Sci. Technol., 2009, 43, 1612–1617 CrossRef CAS.
- M. A. Rahman, H. Hasegawa, M. M. Rahman, M. A. M. Miah and A. Tasmin, Straighthead disease of rice (Oryza sativa L.) induced by arsenic toxicity, Environ. Exp. Bot., 2008, 62, 54–59 CrossRef CAS.
- S. W. Shen, X. F. Li, W. R. Cullen, M. Weinfeld and X. C. Le, Arsenic binding to proteins, Chem. Rev., 2013, 113, 7769–7792 CrossRef CAS.
- E. H. Kim, N. J. Yoon, S. U. Kim, T. K. Kwon, S. Sohn and K. S. Choi, Arsenic trioxide sensitizes human glioma cells, but not normal astrocytes, to TRAIL-induced apoptosis via CCAAT/enhancer-binding protein homologous protein-dependent DR5 up-regulation, Cancer Res., 2008, 68, 266–275 CrossRef CAS.
- C. Meharg and A. A. Meharg, Silicon, the silver bullet for mitigating biotic and abiotic stress, and improving grain quality in rice, Environ. Exp. Bot., 2015, 120, 8–17 CrossRef CAS.
- E. Epstein, Silicon: its manifold roles in plants, Ann. Appl. Biol., 2009, 155, 155–160 CrossRef CAS.
- R. J. Haynes, Significance and Role of Si in Crop Production, Adv. Agron., 2017, 146, 83–166 Search PubMed.
- C. Wu, Q. Zou, S. G. Xue, J. Y. Mo, W. S. Pan, L. Q. Lou and M. H. Wong, Effects of silicon (Si) on arsenic (As) accumulation and speciation in rice (Oryza sativa L.) genotypes with different radial oxygen loss (ROL), Chemosphere, 2015, 138, 447–453 CrossRef CAS PubMed.
- P. Tripathi, R. D. Tripathi, R. P. Singh, S. Dwivedi, D. Goutam, M. Shri, P. K. Trivedi and D. Chakrabarty, Silicon mediates arsenic tolerance in rice (Oryza sativa L.) through lowering of arsenic uptake and improved antioxidant defence system, Ecol. Eng., 2013, 52, 96–103 CrossRef.
- H. Y. Yu, X. D. Ding, F. B. Li, X. Q. Wang, S. R. Zhang, J. C. Yi, C. P. Liu, X. H. Xu and Q. Wang, The availabilities of arsenic and cadmium in rice paddy fields from a mining area: The role of soil extractable and plant silicon, Environ. Pollut., 2016, 215, 258–265 CrossRef CAS PubMed.
- J. F. Ma, N. Yamaji, N. Mitani, X. Y. Xu, Y. H. Su, S. P. McGrath and F. J. Zhao, Transporters of arsenite in rice and their role in arsenic accumulation in rice grain, Proc. Natl. Acad. Sci. U. S. A., 2008, 105, 9931–9935 CrossRef CAS.
- N. K. Savant, G. H. Snyder and L. E. Datnoff, Silicon management and sustainable rice production, Adv. Agron., 1997, 58, 151–199 CAS.
- R. Y. Li, J. L. Stroud, J. F. Ma, S. P. McGrath and F. J. Zhao, Mitigation of arsenic accumulation in rice with water management and silicon fertilization, Environ. Sci. Technol., 2009, 43, 3778–3783 CrossRef CAS PubMed.
- J. A. Raven, Cycling silicon - the role of accumulation in plants - Commentary, New Phytol., 2003, 158, 419–421 CrossRef.
- R. Suriyaprabha, G. Karunakaran, R. Yuvakkumar, P. Prabu, V. Rajendran and N. Kannan, Application of silica nanoparticles for increased silica availability in maize, Solid State Phys., 2013, 1512, 424–425 CAS.
- R. Suriyaprabha, G. Karunakaran, R. Yuvakkumar, V. Rajendran and N. Kannan, Silica nanoparticles for increased silica availability in maize (Zea mays. L) seeds under hydroponic conditions, Curr. Nanosci., 2012, 8, 902–908 CrossRef CAS.
- L. Wang, Y. Wang, Q. Chen, W. Cao, M. Li and F. Zhang, Silicon induced cadmium tolerance of rice seedlings, J. Plant Nutr., 2001, 24, 397 CAS.
- S. Kumar, Y. Milstein, Y. Brami, M. Elbaum and R. Elbaum, Mechanism of silica deposition in sorghum silica cells, New Phytol., 2017, 213, 791–798 CrossRef CAS.
- C. C. Zhang, L. J. Wang, W. X. Zhang and F. S. Zhang, Do lignification and silicification of the cell wall precede silicon deposition in the silica cell of the rice (Oryza sativa L.) leaf epidermis, Plant Soil, 2013, 372, 137–149 CrossRef CAS.
- D. K. Tripathi, S. Singh, V. P. Singh, S. M. Prasad, D. K. Chauhan and N. K. Dubey, Silicon nanoparticles more efficiently alleviate arsenate toxicity than silicon in maize cultiver and hybrid differing in arsenate tolerance, Front. Environ. Sci., 2016, 4, 46 Search PubMed.
- C. P. Liu, L. Wei, S. R. Zhang, X. H. Xu and F. B. Li, Effects of nanoscale silica sol foliar application on arsenic uptake, distribution and oxidative damage defense in rice (Oryza sativa L.) under arsenic stress, RSC Adv., 2014, 4, 57227–57234 RSC.
- C. W. He, J. Ma and L. J. Wang, A hemicellulose-bound form of silicon with potential to improve the mechanical properties and regeneration of the cell wall of rice, New Phytol., 2015, 206, 1051–1062 CrossRef CAS.
- M. Greger, A. H. Kabir, T. Landberg, P. J. Maity and S. Lindberg, Silicate reduces cadmium uptake into cells of wheat, Environ. Pollut., 2016, 211, 90–97 CrossRef CAS.
- J. H. Cui, T. X. Liu, F. B. Li, J. C. Yi, C. P. Liu and H. Y. Yu, Silica nanoparticles alleviate cadmium toxicity in rice cells: Mechanisms and size effects, Environ. Pollut., 2017, 228, 363–369 CrossRef CAS.
- J. R. Thomas, A. G. Darvill and P. Albersheim, Structure of plant cell-walls .24. Isolation and structural characterization of the pectic polysaccharide rhamnogalacturonan-Ii from walls of suspension-cultured rice cells, Carbohydr. Res., 1989, 185, 261–277 CrossRef CAS.
- J. Ma, H. M. Cai, C. W. He, W. J. Zhang and L. J. Wang, A hemicellulose-bound form of silicon inhibits cadmium ion uptake in rice (Oryza sativa) cells, New Phytol., 2015, 206, 1063–1074 CrossRef CAS PubMed.
- D. H. Lin and B. S. Xing, Root uptake and phytotoxicity of ZnO nanoparticles, Environ. Sci. Technol., 2008, 42, 5580–5585 CrossRef CAS PubMed.
- J. D. Maksimovic, M. Mojovic, V. Maksimovic, V. Romheld and M. Nikolic, Silicon ameliorates manganese toxicity in cucumber by decreasing hydroxyl radical accumulation in the leaf apoplast, J. Exp. Bot., 2012, 63, 2411–2420 CrossRef.
- X. Y. Yang, Z. H. Zeng, J. Y. Yan, W. Fan, H. W. Bian, M. Y. Zhu, J. L. Yang and S. J. Zheng, Association of specific pectin methylesterases with Al-induced root elongation inhibition in rice, Physiol. Plant., 2013, 148, 502–511 CrossRef CAS PubMed.
- S. K. Chakrabarti, J. C. James and R. G. Mirmira, Quantitative assessment of gene targeting in vitro and in vivo by the pancreatic transcription factor, Pdx1. Importance of chromatin structure in directing promoter binding, J. Biol. Chem., 2002, 277, 13286–13293 CrossRef CAS PubMed.
- A. Koziol, J. Cybulska, P. M. Pieczywek and A. Zdunek, Changes of pectin nanostructure and cell wall stiffness induced in vitro by pectinase, Carbohydr. Polym., 2017, 161, 197–207 CrossRef CAS PubMed.
- S. H. Wang, F. Y. Wang and S. C. Gao, Foliar application with nano-silicon alleviates Cd toxicity in rice seedlings, Environ. Sci. Pollut. Res., 2015, 22, 2837–2845 CrossRef CAS.
- G. Abbas, B. Murtaza, I. Bibi, M. Shahid, N. K. Niazi, M. I. Khan, M. Amjad, M. Hussain and M. Natasha, Arsenic uptake, toxicity, detoxification, and speciation in plants: physiological, biochemical, and molecular aspects, Int. J. Environ. Res. Public Health, 2018, 15, 1–45 Search PubMed.
- M. D. Brand and D. G. Nicholls, Assessing mitochondrial dysfunction in cells, Biochem. J., 2011, 435, 297–312 CrossRef CAS.
- D. C. Logan and C. J. Leaver, Mitochondria-targeted GFP highlights the heterogeneity of mitochondrial shape, size and movement within living plant cells, J. Exp. Bot., 2000, 51, 865–871 CrossRef CAS.
- D. J. Cosgrove, Growth of the plant cell wall, Nat. Rev. Mol. Cell Biol., 2005, 6, 850–861 CrossRef CAS.
- D. Vromman, J. P. Martinez, M. Kumar, Z. Slejkovec and S. Lutts, Comparative effects of arsenite (As(III)) and arsenate (As(V)) on whole plants and cell lines of the arsenic-resistant halophyte plant species Atriplex atacamensis, Environ. Sci. Pollut. Res., 2018, 25, 34473–34486 CrossRef CAS.
- X. F. Zhu, Z. W. Wang, J. X. Wan, Y. Sun, Y. R. Wu, G. X. Li, R. F. Shen and S. J. Zheng, Pectin enhances rice (Oryza sativa) root phosphorus remobilization, J. Exp. Bot., 2015, 66, 1017–1024 CrossRef CAS.
- X. F. Zhu, G. J. Lei, T. Jiang, Y. Liu, G. X. Li and S. J. Zheng, Cell wall polysaccharides are involved in P-deficiency-induced Cd exclusion in Arabidopsis thaliana, Planta, 2012, 236, 989–997 CrossRef CAS.
- N. C. Carpita and D. M. Gibeaut, Structural models of primary-cell walls in flowering plants-consistency of molecular-structure with the physical-properties of the walls during growth, Plant J., 1993, 3, 1–30 CrossRef CAS PubMed.
- A. Peaucelle, S. Braybrook and H. Hofte, Cell wall mechanics and growth control in plants: the role of pectins revisited, Front. Plant Sci., 2012, 3, 1–6 Search PubMed.
- P. Milani, M. Gholamirad, J. Traas, A. Arneodo, A. Boudaoud, F. Argoul and O. Hamant, In vivo analysis of local wall stiffness at the shoot apical meristem in Arabidopsis using atomic force microscopy, Plant J., 2011, 67, 1116–1123 CrossRef CAS.
- N. Schmohl and W. J. Horst, Cell wall pectin content modulates aluminium sensitivity of Zea mays (L.) cells grown in suspension culture, Plant, Cell Environ., 2000, 23, 735–742 CrossRef CAS.
- J. F. Ma, K. Tamai, N. Yamaji, N. Mitani, S. Konishi, M. Katsuhara, M. Ishiguro, Y. Murata and M. Yano, A silicon transporter in rice, Nature, 2006, 440, 688–691 CrossRef CAS.
- J. F. Ma, N. Yamaji, N. Mitani, K. Tamai, S. Konishi, T. Fujiwara, M. Katsuhara and M. Yano, An efflux transporter of silicon in rice, Nature, 2007, 448, U209–U212 CrossRef.
- A. T. Fleck, J. Mattusch and M. K. Schenk, Silicon decreases the arsenic level in rice grain by limiting arsenite transport, J. Plant Nutr. Soil Sci., 2013, 176, 785–794 CAS.
- N. Mitani-Ueno, N. Yamaji, F. J. Zhao and J. F. Ma, The aromatic/arginine selectivity
filter of NIP aquaporins plays a critical role in substrate selectivity for silicon, boron, and arsenic, J. Exp. Bot., 2011, 62, 4391–4398 CrossRef CAS PubMed.
- S. K. Sun, Y. Chen, J. Che, N. Konishi, Z. Tang, A. J. Miller, J. F. Ma and F. J. Zhao, Decreasing arsenic accumulation in rice by overexpressing OsNIP1;1 and OsNIP3;3 through disrupting arsenite radial transport in roots, New Phytol., 2018, 219, 641–653 CrossRef CAS.
Footnote |
† Electronic supplementary information (ESI) available. See DOI: 10.1039/c9en01035a |
|
This journal is © The Royal Society of Chemistry 2020 |