DOI:
10.1039/C9CS00243J
(Review Article)
Chem. Soc. Rev., 2020,
49, 143-179
Synthetic ratiometric fluorescent probes for detection of ions
Received
30th July 2019
First published on 21st November 2019
Abstract
Metal cations and anions are essential for versatile physiological processes. Dysregulation of specific ion levels in living organisms is known to have an adverse effect on normal biological events. Owing to the pathophysiological significance of ions, sensitive and selective methods to detect these species in biological systems are in high demand. Because they can be used in methods for precise and quantitative analysis of ions, organic dye-based ratiometric fluorescent probes have been extensively explored in recent years. In this review, recent advances (2015–2019) made in the development and biological applications of synthetic ratiometric fluorescent probes are described. Particular emphasis is given to organic dye-based ratiometric fluorescent probes that are designed to detect biologically important and relevant ions in cells and living organisms. Also, the fundamental principles associated with the design of ratiometric fluorescent probes and perspectives about how to expand their biological applications are discussed.
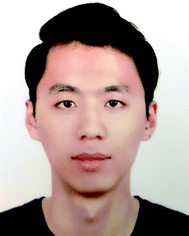
Sang-Hyun Park
| Sang-Hyun Park was born in Seoul, Korea, in 1992. He received his BS degree in Chemistry in 2013 from Purdue University. He has conducted his PhD research under the guidance of Professor Injae Shin of Yonsei University since 2015. As a doctoral student, he is working on identification and mechanistic studies of small molecules that affect apoptosis and/or autophagy as well as mechanistic studies of synthetic ion transporters. |
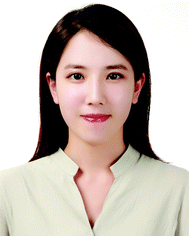
Nahyun Kwon
| Nahyun Kwon obtained her MS degree in Chemistry and Nanoscience from Ewha Womans University. She has conducted her PhD research under the guidance of Professor Juyoung Yoon of Ewha Womans University since 2017. As a doctoral student, she is working on the development of synthetic fluorescent probes, multidimensional drug delivery systems and photodynamic systems. |
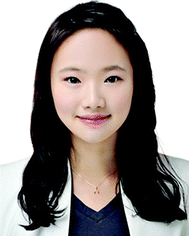
Jee-Hyeon Lee
| Jee-Hyeon Lee received her BS degree in Chemistry in 2017 from the University of Wisconsin-Madison. She has conducted her MS research under the guidance of Professor Injae Shin of Chemistry at Yonsei University since 2018. She is working on functional studies of small molecules that induce neurogenesis or affect apoptosis by suppressing protein–protein interactions. |
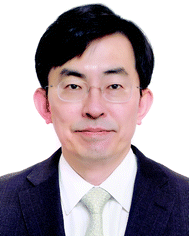
Juyoung Yoon
| Juyoung Yoon received his BS degree from Seoul National University in Korea (1987) and his PhD from The Ohio State University (1994). After completing postdoctoral research at the University of California at Los Angeles (1994–1996) and at The Scripps Research Institute (1996–1998), he joined the faculty at Silla University in 1998. In 2002, he moved to Ewha Womans University, where he is currently a Professor in the Department of Chemistry and Nano Science. He is a Fellow of the Korean Academy of Science and Technology, a Fellow of the Royal Society of Chemistry and a Distinguished Professor of Ewha Womans University. He was listed as a highly cited researcher in chemistry during 2014–2018. His research interests include development of fluorescent probes, activatable photosensitizers, and new organic functional materials. |
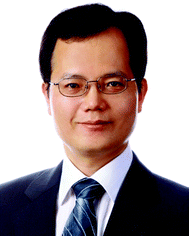
Injae Shin
| Injae Shin received his BS (1985) and MS degrees (1987) in Chemistry from Seoul National University in Korea. His PhD research was performed at the University of Minnesota with Professor Hung-wen Liu (1991–1995). After postdoctoral studies at the University of California at Berkeley with Professor Peter Schultz (1995–1998), he began his independent career as an Assistant Professor of Chemistry at Yonsei University in Korea in 1998 where he became an Associate Professor in 2001 and a Professor in 2006. He is a Fellow of the Korean Academy of Science and Technology and a Fellow of the Royal Society of Chemistry. His research interests include synthesis of various compounds, development of bioactive molecules that regulate biological processes, and functional studies of glycans using chemical tools including glycan microarrays. |
1. Introduction
Owing to their high sensitivity, simplicity and fast response times, fluorescent probes have attracted considerable attention for applications in both optical imaging and analytical sensing.1–6 Fluorescent probes are typically small molecules that can be employed for quantitative determination of analytes in a highly selective and sensitive manner. In the early stages of their development, fluorescent probes were largely designed based on molecular recognition and host–guest chemistry7 and, as a result, they operated in a reversible fashion. In contrast, in the last couple of decades organic reactions have been applied to design self-immolative or reaction based fluorescent probes.8,9 However, quantification of a target analyte using fluorescent probes that possess single emission features can be fraught with difficulty, because various analyte-independent factors, such as instrumental parameters, microenvironments around and local concentrations of probe molecules and photobleaching, interfere with precise analysis. The most effective strategy for overcoming these issues and ensuring reliability involves the use of ratiometric approaches.10,11 Ratiometric fluorescent probes rely on analyte-induced changes in the intensity of two or more emission bands. A ratio of intensities at two wavelengths directly correlates with the concentration of the target analyte and, as a result, these probes possess effective internal referencing systems which greatly increase their sensitivity and improve quantification. Consequently, this approach compensates for environmental factors and eliminates most ambiguities.
Cations and anions are crucial for a variety of biological processes. Dysregulation of specific ion concentrations in cells has a great influence on diverse biological events. For example, although various metal cations, including Cu+/Cu2+, Fe2+/Fe3+, Zn2+ and Na+, are essential for normal biological processes, dysregulation of homeostasis of these ions is closely correlated with the onset of various diseases. In addition, metal cations widely used in industry, such as Hg2+, Cd2+ and Pd species, have adverse effects on humans and other living organisms. Accordingly, fluorescent probes that detect these metal ions have potential use as diagnostic agents.12–17 Furthermore, detection of anions also has drawn great attention in the last few decades because exogenous and endogenous anions in living systems affect normal biological processes.18–20
The design of ratiometric fluorescent probes typically takes advantage of several photophysical properties including internal charge transfer (ICT),21 fluorescence resonance energy transfer (FRET),21 monomer–excimer formation,21 and excited-state intramolecular proton transfer (ESIPT) (Fig. 1).22 ICT fluorophores composed of conjugated electron-donating/electron-withdrawing (donor/acceptor, D/A) moieties typically display large Stokes shifts (Fig. 1a). Upon addition of the analyte, blue- or red-shift of excitation/emission wavelengths occurs owing to the interaction of one of the donor or acceptor moieties with an analyte. FRET relies on energy transfer from an excited state of the donor fluorophore (DF) to the ground state of the acceptor fluorophore (AF) (Fig. 1b). Effective FRET takes place when the emission spectrum of the DF overlaps well with the absorption spectrum of the AF. Generally, flat polycyclic aromatic hydrocarbons, such as anthracene and pyrene, have the ability to form excimers (Fig. 1c). The excited state of a fluorophore of this type can interact with the ground state of another molecule of the fluorophores to form an excited state complex referred to as an excimer. In this case, emission which is red-shifted and broadened arises from the excimer. ESIPT fluorophores display intense emission, light stability and large Stokes shifts (Fig. 1d). For example, upon excitation a preferred enol-form can be transformed into an excited keto tautomer by ESIPT. Relaxation of the excited keto-form regenerates the enol form by reverse proton transfer. Importantly, the ESIPT process is often influenced by pH, hydrogen bonding capacity and solvent polarity.
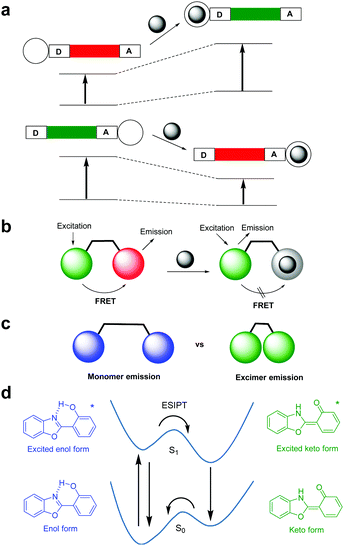 |
| Fig. 1 Photophysical processes responsible for ratiometric fluorescent changes; (a) internal charge transfer (ICT), (b) fluorescence resonance energy transfer (FRET), (c) monomer–excimer formation, and (d) excited-state intramolecular proton transfer (ESIPT). | |
Owing to the biological significance of ions, sensitive and selective methods for their detection in cells are in high demand. Since 2015, a number of synthetic ratiometric fluorescent probes for ions in cells have been reported. The discussion in this review focuses on the results of recent studies (2015–2019) of synthetic ratiometric fluorescent probes, which have been applied to sense exogenous and endogenous metal cations and anions in cells and living organisms. Primary attention is given to progress made in applications of these probes to detect ions in living systems. In addition, design strategies and sensing mechanisms for these probes are also discussed.
2. Ratiometric fluorescent probes for metal ions
2.1 Detection of copper ions
Copper ions are the third most abundant heavy metal ions in humans and other biological systems, and they are a key component of a wide variety of enzymes. Copper ions are implicated in various physiological processes and their homeostasis in humans is crucial for maintaining health. Excessive intake of copper ions is known to cause various symptoms, such as sleepiness and high blood pressure, as well as neurological maladies including Alzheimer's and Parkinson's diseases.23
Because of the physiological and pathological importance of copper ions, ratiometric fluorescent probes have been developed to detect these ions in cells. In this context, several FRET-based, ratiometric fluorescent probes have been developed for this purpose. For example, Ye and coworkers developed probe 1 which consists of a naphthalimide derivative serving as a FRET donor and a rhodamine spirocyclic ring derivative as a precursor of a FRET acceptor (Fig. 2b).24 The intact probe (1 mM Tris–HCl, pH 7.4) exhibits fluorescence emission at 540 nm upon excitation at 420 nm. However, addition of Cu2+ to a solution of 1 leads to a red-shift of the emission from 540 nm to 570 nm. This change is attributed to the turn-on of the FRET system as a consequence of Cu2+-promoted hydrolysis of the spirocyclic ring-closed rhodamine hydrazide to generate its ring-opened counterpart (Fig. 2a).25 The detection limit of 1 for Cu2+ was determined to be 18.6 nM with a response time of less than 1 min. This probe shows high selectivity toward Cu2+ over other metal ions. Probe 1 was applied to image Cu2+ exogenously supplemented in MGC-803 cells (Fig. 2c).
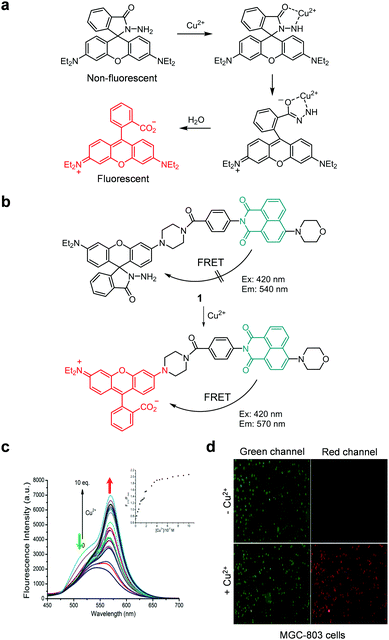 |
| Fig. 2 (a) The Cu2+-promoted hydrolysis of nonfluorescent rhodamine hydrazide to produce strongly fluorescent rhodamine; (b) a proposed mechanism for sensing Cu2+ by 1; (c) Cu2+ concentration-dependent fluorescence changes of 1 (Ex: 420 nm); (d) its application for detection of Cu2+ in MGC-803 cells (reproduced from ref. 24 with permission from Elsevier, copyright 2016). | |
The Cao group also devised a similar FRET-based fluorescent probe, TY-1, composed of a pyrido[1,2-a]benzimidazole derivative as a FRET donor and a rhodamine spirocyclic ring system as a FRET acceptor precursor (Fig. 3).26 The intact probe (10 mM HEPES, pH 7.2) exhibits fluorescence emission at 438 nm upon excitation at 380 nm. However, in response to Cu2+, the intensity of fluorescence of TY-1 at 438 nm decreases significantly concomitant with an increase in emission at 570 nm. The detection limit of TY-1 for Cu2+ was determined to be 42 nM. This probe responds selectively to Cu2+ over other metal ions and was employed to image exogenous Cu2+ in HeLa cells.
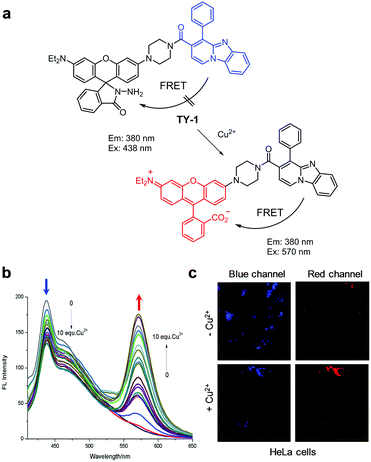 |
| Fig. 3 (a) A proposed mechanism for sensing Cu2+ by TY-1; (b) Cu2+ concentration-dependent fluorescence changes of TY-1 (Ex: 380 nm); (c) its application for detection of Cu2+ in HeLa cells (reproduced from ref. 26 with permission from Elsevier, copyright 2017). | |
In continuing studies, the Ge group designed TY-2, an analogue of TY-1 (Fig. 4).27 The difference between TY-2 and TY-1 is that the former possesses an indolizine instead of a pyrido[1,2-a]benzimidazole moiety as a FRET donor. The fluorescence response of TY-2 to Cu2+ was similar to that of TY-1 and the new probe was also used to image exogenous Cu2+ in glioma cells.
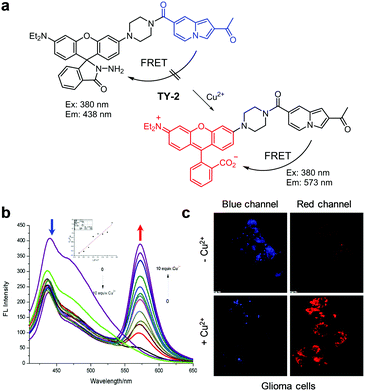 |
| Fig. 4 (a) A proposed mechanism for sensing Cu2+ by TY-2; (b) Cu2+ concentration-dependent fluorescence changes of TY-2 (Ex: 380 nm); (c) its application for detection of Cu2+ in glioma cells (reproduced from ref. 27 with permission from Elsevier, copyright 2017). | |
Zeng and coworkers developed another type of a FRET-based fluorescent probe 2 that targets lysosomal Cu2+ (Fig. 5).28 This probe contains a naphthalimide fluorophore as a FRET donor, a morpholine moiety for targeting lysosomes, and a rhodamine spirocyclic ring system as a FRET acceptor precursor. Probe 2 (H2O/CH3CN = 2
:
1) displays emission at 519 nm when excited at 440 nm. Upon addition of Cu2+, the probe displays an increase in emission at 580 nm and a decrease in emission at 519 nm owing to the turn-on of the FRET process. This probe exhibits high selectivity toward Cu2+ over other metal ions and it has a detection limit of 1.45 nM. The results of a study exploring biological imaging applications show that Cu2+ ions in lysosomes of mouse fibroblast L929 cells can be imaged.
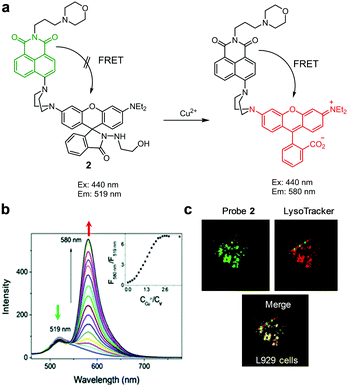 |
| Fig. 5 (a) A proposed mechanism for sensing Cu2+ by 2; (b) Cu2+ concentration-dependent fluorescence changes of 2 (Ex: 440 nm); (c) its application for detection of Cu2+ in L929 cells (reproduced from ref. 28 with permission from the Royal Society of Chemistry, copyright 2017). | |
Later, Wu et al. also reported the lysosome-targeting, FRET-based fluorescent probe 3 for Cu2+ (Fig. 6).29 This probe consists of a pyrrole derivative possessing a 4-(2-aminoethyl)morpholine moiety that serves as a fluorescence donor and spirocyclic rhodamine as a FRET acceptor precursor. Upon excitation at 360 nm (CH3CN/H2O = 8
:
2), the probe emits fluorescence at 486 nm. However, addition of Cu2+ leads to activation of the FRET process between pyrrole and rhodamine moieties, the event which leads to a significant increase in emission arising from the rhodamine fluorophore at 552 nm. This phenomenon is not observed when other metal ions are added. The detection limit of 3 for Cu2+ was determined to be 105 nM. The results of Job's plot analysis showed that this probe formed a 1
:
1 complex with Cu2+. Probe 3 was utilized to detect exogenous Cu2+ in lysosomes of HeLa cells.
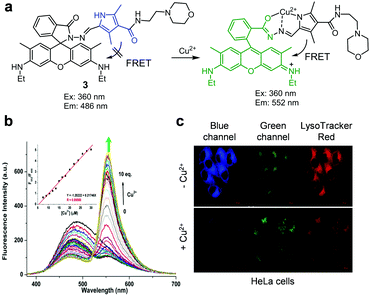 |
| Fig. 6 (a) A proposed mechanism for sensing Cu2+ by 3; (b) Cu2+ concentration-dependent fluorescence changes of 3 (Ex: 360 nm); (c) its application for detection of Cu2+ in lysosomes of HeLa cells (reproduced from ref. 29 with permission from Elsevier, copyright 2019). | |
The group headed by Kang and Lee developed the endoplasmic reticulum (ER)-targeting, ratiometric fluorescent probe 4 for copper ions (Fig. 7).30 The probe itself (20 mM HEPES, pH 7.4) displays fluorescence emission at 480 nm when excited at 410 nm. However, addition of Cu2+ or Cu+ to the probe induces hydrolysis of hydrazide in 4, which leads to a decrease in fluorescence at 480 nm and an increase in emission at 545 nm. Detection limits of 4 for Cu2+ and Cu+ are 1.1 μM and 0.7 μM, respectively. Probe 4 responds to Cu2+ and Cu+ without interference from other metal ions. Finally, the ER of HeLa cells exposed to Cu2+ can be imaged using 4.
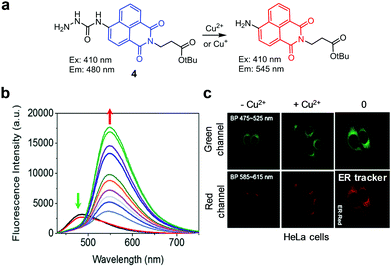 |
| Fig. 7 (a) A proposed mechanism for sensing copper ions by 4; (b) Cu2+ concentration-dependent fluorescence changes of 4 (Ex: 410 nm); (c) its application for detection of Cu2+ in the ER of HeLa cells (reproduced from ref. 30 with permission from the Royal Society of Chemistry, copyright 2017). | |
Zhao and Xu and coworkers designed the aggregation-induced ratiometric fluorescent Cu2+ probe 5 (Fig. 8).31 In CH3OH, probe 5 shows emission at 450 nm when excited at 385 nm but it forms a 2
:
1 complex with Cu2+ in which ICT takes place, leading to an increase in the fluorescence intensity at 511 nm. In aqueous solutions (10 mM HEPES, pH 7.4), the probe displays aggregation-induced emission (AIE) at 480 nm upon excitation at 385 nm. In the presence of Cu2+, the aggregated form of 5 is disassembled to produce a complex of 5 with Cu2+, leading to an increase in fluorescence intensity at 525 nm and a concomitant decrease at 480 nm. The detection limit of 5 for Cu2+ is 21.3 nM and its response time to Cu2+ is less than 15 min. With the exception of Co2+ and Hg2+, which cause slight fluorescence quenching, other metal ions do not induce changes in the fluorescence of 5. This probe was applied to detect Cu2+ supplemented in HeLa cells.
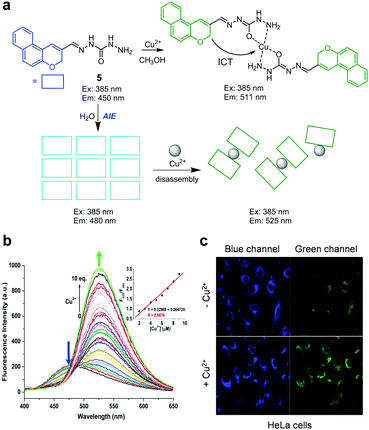 |
| Fig. 8 (a) A proposed mechanism for sensing Cu2+ by 5 in MeOH and water; (b) Cu2+ concentration-dependent fluorescence changes of 5 in aqueous solutions (Ex: 385 nm); (c) its application for detection of Cu2+ in HeLa cells (reproduced from ref. 31 with permission from Elsevier, copyright 2018). | |
The Park and Lee groups developed the ratiometric fluorescent probe 6 for detecting Cu+ (Fig. 9).32 This probe consists of a tripeptide receptor (Trp–Pro–His), which mimics the Cu+ binding site of the cation efflux system protein CusF, and a pyrene fluorophore. In the absence of Cu+ (10 mM HEPES, pH 7.4), 6 displays emission arising from the pyrene moiety at 396 nm upon excitation at 342 nm. Addition of Cu+ induces a red-shift in the emission of 6 from 396 nm to 472 nm. Binding of Cu+ to 6 promotes the interaction between the two pyrene moieties that leads to excimer emission. The binding affinity of 6 with Cu+ is 5.73 × 10−21 M2. This probe ratiometrically responds to Cu+ without interference from other metal ions including Cu+. Probe 6 was applied to detect Cu+ exogenously supplemented in A549 cells.
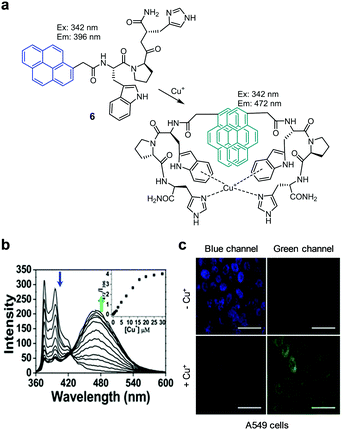 |
| Fig. 9 (a) A proposed mechanism for sensing Cu+ by 6; (b) Cu+ concentration-dependent fluorescence changes of 6 (Ex: 342 nm); (c) its application for detection of Cu+ in A549 cells (reproduced from ref. 32 with permission from Elsevier, copyright 2018). | |
2.2 Detection of Zn2+
Zinc ions, the second most abundant transition metal ions in the human body, play important roles in catalytic functions of various enzymes and in gene transcription induced by transcription factors.33 Most Zn2+ in biological systems is tightly bound to proteins in order to preserve its structural integrity and catalytic functions. However, loosely bound Zn2+ is also found in certain human tissues such as the brain. When free Zn2+ is deficient in the brain, neurons are damaged to induce amyotrophic lateral sclerosis, Alzheimer's and Parkinson's diseases, and epilepsy. On the other hand, an excess of labile Zn2+ causes acute kidney injury. It is also known that labile Zn2+ is generated when cells undergo apoptosis.34 Owing to the pathophysiological significance of free Zn2+ in cells, a number of ratiometric fluorescent probes have been developed to detect the levels of labile Zn2+ in cells.35
For example, Zhang and coworkers devised the ratiometric fluorescent zinc probe HcZ9, which consists of a zinc ion-sensitive fluorophore (ZP1) and the zinc ion-insensitive 7-hydroxycoumarin-3-carboxylic acid (Hc), linked through the peptide Pro9Lys (Fig. 10).36 Intact HcZ9 (50 mM PIPES, 100 mM KCl, pH 7) displays emission at 365–495 nm upon excitation at 360 nm. In the presence of Zn2+, the intensity of the green fluorescence at 495–650 nm (excitation: 485 nm) arising from Zn2+ bound ZP1 increases but the fluorescence emission arising from Hc at 365–495 nm (excitation: 360 nm) remains unchanged. Thus, HcZ9 serves as a dual-excitation, dual-emission probe for Zn2+. However, the binding mode of this probe to Zn2+ was not proposed. This probe, which exhibits high selectivity toward Zn2+ over other metal ions, was employed to determine the level of Zn2+ exogenously supplemented in several mammalian cells.
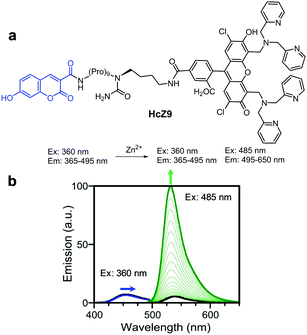 |
| Fig. 10 (a) Structure of HcZ9; (b) Zn2+ concentration-dependent fluorescence changes of HcZ9 (reproduced from ref. 36 with permission from the American Chemical Society, copyright 2014). | |
Bourassa et al. exploited the two-photon ratiometric fluorescent probe Chromis-1 for Zn2+ (Fig. 11).37 In the absence of Zn2+ (10 mM PIPES, 0.1 M KCl, pH 7), Chromis-1 fluoresces at 440 nm with two-photon excitation at 720 nm. However, addition of Zn2+ to the probe leads to a red-shift of the emission from 440 nm to 510 nm. This change is attributed to an increase in ICT when Zn2+ ions bind to the probe. However, the binding mode of the probe to Zn2+ was not proposed. The dissociation constant (Kd) of Chromis-1 and Zn2+ was determined to be 1.5 nM and the probe responded selectively to Zn2+ and not to other metal ions. Chromis-1 was used to image Zn2+ exogenously added in mouse NIH3T3 fibroblasts and endogenous Zn2+ in oligodendrocytes.
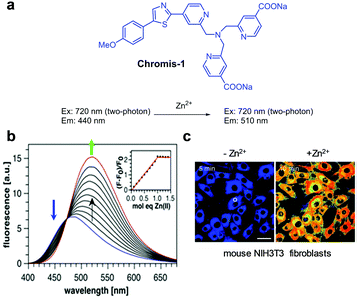 |
| Fig. 11 (a) Structure of Chromis-1; (b) Zn2+ concentration-dependent fluorescence changes of Chromis-1 (Ex: 358 nm); (c) its application for detection of Zn2+ in NIH3T3 fibroblasts (reproduced from ref. 37 with permission from the American Chemical Society, copyright 2018). | |
Santhakumar et al. designed and prepared the bipyridine-bridged bispyrrole-based fluorescent probe BP for ratiometric fluorescence imaging of Zn2+ in biological systems (Fig. 12).38 In this probe, the 2,2′-bipyridine moiety serving as a Zn2+ chelating group is linked to pyrrole donor groups through a π-bridge. When Zn2+ ions bind to BP in phosphate free saline buffer (PFS, pH 7.4), a partial positive charge is generated in the bipyridine moiety and is then transferred to the pyrrole donor moiety via intramolecular charge transfer. This process causes a red-shift of the fluorescence from 510–550 nm to 575–625 nm upon excitation at 460–490 nm. The binding constant of BP to Zn2+ was determined to be 3.6 × 105 M−1. Probe BP was used to detect exogenously supplemented or endogenous Zn2+ in C6 glioma cells and hippocampal slices, respectively.
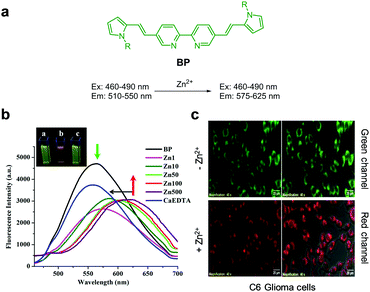 |
| Fig. 12 (a) Structure of BP; (b) Zn2+ concentration-dependent fluorescence changes of BP; (c) its application for detection of Zn2+ in C6 glioma cells (reproduced from ref. 38 with permission from Nature, copyright 2018). | |
Ghosh and coworkers devised the 1,4-dihydroxyanthraquinone-based fluorescent probe 7 for Zn2+ (Fig. 13).39 The intact probe (DMF/H2O = 9
:
1, pH 7.5) exhibits emission at 544 and 567 nm upon excitation at 499 nm. However, binding of Zn2+ to 7 promotes a fluorescence maximum shift to 600 nm, which is associated with the advent of intramolecular charge transfer from the phenolic group to the π system of the quinone moiety. The probe responds selectively to Zn2+ without interference from other metal ions. The limit of detection of 7 for Zn2+ was measured to be 9 × 10−7 M. Probe 7 was used to detect Zn2+ exogenously supplemented in Tecoma stans.
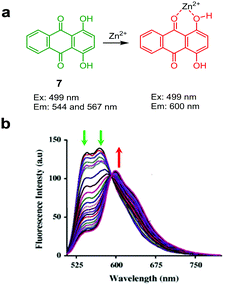 |
| Fig. 13 (a) A proposed mechanism for sensing Zn2+ by 7; (b) Zn2+ concentration-dependent fluorescence changes of 7 (Ex: 499 nm) (reproduced from ref. 39 with permission from Elsevier, copyright 2015). | |
Lee and coworkers developed the ratiometric fluorescent probe 8, which is composed of a symmetric peptide receptor containing two imidazole and two pyrenesulfonamide groups that selectively complex with Zn2+ (Fig. 14).40 Probe 8 (10 mM HEPES, pH 7.4) upon excitation at 342 nm displays an excimer emission at 508 nm associated with two pyrene moieties. However, binding of Zn2+ to 8 induces a decrease in excimer emission at 508 nm and an increase in fluorescence at 379 and 399 nm arising from a monomeric pyrene. Job's plot analysis showed that the probe forms a 1
:
1 complex with Zn2+. The dissociation constant of a complex between 8 and Zn2+ is 300 pM. This probe, which detected Zn2+ without interference arising from other metal ions, was utilized to image Zn2+ exogenously supplemented in RKO cells.
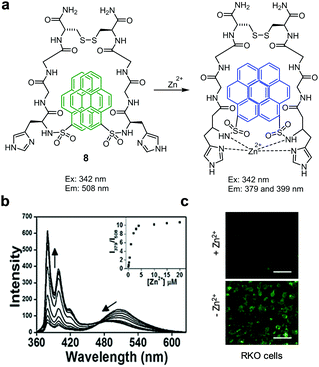 |
| Fig. 14 (a) A proposed mechanism for sensing Zn2+ by 8; (b) Zn2+ concentration-dependent fluorescence changes of 8 (Ex: 342 nm); (c) its application for detection of Zn2+ in RKO cells (reproduced from ref. 40 with permission from Elsevier, copyright 2017). | |
The group headed by Chen and Zhang designed and synthesized the ICT-based fluorescent probe L1 for ratiometric detection of Zn2+ (Fig. 15).41 This probe is composed of a quinoline backbone with tetrahydrofurancarboxamide and phenylethynyl groups at the respective C-8 and C-5 positions. The unbound form of L1 in DMSO/H2O (8
:
2) displays strong emission at 430 nm with excitation at 390 nm. However, addition of Zn2+ to L1 promotes a significant decrease in the intensity of emission at 430 nm and a concomitant increase in fluorescence at 525 nm. The ratiometric fluorescent response of L1 by Zn2+ is attributed to an enhancement of ICT from the amide nitrogen adjacent to the conjugated quinoline moiety. The detection limit of L1 for Zn2+ was measured to be 41 nM and a good linear relationship was found to exist between the fluorescence intensity ratio I525nm/I430nm and the concentration of Zn2+. Probe L1 displays a highly selective response to Zn2+ over other metal ions and it has been applied to monitor Zn2+ exogenously added in HepG2 cells.
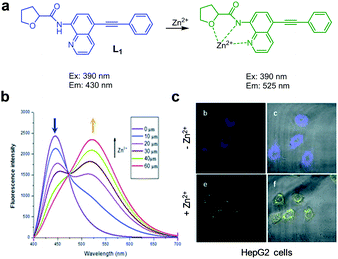 |
| Fig. 15 (a) A proposed mechanism for sensing Zn2+ by L1; (b) Zn2+ concentration-dependent fluorescence changes of L1 (Ex: 390 nm); (c) its application for detection of Zn2+ in HepG2 cells (reproduced from ref. 41 with permission from the Royal Society of Chemistry, copyright 2017). | |
Stasiuk and coworkers designed the Zn2+ selective ratiometric fluorescent probe AQA-F (Fig. 16).42 The probe (10 mM HEPES, pH 7.68) displays an emission maximum at 420 nm with a quantum yield of 0.042 upon excitation at 320 nm. Addition of Zn2+ to a solution of AQA-F leads to an increase in emission at 500 nm with a quantum yield of 0.35. As a consequence, binding of Zn2+ to AQA-F induces a change in its emission profile, enabling its use for determination of Zn2+ levels. The binding constant of AQA-F to Zn2+ was determined to be 15.2 μM. Although the binding mode of Zn2+ to AQA-F was not proposed, the probe showed good selectivity toward Zn2+ over other metal ions. AQA-F was applied to monitor both endogenous and exogenous levels of Zn2+ in healthy prostate RWPE-1 and prostate cancer PC-3 cells. The results of cell image analysis show that normal prostate cells have a higher level of Zn2+ than cancer cells.
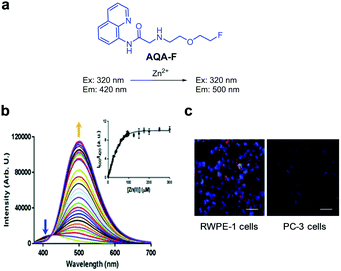 |
| Fig. 16 (a) Structure of AQA-F; (b) Zn2+ concentration-dependent fluorescence changes of AQA-F (Ex: 320 nm); (c) its application for detection of endogenous Zn2+ in RWPE-1 and PC-3 cells (reproduced from ref. 42 with permission from the Royal Society of Chemistry, copyright 2018). | |
The Jin and Tian groups devised an ICT-based ratiometric two-photon fluorescent probe, P-Zn, for imaging Zn2+ in biological systems (Fig. 17).43 In the presence of Zn2+ (50 mM HEPES/MeOH = 1
:
1, pH 7.4), the emission peak of P-Zn at 465 nm induced by two-photon excitation at 800 nm decreases and a new emission peak at 550 nm arises. This change is attributed to an increase in ICT when Zn2+ binds to the probe. The dissociation constant of the complex between P-Zn and Zn2+ was determined to be 2.58 μM and its detection limit for Zn2+ was 15 nM. Because P-Zn responded selectively to Zn2+ without interference from other metal ions, it was used to detect Zn2+ in SHSY-5Y cells and zebrafish.
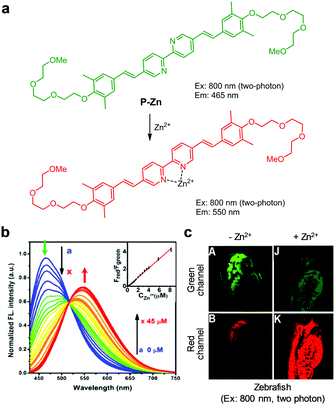 |
| Fig. 17 (a) A proposed mechanism for sensing Zn2+ by P-Zn; (b) Zn2+ concentration-dependent fluorescence changes of P-Zn (Ex: 405 nm); (c) its application for detection of Zn2+ in zebrafish (reproduced from ref. 43 with permission from the American Chemical Society, copyright 2017). | |
Mehdi and coworkers designed the ratiometric fluorescent probe 8AQ, which consists of two naphthylamide-quinoline moieties linked via an isobutylene group (Fig. 18).44 In the absence of Zn2+ (50 mM Tris–HCl, pH 7.5), 8AQ displays fluorescence at 430 nm upon excitation at 366 nm. However, addition of Zn2+ to the probe promotes a decrease in the intensity of emission at 430 nm and a significant increase in emission at 536 nm with a shoulder at 493 nm. The detection limit of 8AQ for Zn2+ was measured to be 2.6 nM and the probe responded selectively to Zn2+ over other metal ions. To evaluate its usefulness in studies of biological systems, 8AQ was used to monitor Zn2+ supplemented in HepG2 cells.
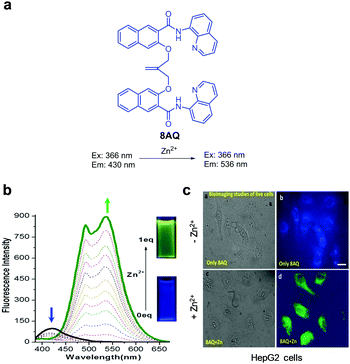 |
| Fig. 18 (a) Structure of 8AQ; (b) Zn2+ concentration-dependent fluorescence changes of 8AQ (Ex: 366 nm); (c) its application for detection of Zn2+ in HepG2 cells (reproduced from ref. 44 with permission from Wiley-VCH, copyright 2017). | |
2.3 Detection of Hg2+
Mercury is a global environmental pollutant distributed in air, water, and soil. Mercury enters the human food chain and eventually accumulates in the human body. Mercury ions (Hg2+) can pass through biological membranes readily and form chelates with mercapto, carboxyl, carbonyl, and amino groups in proteins. Thus, Hg2+ causes serious damage to human health, particularly through its effects on the central nervous and endocrine systems.45 Because of environmental and health related issues, ratiometric fluorescent probes for Hg2+ have been extensively explored.
Various FRET-based fluorescent probes have been developed to ratiometrically detect Hg2+ in cells. For instance, Tian and coworkers constructed the fluorescent probe ITR, which consists of an indole–triazole–rhodamine triad for monitoring Hg2+ (Fig. 19).46 Upon excitation at 390 nm, the probe (EtOH/MOPS = 3
:
7) displays fluorescence at 490 nm arising from the indole–triazole conjugate. Binding of Hg2+ to ITR promotes opening of the spirocyclic ring of the rhodamine dye. As a consequence, FRET from the indole–triazole conjugate to the ring-opened rhodamine dye takes place, leading to a decrease in fluorescence at 490 nm (excitation at 390 nm) from the indole–triazole conjugate and an increase in emission from the ring-opened rhodamine at 590 nm. ITR showed high selectivity for detection of Hg2+ over other metal cations and anions and its detection limit for Hg2+ was calculated to be 11 nM. This probe was utilized for ratiometric fluorescence imaging of Hg2+ supplemented in MCF-7 cells.
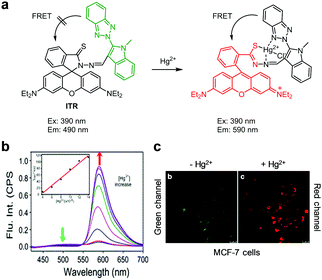 |
| Fig. 19 (a) A proposed mechanism for sensing Hg2+ by ITR; (b) Hg2+ concentration-dependent fluorescence changes of ITR (Ex: 390 nm); (c) its application for detection of Hg2+ in MCF-7 cells (reproduced from ref. 46 with permission from Springer US, copyright 2015). | |
Ratiometric fluorescent probes DRh and 9, which are similar to ITR in that they undergo Hg2+-promoted spirolactam ring-opening of a rhodamine dye, have been developed (Fig. 20 and 21).47,48 In the case of DRh (50 mM Tris–HCl/CH3CN = 9
:
1, pH 7.0), emission from the dansyl dye occurs at 538 nm upon excitation at 350 nm. However, addition of Hg2+ to DRh results in a great reduction of emission at 538 nm and a concomitant increase in fluorescence from a ring-opened rhodamine dye at 582 nm. Probe 9 displays a more prominent Hg2+-promoted change in its fluorescence properties than DRh. Specifically, emission of the benzoxazole donor in 9 (Tris–HCl/CH3CN = 1
:
1, pH 7.4) occurs at 430 nm (excitation at 370 nm) in the absence of Hg2+ but, in the presence of Hg2+, 9 shows a great increase in emission from the rhodamine acceptor at 597 nm (a 167 nm red-shift) concomitant with a decrease in emission from the benzoxazole donor. This change is attributed to the operation of through-bond energy transfer (TBET) from the benzoxazole donor to a ring-opened rhodamine acceptor promoted by binding of Hg2+ to 9. Both DRh and 9 show high selectivity for Hg2+ over other metal ions and have respective detection limits of 0.8 nM and 1.31 nM for Hg2+. Both probes were applied to imaging Hg2+ exogenously supplemented in HeLa cells.
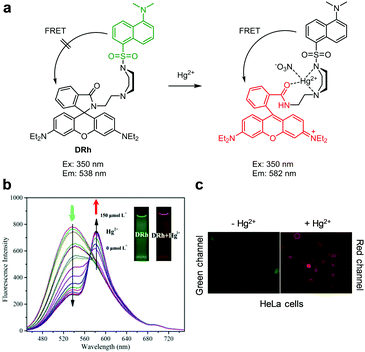 |
| Fig. 20 (a) A proposed mechanism for sensing Hg2+ by DRh; (b) Hg2+ concentration-dependent fluorescence changes of DRh (Ex: 350 nm); (c) its application for detection of Hg2+ in HeLa cells (reproduced from ref. 47 with permission from Elsevier, copyright 2016). | |
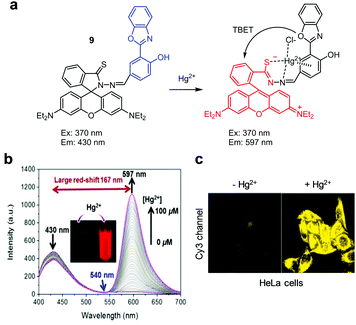 |
| Fig. 21 (a) A proposed mechanism for sensing Hg2+ by 9; (b) Hg2+ concentration-dependent fluorescence changes of 9 (Ex: 370 nm); (c) its application for detection of Hg2+ in HeLa cells (reproduced from ref. 48 with permission from Elsevier, copyright 2018). | |
Several other FRET-based fluorescent probes for Hg2+, including 10, TMUHg-1, TMUHg-2, and TMUHg-3, were designed based on Hg2+-promoted cyclization of a thiosemicarbazide moiety to form a 1,3,4-oxadiazole ring bonded to a rhodamine fluorophore (Fig. 22).49 Probe 10 contains a 7-aminocoumarin group as a FRET donor and a rhodamine derivative as a FRET acceptor precursor (Fig. 23).50 Probe 10 (10 mM HEPES/EtOH = 1
:
1, pH 7.4) displays emission from the 7-aminocoumarin fluorophore at 478 nm (excitation at 440 nm) but, as expected, it does not exhibit rhodamine fluorescence. However, addition of Hg2+ to 10 leads to a great decrease in emission from 7-aminocoumarin and a remarkable increase in fluorescence from the formed rhodamine dye at 587 nm (excitation at 440 nm). This change is attributed to the operation of FRET from 7-aminocoumarin to the ring-opened rhodamine dye. TMUHg-1, TMUHg-2 and TMUHg-3, which contain different FRET donors but the same FRET acceptor as 10, also respond to Hg2+ in a similar way (Fig. 24–26).51–53 The four probes 10, TMUHg-1, TMUHg-2, and TMUHg-3 showed great selectivity toward Hg2+ over other metal cations and they all were successfully employed to ratiometrically image Hg2+ supplemented in mammalian cells.
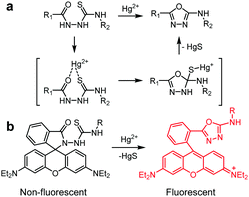 |
| Fig. 22 (a) Hg2+-Induced cyclization of thiosemicarbazide to 1,3,4-oxadiazole and (b) conversion of nonfluorescent rhodamine to strongly fluorescent rhodamine by Hg2+. | |
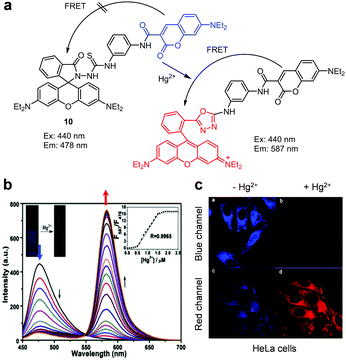 |
| Fig. 23 (a) A proposed mechanism for sensing Hg2+ by 10; (b) Hg2+ concentration-dependent fluorescence changes of 10 (Ex: 440 nm); (c) its application for detection of Hg2+ in HeLa cells (reproduced from ref. 50 with permission from Elsevier, copyright 2015). | |
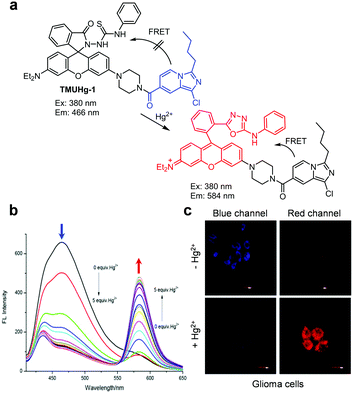 |
| Fig. 24 (a) A proposed mechanism for sensing Hg2+ by TMUHg-1; (b) Hg2+ concentration-dependent fluorescence changes of TMUHg-1 (Ex: 380 nm); (c) its application for detection of Hg2+ in glioma cells (reproduced from ref. 51 with permission from Elsevier, copyright 2017). | |
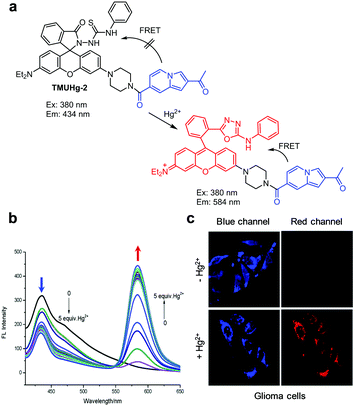 |
| Fig. 25 (a) A proposed mechanism for sensing Hg2+ by TMUHg-2; (b) Hg2+ concentration-dependent fluorescence changes of TMUHg-2 (Ex: 380 nm); (c) its application for detection of Hg2+ in glioma cells (reproduced from ref. 52 with permission from the Royal Society of Chemistry, copyright 2017). | |
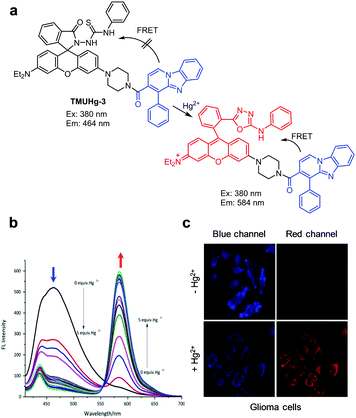 |
| Fig. 26 (a) A proposed mechanism for sensing Hg2+ by TMUHg-3; (b) Hg2+ concentration-dependent fluorescence changes of TMUHg-3 (Ex: 380 nm); (c) its application for detection of Hg2+ in glioma cells (reproduced from ref. 53 with permission from Elsevier, copyright 2017). | |
Tang and coworkers developed the dark through-bond energy transfer (DTBET) based probes, p- and m-TPE-RNS, for ratiometric detection of Hg2+ (Fig. 27).54 Tetraphenylethene (TPE), which possesses an aggregation-induced emission property and a low emission quantum yield in a solution, was used as a dark donor and a rhodamine B derivative was selected as the acceptor in these probes. Probe p-TPE-RNS (CH3CN/H2O = 2
:
3) displays emission at 485 nm upon excitation at 355 nm. However, addition of Hg2+ to the probe promotes cyclization of thiosemicarbazide to form a 1,3,4-oxadiazole linked rhodamine derivative, which undergoes energy transfer from TPE (a dark donor) to the rhodamine moiety (an acceptor), leading to an increase in fluorescence at 595 nm and a decrease at 485 nm. Similarly, m-TPE-RNS displays emission at 480 nm upon excitation at 355 nm, and addition of Hg2+ to the probe leads to an increase in fluorescence at 572 nm and a decrease in emission at 480 nm. Both p- and m-TPE-RNS have great selectivity toward Hg2+ over other metal ions. TPE-RNS was applied to image Hg2+ supplemented in HeLa cells.
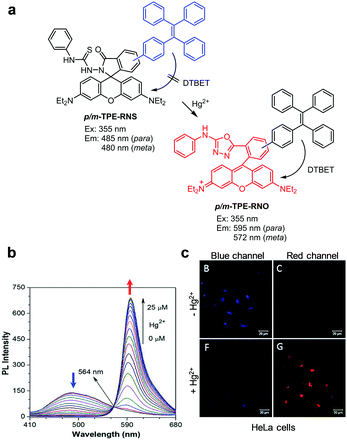 |
| Fig. 27 (a) A proposed mechanism for sensing Hg2+ by TPE-RNS; (b) Hg2+ concentration-dependent fluorescence changes of p-TPE-RNS (Ex: 355 nm); (c) its application for detection of Hg2+ in HeLa cells (reproduced from ref. 54 with permission from the Royal Society of Chemistry, copyright 2017). | |
Wang and coworkers prepared the ratiometric fluorescent probe DPPL, which was designed based on Hg2+-promoted cleavage of a thioacetal group (Fig. 28).55 This probe consists of a diketopyrrolopyrrole dye containing two thioacetal groups and six oligoethylene glycol chains to cause increased water solubility. DPPL itself (THF/HEPES = 1
:
9, pH 7.4) exhibits emission at 535 nm upon excitation at 470 nm. Treatment of the probe with Hg2+ causes cleavage of thioacetal groups in the probe to generate aldehyde groups. This change leads to a decrease in emission at 535 nm and an increase in fluorescence at 610 nm. DPPL responds selectively to Hg2+ without interference from other metal cations. This probe was applied to detect exogenous Hg2+ in HeLa cells.
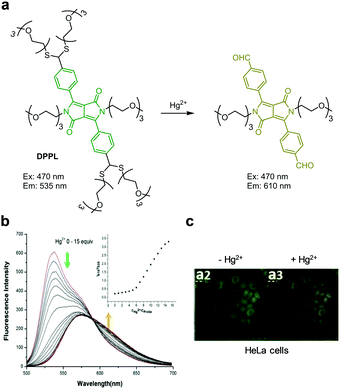 |
| Fig. 28 (a) A proposed mechanism for sensing Hg2+ by DPPL; (b) Hg2+ concentration-dependent fluorescence changes of DPPL (Ex: 470 nm); (c) its application for detection of Hg2+ in HeLa cells (reproduced from ref. 55 with permission from Elsevier, copyright 2016). | |
The reaction-based ratiometric fluorescent probe ATC-Hg for detection of Hg2+ was developed by Yu and coworkers (Fig. 29).56ATC-Hg is composed of a 7-aminocoumarin group containing a 1,3-dithiolane moiety at C-3 and an ortho-2-aminophenyl group at C-4. The intact probe (10 mM PBS, pH 7.4) displays emission at 492 nm upon excitation at 405 nm. When Hg2+ is added to a solution of ATC-Hg, the emission at 492 nm is reduced and concomitantly an increase in fluorescence takes place at 572 nm. This change is attributed to Hg2+-promoted cleavage of 1,3-dithiolane and subsequent condensation of the formed aldehyde group with the amino group in the ortho-2-aminophenyl moiety, which generates a heterocyclic aromatic group in association with a fluorescence change of 7-aminocoumarin brought about by ICT. ATC-Hg exhibits high selectivity toward Hg2+ over other metal cations, and its detection limit for Hg2+ is 27 nM. ATC-Hg was utilized for imaging exogenous Hg2+ in both HeLa and E. coli cells.
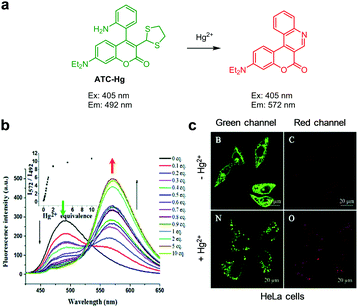 |
| Fig. 29 (a) A proposed mechanism for sensing Hg2+ by ATC-Hg; (b) Hg2+ concentration-dependent fluorescence changes of ATC-Hg (Ex: 405 nm); (c) its application for detection of Hg2+ in HeLa cells (reproduced from ref. 56 with permission from the Royal Society of Chemistry, copyright 2018). | |
Han's group prepared the thiocoumarin-based, ratiometric fluorescent probe MS4 to selectively monitor Hg2+ (Fig. 30).57MS4 contains a thiocarbonyl group, which is converted to the carbonyl group via the desulfurization process promoted by Hg2+. This conversion affects the ICT system. MS4 (10 mM PBS, 1% DMSO, pH 7.4) displays emission at 543 nm with excitation at 420 nm. Upon addition of Hg2+ to the probe, the emission at 543 nm rapidly decreases and a new emission at 477 nm increases. MS4 has high selectivity toward Hg2+ over other metal ions. Fluorescence images of Hg2+ added in HeLa cells were successfully obtained using MS4.
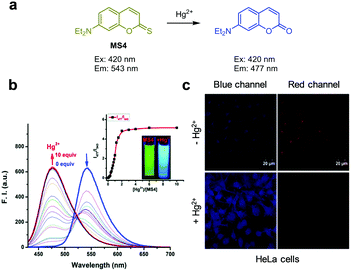 |
| Fig. 30 (a) A proposed mechanism for sensing Hg2+ by MS4; (b) Hg2+ concentration-dependent fluorescence change of MS4 (Ex: 420 nm); (c) application of MS4 for detection of Hg2+ in HeLa cells (reproduced from ref. 57 with permission from the Royal Society of Chemistry, copyright 2018). | |
Yoon and coworkers designed and prepared a two-photon fluorescent probe, NAP-PS, which contains a diphenylphosphinothioyl group at the 14-OH position of a 1,8-naphthalimide fluorophore, to achieve ratiometric detection of Hg2+ (Fig. 31).58 The intact probe (1 mM HEPES, pH 7.4) emits strong blue fluorescence at 450 nm upon two-photon excitation at 740 nm. Addition of Hg2+ to the probe results in a decrease in fluorescence at 450 nm and an increase in emission at 560 nm. Cleavage of the thiophosphinate ester P–O bond in NAP-PS by Hg2+ produces NAP-O−, which displays emission at a longer wavelength via the ICT process between the strong electron-donating oxy-anion and the strong electron-withdrawing naphthalimide group. NAP-PS shows great selectivity toward Hg2+ over other metal ions. NAP-PS was applied for ratiometric detection of supplemented Hg2+ in HeLa cells and rat liver tissues.
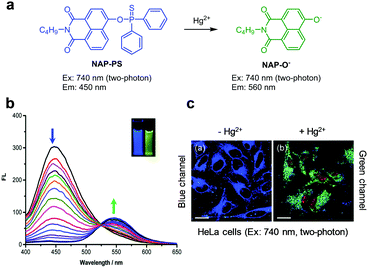 |
| Fig. 31 (a) A proposed mechanism for sensing Hg2+ by NAP-PS; (b) Hg2+ concentration-dependent fluorescence changes of NAP-PS (Ex: 380 nm); (c) its application for detection of Hg2+ in HeLa cells (reproduced from ref. 58 with permission from the Royal Society of Chemistry, copyright 2019). | |
Zeng and coworkers designed and prepared the ratiometric near-infrared (NIR) fluorescent probe L for detection of Hg2+ (Fig. 32).59 Probe L consists of a chromenylium–cyanine fluorophore and two nitrogen, sulphur and oxygen containing side chains for chelating Hg2+. Probe L (EtOH/H2O = 1
:
2) exhibits NIR fluorescence at 728 nm with excitation at 500 nm. However, upon binding of Hg2+ to L, the NIR fluorescence emission at 728 nm is attenuated and a blue-shifted fluorescence band at 663 nm increases as a consequence of weakened ICT. Probe L exhibits high selectivity toward Hg2+ over other metal ions and its detection limit is 9.59 nM. Probe L was utilized to measure a time-dependent ratiometric response to Hg2+ supplemented in mouse fibroblast L929 cells.
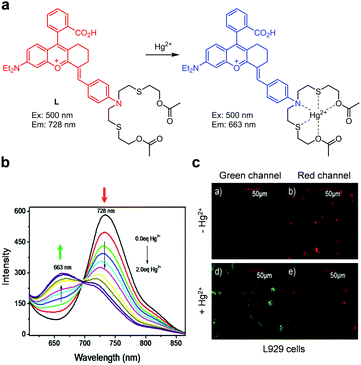 |
| Fig. 32 (a) A proposed mechanism for sensing Hg2+ by L; (b) Hg2+ concentration-dependent fluorescence change of L (Ex: 500 nm); (c) its application for detection of Hg2+ in L929 cells (reproduced from ref. 59 with permission from Elsevier, copyright 2019). | |
Yin and coworkers developed a photo-induced electron transfer (PET)-based ratiometric fluorescent probe, 11, for detection of lysosomal Hg2+ (Fig. 33).60 This probe contains a 4-amino-7-nitrobenzo-2,1,3-oxadiazole fluorophore and a lysosome-targeting morpholine moiety. After treatment with Hg2+, the fluorescence maximum of 11 (20 mM HEPES, pH 7.4) changes from 535 nm to 595 nm upon excitation at 480 nm. This emission change is attributed to the turn-off of the PET process from the morpholine electron donor to the benzo-oxadiazole acceptor upon binding of Hg2+ to 11. Crystallographic analysis shows that a 2
:
2 complex is formed between 11 and Hg2+ through Hg2+ coordination with two nitrogen atoms. Probe 11 exhibited good selectivity toward Hg2+ over other metal cations and it was applied for imaging exogenous Hg2+ in lysosomes of HeLa cells.
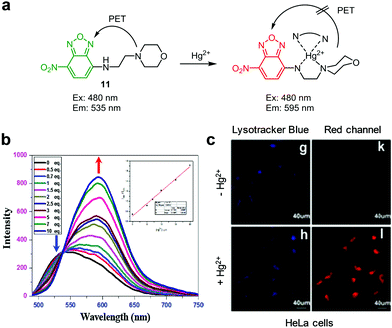 |
| Fig. 33 (a) A proposed mechanism for sensing Hg2+ by 11; (b) Hg2+ concentration-dependent fluorescence changes of 11 (Ex: 480 nm); (c) its application for detection of Hg2+ in HeLa cells (reproduced from ref. 60 with permission from Elsevier, copyright 2016). | |
Yi and coworkers created a polymer nanoparticle-based ratiometric fluorescent probe, NP3, for detection of Hg2+ (Fig. 34).61 This probe consists of a Hg2+-insensitive 4-ethoxy-9-allyl-1,8-naphthalimide (EANI) fluorophore in a core of nanoparticles, and a Hg2+-sensitive fluorescein derivative (AEMH-FITC) serving as the Hg2+-recognition group on the surface of nanoparticles. In the absence of Hg2+, the probe (H2O, pH 7.0) displays dual emissions at 432 nm (EANI) and 528 nm (AEMH-FITC) upon excitation at 405 nm. When Hg2+ ions bind to AEMH-FITC of NP3, FITC fluorescence is effectively quenched via a PET mechanism. As a result, the emission arising from FITC at 528 nm decreases but that of EANI remains unchanged. NP3 exhibits highly selective ratiometric detection of Hg2+ and has a detection limit of 75 nM for Hg2+. This probe was employed to visualize Hg2+ supplemented in HeLa cells.
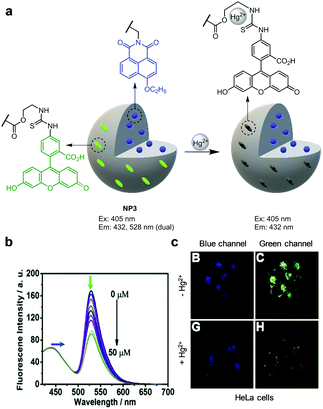 |
| Fig. 34 (a) A proposed mechanism for sensing Hg2+ by NP3; (b) Hg2+ concentration-dependent fluorescence changes of NP3 (Ex: 405 nm); (c) its application for detection of Hg2+ in HeLa cells (reproduced from ref. 61 with permission from Elsevier, copyright 2017). | |
Zhang and coworkers reported the ESIPT-based fluorescent probe Pvi for ratiometric detection of Hg2+ (Fig. 35).62 The intact probe (10 mM PBS, 1% CH3CN, pH 7.4) displays emission at 380 nm upon excitation at 334 nm. After treatment with Hg2+, an emission band at 477 nm increases with a great decrease in fluorescence at 380 nm. This change is associated with Hg2+-promoted cleavage of the enol ether moiety in Pvi to liberate a free hydroxyl group and thus to enhance ESIPT. Pvi exhibited good selectivity for Hg2+ over other metal cations and was utilized to detect Hg2+ added in HeLa cells.
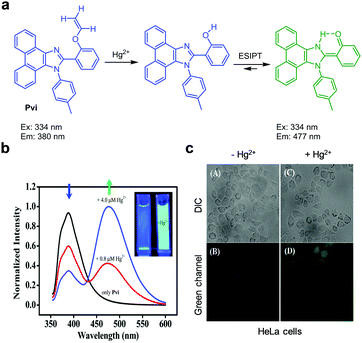 |
| Fig. 35 (a) A proposed mechanism for sensing Hg2+ by Pvi; (b) Hg2+ concentration-dependent fluorescence changes of Pvi (Ex: 334 nm); (c) its application for detection of Hg2+ in HeLa cells (reproduced from ref. 62 with permission from the Royal Society of Chemistry, copyright 2015). | |
Wang et al. designed the NIR ratiometric three-channel fluorescent probe HCy-SeH for combined detection of the superoxide anion radical (O2˙−) and Hg2+ (Fig. 36).63HCy-SeH (10 mM HEPES, pH 7.4) does not fluoresce but its oxidation by O2˙− produces Cy-SeH which emits red fluorescence at 800 nm upon excitation at 755 nm. Cy-SeH also exists in equilibrium with its conjugate base, Cy
Se, which exhibits a green emission at 567 nm upon excitation at 405 nm. In the presence of Hg2+, Cy-SeH or Cy
Se is transformed into Keto-Cy, which emits fluorescence at 607 nm (excitation at 510 nm). Thus, HCy-SeH can be used as a dual-responsive probe for the associated detection of O2˙− and Hg2+. Analysis of HEK293 cell images using HCy-SeH revealed that accumulation of Hg2+ in cells induces a burst of O2˙−. This probe was also successfully applied to image O2˙− and Hg2+ in mice models.
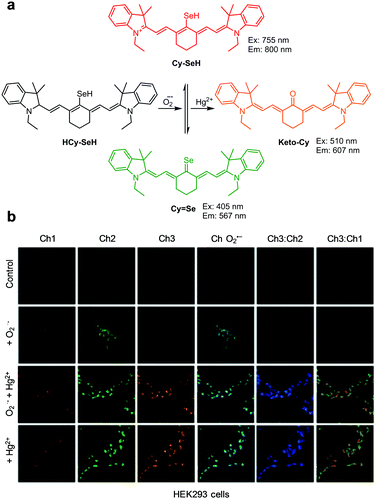 |
| Fig. 36 (a) A proposed mechanism for combined detection of O2˙− and Hg2+ by HCy-SeH; (b) its application for detection of O2˙− and/or Hg2+ in HEK293 cells (reproduced from ref. 63 with permission from the American Chemical Society, copyright 2018). | |
2.4 Detection of Fe2+/Fe3+
Iron ions (Fe2+/Fe3+) are essential for several vital cellular activities in all living organisms.64 Fe2+ and Fe3+ are mainly present in the form of complexes with proteins and play key roles in oxygen transport and storage, electron transfer, redox and many other functions. In living systems, entry of Fe3+ into cells is facilitated by transferrin where it is either stored or reduced to form Fe2+. Fe2+ is transported into cells as a complex with divalent metal transporters 1 (DMT-1) and distributes to organelles such as mitochondria and nucleus. Free Fe2+ can induce oxidative stress by generating hydroxyl radicals, which cause oxidative damage to various biomolecules. Excess or limited amounts of labile Fe2+ in living systems promote atherosclerosis or anemia, respectively. Owing to their physiological and pathological significance in living organisms, ratiometric fluorescent probes have been devised to detect Fe2+ and Fe3+ in cells.
Goel and coworkers developed the ratiometric fluorescent probe NAP-3, which consists of a naphtha[2,1-b][1,10]-phenanthroline (NAP) moiety conjugated to donor–acceptor and chromophoric π-groups (Fig. 37).65 In aqueous solutions (H2O/DMSO = 9
:
1, pH 7.4), NAP-3 displays green-yellow fluorescence at 544 nm upon excitation at 365 nm. However, binding of Fe3+ to NAP-3 leads to a large decrease in the intensity of emission at 544 nm and an increase in emission at 605 nm (excitation at 365 nm). The detection limit of NAP-3 for Fe3+ was determined to be 9.1 nM. This probe selectively responds to Fe3+ without interference from other metal ions. NAP-3 was employed to monitor exogenous Fe3+ in human liver carcinoma HepG2 cells and endogenous Fe3+ in FTN-1 (iron storing proteins) silenced C. elegans.
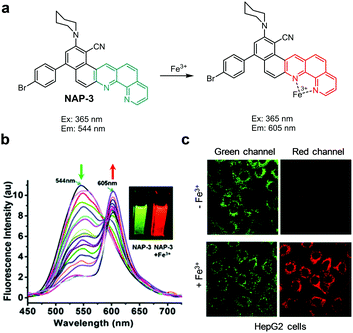 |
| Fig. 37 (a) A proposed mechanism for sensing Fe3+ by NAP-3; (b) Fe3+ concentration-dependent fluorescence changes of NAP-3 (Ex: 365 nm); (c) its application for detection of Fe3+ in HepG2 cells (reproduced from ref. 65 with permission from the Royal Society of Chemistry, copyright 2015). | |
Geng et al. devised the bis-thiazole based ratiometric fluorescent probe L for Fe3+ detection (Fig. 38).66 The probe itself displays strong fluorescence at 448 nm with excitation at 385 nm. Fe3+ (EtOH/H2O = 9
:
1) binds to L to form the 2
:
1 complex FeL2, in which a chelation-enhanced fluorescence (CHEF) process causes an increase in the intensity of an emission band at 486 nm and a decrease in emission at 448 nm. Probe L has a detection limit of 0.6 μM for Fe3+. The association constant of L with Fe3+ to form FeL2 was determined to be 2.76 × 103 M−2. The probe, which responded to Fe3+ and Hg2+ but not to other metal ions, was applied to detect Fe3+ supplemented in HeLa cells and in zebrafish.
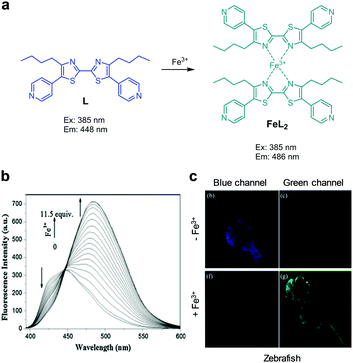 |
| Fig. 38 (a) A proposed mechanism for sensing Fe3+ by L; (b) Fe3+ concentration-dependent fluorescence changes of L (Ex: 385 nm); (c) its application for detection of Fe3+ in zebrafish (reproduced from ref. 66 with permission from Elsevier, copyright 2016). | |
Chang's research group reported the results of studies leading to the development of the endoperoxide reactivity-based FRET probe FIP-1 for ratiometric fluorescent detection of Fe2+ (Fig. 39).67 This probe is composed of fluorescein and Cy3, which are linked through an Fe2+-cleavable endoperoxide bridge. In the probe, FRET between fluorescein and Cy3 takes place with an efficiency of ca. 85%. Thus, the intact probe (50 mM HEPES, pH 7.4) exhibits fluorescence at 556 nm upon excitation at 488 nm. However, cleavage of the endoperoxide bridge in the probe via an Fe2+-triggered process leads to disruption of FRET from a fluorescein donor to a Cy3 acceptor. As a result, the intensity of fluorescence from the fluorescein moiety in the probe at 515 nm increases. FIP-1 responds to Fe2+ and Cu+ but not to other metal ions. This probe was used to detect labile Fe2+ in MDA-MB-231 cells treated with 35MEW28, a ferroptosis inducer.
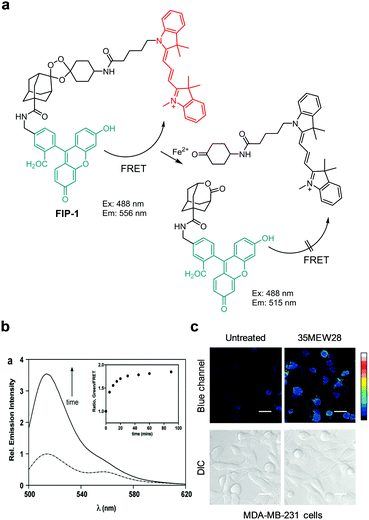 |
| Fig. 39 (a) A proposed mechanism for sensing Fe2+ by FIP-1; (b) fluorescence spectrum of FIP-1 in the absence and presence of Fe2+ (Ex: 488 nm); (c) its application for detection of Fe2+ in MDA-MB-213 cells (reproduced from ref. 67 with permission from the American Chemical Society, copyright 2016). | |
Tian and coworkers devised a nanocluster-based ratiometric fluorescent probe, AuNC@FeL@Cy7, to monitor Fe2+ (Fig. 40).68 The nanoprobe consists of a gold nanocluster (AuNC) serving as a fluorophore, a ligand (FeL) for Fe2+, and a water-soluble sulfocyanine 7 (Cy7) dye which is covalently attached to AuNC@FeL as an internal reference. In the absence of Fe2+, the nanoprobe displays emission at both 622 and 820 nm (excitation at 552 nm) arising from the respective AuNC@FeL and Cy7 groups. When Fe2+ ions bind to the FeL moiety, the fluorescence arising from AuNC in the probe at 622 nm decreases greatly owing to charge transfer from AuNCs to Fe2+ but the emission band at 820 nm remains almost unchanged. The response time of AuNC@FeL@Cy7 to Fe2+ is ca. 1 s and its detection limit for Fe2+ is calculated to be 210 nM. This probe has great selectivity toward Fe2+ over other metal ions and is utilized to detect Fe2+ exogenously supplemented in neurons and HepG2 cells.
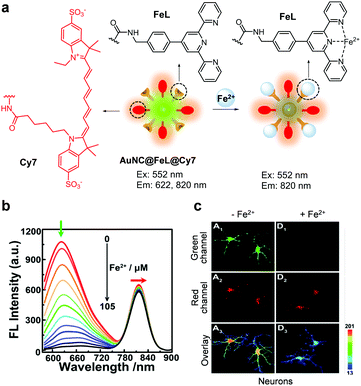 |
| Fig. 40 (a) A proposed mechanism for sensing Fe2+ by AuNC@FeL@Cy7; (b) Fe2+ concentration-dependent fluorescence changes of AuNC@FeL@Cy7 (Ex: 552 nm); (c) its application for detection of Fe2+ in neurons (reproduced from ref. 68 with permission from the American Chemical Society, copyright 2018). | |
2.5 Detection of Pd species
Palladium (Pd) is widely used in chemical, industrial, electrical and pharmaceutical applications. For example, Pd is utilized in dental materials, automobile catalytic converters and electrical contacts69 as well as in catalysts for carbon–carbon or carbon–heteroatom bond forming reactions that produce fine chemicals or medicines.70 It is known that Pd2+ ions adversely affect human health by binding to DNA, proteins and other biomolecules and thus by disrupting various biological processes.71 Because of its biological significance, fluorescent probes have been exploited to ratiometrically detect palladium.
Zhang and coworkers designed a through-bond energy transfer (TBET)-based, two-photon ratiometric fluorescent probe, Np-Rh-Pd, to detect Pd2+ (Fig. 41).72 This probe is composed of a naphthalene derivative with a D–π–A structure serving as a two-photon fluorescence donor and a spirocyclic ring-closed rhodamine derivative as a fluorescence acceptor precursor. Np-Rh-Pd itself (PBS/EtOH = 1
:
1, pH 7.4) displays emission from the naphthalene derivative at 495 nm upon one-photon excitation at 405 nm or two-photon excitation at 780 nm. When Pd2+ ions bind to Np-Rh-Pd, the intensity of a new fluorescence band at 595 nm increases and the emission at 495 nm decreases. This change is a consequence of the operation of a process involving turn-on of rhodamine fluorescence by Pd2+-promoted spirolactam ring opening of a rhodamine moiety followed by energy transfer from the naphthalene derivative to a rhodamine dye. Np-Rh-Pd has a detection limit of 230 nM for Pd2+ and it has great selectivity for Pd2+ without interference from biothiols and other metal cations. Np-Rh-Pd was used for ratiometric fluorescence imaging of Pd2+ supplemented in HeLa cells and mouse liver tissues.
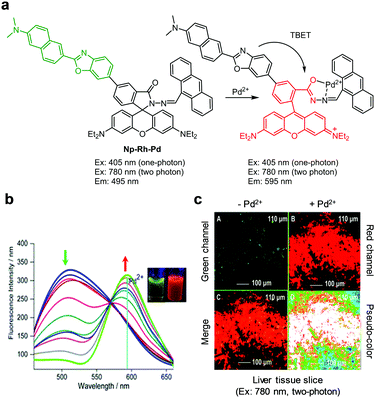 |
| Fig. 41 (a) A proposed mechanism for sensing Pd2+ by Np-Rh-Pd; (b) Pd2+ concentration-dependent fluorescence changes of Np-Rh-Pd (Ex: 415 nm); (c) its application for detection of Pd2+ in liver tissue slices (reproduced from ref. 72 with permission from the American Chemical Society, copyright 2015). | |
Lin and coworkers reported the results of a study of the FRET-based ratiometric fluorescent probe CR-Pd for imaging Pd2+ in cells (Fig. 42).73 This probe is composed of a 7-aminocoumarin group as an energy donor and a rhodamine moiety as an energy acceptor. In the absence of Pd2+, CR-Pd (10 mM PBS/EtOH = 1
:
1, pH 7.4) displays fluorescence arising from 7-aminocoumarin at 472 nm upon excitation at 400 nm. When Pd2+ ions bind to CR-Pd, a new emission band forms at 594 nm and the intensity of the band at 472 nm decreases. This phenomenon is attributed to the turn-on of rhodamine fluorescence via spirolactam ring opening and the FRET process from 7-aminocoumarin to rhodamine. CR-Pd has high selectivity for Pd2+ over other ions and is utilized to detect exogenous Pd2+ in mitochondria of HeLa and A549 cells.
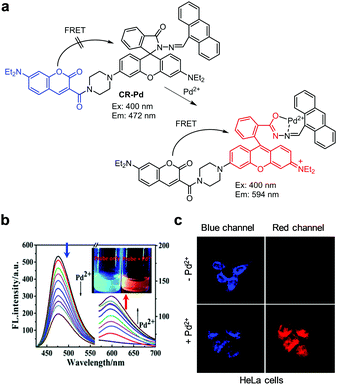 |
| Fig. 42 (a) A proposed mechanism for sensing Pd2+ by CR-Pd; (b) Pd2+ concentration-dependent fluorescence changes of CR-Pd (Ex: 400 nm); (c) its application for detection Pd2+ in HeLa cells (reproduced from ref. 73 with permission from the Royal Society of Chemistry, copyright 2019). | |
Feng and coworkers created the FRET-based ratiometric fluorescent probe 12 for detection of palladium (Fig. 43).74 Probe 12 contains a 3-aminophthalimide moiety as a fluorescence donor and a rhodamine/fluorescein hybrid (rhodol) as a fluorescence acceptor, which are linked through a piperazine ring. Probe 12 (10 mM HEPES/CH3CN = 4
:
1, pH 7.4) displays fluorescence arising from 3-aminophthalimide at 490 nm with excitation at 390 nm. Addition of palladium to a solution of 12 leads to removal of the allyloxycarbonyl group attached to a rhodol moiety in the probe, which results in fluorescence emission of the rhodol dye. Subsequently, energy transfer from 3-aminophthalimide to rhodol via both ESIPT and FRET causes an increase in fluorescence at 547 nm when excited at 390 nm. Probe 12 shows high sensitivity for palladium with a detection limit of 31 nM, and responds to Pd0, Pd2+ and Pd4+ to a similar degree, but not to other cations, anions, amino acids and biothiols. This probe was used for imaging palladium supplemented in HeLa cells.
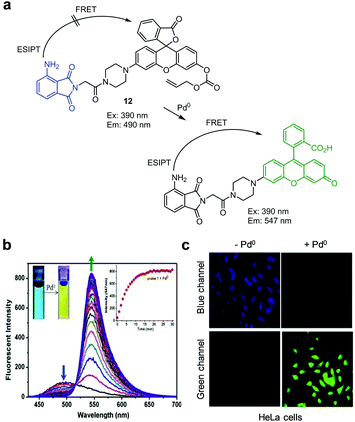 |
| Fig. 43 (a) A proposed mechanism for sensing palladium by 12; (b) Pd0 concentration-dependent fluorescence changes of 12 (Ex: 390 nm); (c) its application for detection of Pd0 in HeLa cells (reproduced from ref. 74 with permission from Elsevier, copyright 2018). | |
Zhang and coworkers designed and prepared the ratiometric two-photon fluorescent probe Np-Pd for detection of Pd2+ (Fig. 44).75 This probe (10 mM PBS, 1% DMSO, pH 7.4) exhibits fluorescence at 445 nm with two-photon excitation at 820 nm. In the presence of Pd2+, removal of the propargyl group from Np-Pd takes place to induce a change in the emission maximum from 445 nm to 550 nm. Np-Pd has a detection limit of 280 nM for Pd2+ and shows a selective response to Pd2+ over biothiols and other metal ions. This probe was used for ratiometric fluorescence imaging of Pd2+ supplemented in HeLa cells and mouse liver tissues.
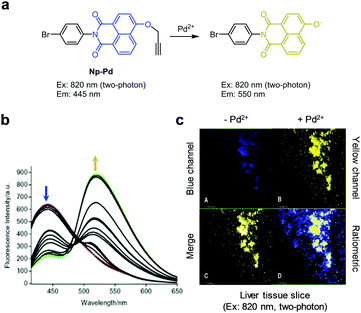 |
| Fig. 44 (a) A proposed mechanism for sensing Pd2+ by Np-Pd; (b) Pd2+ concentration-dependent fluorescence changes of Np-Pd (Ex: 375 nm); (c) its application for detection of Pd2+ in a liver tissue slice (reproduced from ref. 75 with permission from Elsevier, copyright 2016). | |
Liu and coworkers developed the two-photon ratiometric ESIPT fluorescent probe 13 for detection of Pd0, Pd2+ and Pd4+ (Fig. 45).76 The probe (10 mM PBS/THF = 9
:
1, pH 7.4) exhibits fluorescence emission at 470 nm with one-photon excitation at 380 nm or two-photon excitation at 760 nm. However, addition of palladium species to a solution of 13 leads to a change in its emission maximum from 470 nm to 552 nm. This phenomenon is attributed to the operation of an ESIPT process between carbonyl and hydroxyl groups triggered by Pd0-induced cleavage of the allyloxycarbonyl group in 13. This probe has a detection limit of 9 nM for palladium and exhibits good selectivity for palladium over other metal ions. Probe 13 was utilized to image exogenous palladium in HeLa cells and cervical tissue slices.
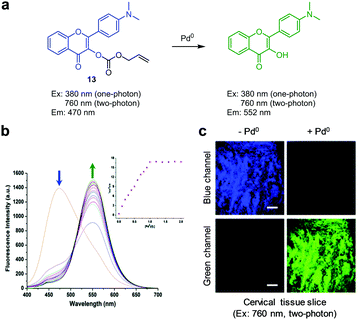 |
| Fig. 45 (a) A proposed mechanism for sensing palladium by 13; (b) Pd0 concentration-dependent fluorescence changes of 13 (Ex: 380 nm); (c) its application for detection of Pd0 in a cervical tissue slice (reproduced from ref. 76 with permission from the Royal Society of Chemistry, copyright 2017). | |
2.6 Detection of Al3+
Aluminium is one of the most abundant metal elements in the earth's crust. In excess amounts, the metal is harmful to plants and animals. In addition, long-term exposure or intake of Al3+ causes various human diseases owing to accumulation of the ion in various organs. In particular, an excess amount of Al3+ in brain tissues causes severe damage to the central nervous system and thus a high risk for neurological diseases.77 Therefore, selective and sensitive methods for detection of Al3+ in biological systems are in great demand.
The Zeng and Wu groups developed a ratiometric fluorescent probe, Py-L-COOH, to detect Al3+ (Fig. 46).78 The intact probe (DMSO/H2O = 15
:
85) exhibits emission at 381 nm with excitation at 353 nm. Addition of Al3+ to a solution of Py-L-COOH leads to the formation of a 1
:
2 complex from which excimer emission occurs at 475 nm in concert with a decrease in emission at 381 nm. Py-L-COOH responds selectively to Al3+ without interference from other metal ions and has a detection limit of 0.29 μM for Al3+. This probe was employed to detect Al3+ supplemented in HeLa cells.
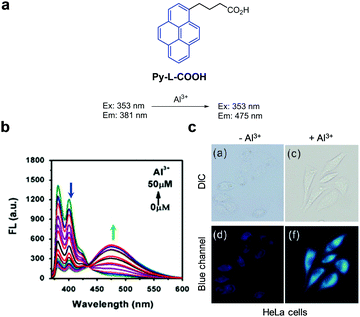 |
| Fig. 46 (a) A proposed mechanism for sensing Al3+ by Py-L-COOH; (b) Al3+ concentration-dependent fluorescence changes of Py-L-COOH (Ex: 353 nm); (c) its application for detection of Al3+ in HeLa cells (reproduced from ref. 78 with permission from Elsevier, copyright 2016). | |
Xu and coworkers designed and synthesized the ratiometric fluorescent Al3+ probe FA, which consists of 4-methyl-3-hydroxyflavone containing an electron-withdrawing allyloxycarbonyl group at the 3-OH position (Fig. 47).79FA itself (H2O/CH3CN = 3
:
1) displays emission at 395 nm with excitation at 348 nm. In the presence of Al3+, the fluorescence emission at 451 nm increases through operation of an excited-state charge transfer (ESCT) process but the emission at 395 nm remains unchanged. FA has a detection limit of 0.75 μM for Al3+ and responds selectively to Al3+ with a binding constant of 4.9 × 104 M−1. Analysis of Job's plot shows that FA forms a 2
:
1 complex with Al3+. FA was used to detect Al3+ supplemented in HeLa cells.
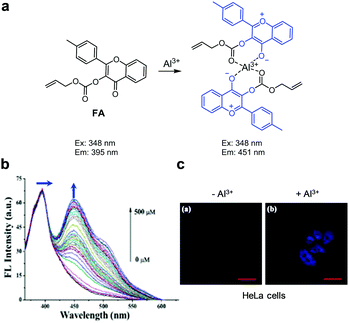 |
| Fig. 47 (a) A proposed mechanism for sensing Al3+ by FA; (b) Al3+ concentration-dependent fluorescence changes of FA (Ex: 348 nm); (c) its application for detection of Al3+ in HeLa cells (reproduced from ref. 79 with permission from Elsevier, copyright 2018). | |
2.7 Detection of Na+
A sodium ion is the primary cation in extracellular fluids in animals. The intracellular sodium ion concentration is ca. 10 mM and its extracellular concentration is ca. 150 mM.80 High concentrations of Na+ in blood causes edema, thirst and reduced urine production.81 On the other hand, a low level of Na+ in blood leads to headache, confusion and seizures. Although Na+ is crucial for the function of normal biological processes, only a limited number of probes for ratiometric detection of this ion have been developed.
One example of a ratiometric fluorescent probe for Na+ is NaGY-AM, which consists of benzophosphole P-oxide and aza-crown ether serving as a sodium ion binder (Fig. 48).82 In the absence of Na+, NaGY-AM (50 mM HEPES, 1% DMSO, pH 7.4) displays emission at 656 nm when excited at 405 nm. However, binding of Na+ to the aza-crown ether ring in the probe leads to a decrease in fluorescence at 656 nm and an increase in emission at 620 nm. This hypsochromic shift is attributed to a reduction of ICT resulting from binding of Na+ to NaGY-AM. This probe has high selectivity toward Na+ with a dissociation constant of 16.0 mM. NaGY-AM was applied to visualize exogenous Na+ in HeLa cells.
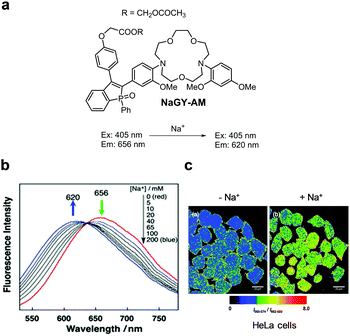 |
| Fig. 48 (a) Structure of NaGY-AM; (b) Na+ concentration-dependent fluorescence changes of NaGY-AM (Ex: 405 nm); (c) its application for detection of Na+ in HeLa cells (reproduced from ref. 82 with permission from the Royal Society of Chemistry, copyright 2015). | |
2.8 Detection of Co2+
Cobalt is an important metal for humans and other organisms. The amount of cobalt present in the human body is 1.1–1.5 mg, 43% of which is stored in muscle tissue, 14% in bone and 43% in other soft tissues.83 This metal is involved in diverse biological processes, such as hematopoiesis and metabolism of fats, and is a component of vitamin B12. However, exposure to an excess of cobalt is associated with various diseases including cardiomyopathy and interstitial lung disease. Owing to the importance of cobalt homeostasis to physiology and pathology, ratiometric fluorescence probes that selectively monitor cobalt in biological systems have been developed.
The Xu and Qian groups investigated the ratiometric fluorescent Co2+ probe E3, which was designed based on the operation of an ICT mechanism (Fig. 49).84 The intact probe (H2O/EtOH = 6
:
4) displays fluorescence at 528 nm when excited at 440 nm. However, addition of Co2+ to E3 causes a blue-shift of the emission from 528 nm to 474 nm. This change may be attributed to the triggering of ICT by binding of Co2+ to four nitrogen atoms in two aminomethyl pyridine arms in E3. This probe shows a selective response to Co2+ without interference from other metal ions. E3 was employed to detect Co2+ supplemented in HeLa cells.
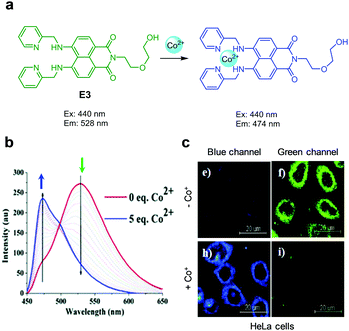 |
| Fig. 49 (a) A proposed mechanism for sensing Co2+ by E3; (b) Co2+ concentration-dependent fluorescence changes of E3; (c) its application for detection of Co2+ in HeLa cells (reproduced from ref. 84 with permission from the Royal Society of Chemistry, copyright 2015). | |
2.9 Detection of Cd2+
Cadmium, one of the most toxic transition metals, is widely utilized in industry. This metal ion (Cd2+) accumulates in soil, plants and other organisms. Cd2+ induces lipid peroxidation and affects antioxidant enzymes.85 In addition, it inhibits DNA synthesis and cell division. Long-term exposure of humans to cadmium causes various diseases including cancers and neurodegenerative diseases.
The Qian and Zhu groups designed and synthesized the ratiometric fluorescent probe NJ1Cd to monitor Cd2+ (Fig. 50).86 This probe is composed of a 6-dimethylaminoquinoline group as the fluorophore and a hydrazinopyridine moiety as the Cd2+ chelator. In the absence of Cd2+, NJ1Cd (PBS, 0.25% DMSO, pH 7.4) displays emission at 515 nm upon excitation at 375 nm. However, binding of Cd2+ to the probe leads to a red-shift in emission from 515 nm to 570 nm. This change is attributed to the occurrence of intramolecular charge transfer between the electron donor and the electron acceptor after binding of Cd2+ to the probe. The probe responds selectively to Cd2+ among other metal ions tested. NJ1Cd was employed to image Cd2+ added into HeLa cells.
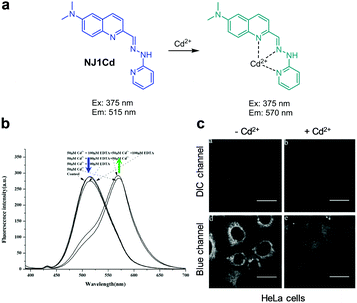 |
| Fig. 50 (a) A proposed mechanism for sensing Cd2+ by NJ1Cd; (b) fluorescence changes of NJ1Cd by Cd2+ (Ex: 375 nm); (c) its application for detection of Cd2+ in HeLa cells (reproduced from ref. 86 with permission from J-STAGE, copyright 2016). | |
The Siva and Chellappa groups designed and prepared the ratiometric fluorescent probe BIMC to detect Cd2+ (Fig. 51).87 The probe itself (HEPES/CH3CN = 9
:
1, pH 7.5) displays emission at 530 nm with excitation at 340 nm. When Cd2+ ions bind to heteroatoms in BIMC, their lone pair electron donating ability is lost, thereby suppressing intramolecular charge transfer. Consequently, addition of Cd2+ to BIMC causes a blue-shift of emission from 530 nm to 418 nm. The detection limit of BIMC was calculated to be 1.5 × 10−10 M for Cd2+. This probe also responds to F− with a detection limit of 1.5 × 10−10 M. However, other metal ions do not alter the fluorescence of BIMC. The results of Job's plot analysis and MS studies show that binding of BIMC to Cd2+ or F− forms a 1
:
1 complex. This probe was applied to detect exogenous Cd2+ in H9c2 cells.
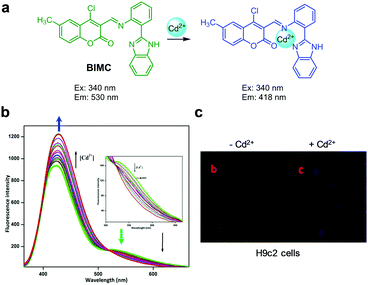 |
| Fig. 51 (a) A proposed mechanism for sensing Cd2+ by BIMC; (b) Cd2+ concentration-dependent fluorescence changes of BIMC (Ex: 340 nm); (c) its application for detection of Cd2+ in H9c2 cells (reproduced from ref. 87 with permission from Elsevier, copyright 2018). | |
2.10 Detection of Ag+
Silver and silver ion containing substances are widely used in pharmaceutical and imaging industries.88 Discharging Ag+ into ecosystems affects food and agriculture, and long-term exposure to excess Ag+ can cause silver poisoning, heart enlargement, skin damage and growth retardation. On the other hand, Ag+ has beneficial bactericidal activity.
Tang and coworkers created the coumarin-based ratiometric fluorescent probe CHa for detection of Ag+ (Fig. 52).89 The intact probe exhibits emission at 520 nm with excitation at 430 nm, but addition of Ag+ leads to a blue-shift of the fluorescence from 520 nm to 450 nm. This change is attributed to Ag+-induced hydrolysis of the hydrazide group in CHa, which generates 3-formylcoumarin that emits at 450 nm. The detection limit of CHa is 61 nM and it has high selectivity toward Ag+ over other metal ions. This probe was applied to detect exogenous Ag+ in HepG2 and HeLa cells.
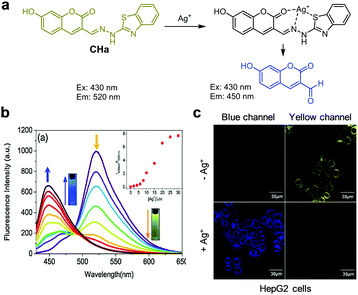 |
| Fig. 52 (a) A proposed mechanism for sensing Ag+ by CHa; (b) Ag+ concentration-dependent fluorescence changes of CHa (Ex: 430 nm); (c) its application for detection of Ag+ in HeLa cells (reproduced from ref. 89 with permission from Elsevier, copyright 2018). | |
2.11 Detection of Ho3+
Holmium is a rare earth element. Substances containing trivalent holmium are used in agriculture, industry and medicine. Holmium suppresses proliferation of bone marrow cells in mice, causes oxidative stress, and decreases the activities of antioxidant enzymes that lead to abnormal production and accumulation of cellular reactive oxygen species (ROS).90 In addition, Ho3+ induces chromosome aberration and DNA damage by promoting cleavage of DNA.
The Yin and Huo groups devised the 1,8-naphthalimide-based, ratiometric fluorescent probe 14 for detection of Ho3+ (Fig. 53).91 The free probe 14 displays emission at 512 nm upon excitation at 385 nm. However, addition of Ho3+ to 14 causes a decrease in fluorescence intensity at 512 nm and the simultaneous formation of a new band at 480 nm owing to coordination of Ho3+ to the hydroxyl and aldehyde groups of the probe. Job's plot analysis indicates that a 1
:
2 complex is formed between Ho3+ and 14. The association constant of the complex is 6.37 × 1011 M−1 and the probe exhibits a detection limit of 6 × 10−8 M for Ho3+. This probe has high selectivity to Ho3+ over other metal ions tested. Probe 14 was employed to image exogenous Ho3+ in A549 cells.
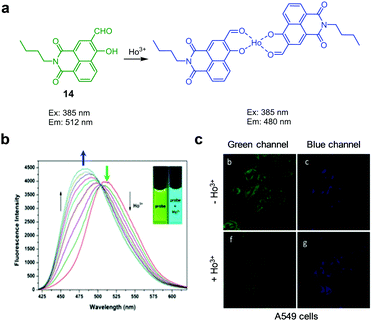 |
| Fig. 53 (a) A proposed mechanism for sensing Ho3+ by 14; (b) Ho3+ concentration-dependent fluorescence changes of 14 (Ex: 380 nm); (c) its application for detection of Ho3+ in A549 cells (reproduced from ref. 91 with permission from Elsevier, copyright 2017). | |
2.12 Detection of gold ions
It has been shown recently that gold-catalyzed reactions are useful in the synthesis of complex organic molecules.92 Unlike elemental gold which is inert, its ionic forms Au+ and Au3+ are reactive and have the ability to bind to biomolecules such as proteins and DNA.93 As a consequence, gold ions perturb various biological processes and occasionally cause severe health problems. For instance, intake of AuCl3 causes damage to the peripheral nervous system or human organs such as the kidneys and liver.94 Because of the physiological and pathological importance of gold ions, ratiometric fluorescent probes have been developed for their detection in cells.95
Emrullahoğlu and coworkers synthesized the BODIPY-based, ratiometric fluorescent probe BURAK-1, which responds to Au3+ (Fig. 54).96 The free BURAK-1 (PBS/EtOH = 6
:
4, pH 7.0) exhibits emission at 562 nm upon excitation at 460 nm. However, when Au3+ ions are added to a solution of BURAK-1, a gold-catalyzed intramolecular cyclization reaction takes place to transform the enynone group in the probe to a furan derivative.97 This event results in a decrease in emission at 562 nm and an increase in fluorescence at 516 nm. It was found that this probe responds to Au3+, Au+ and Hg2+ without interference from other metal cations. BURAK-1 was applied to image Au3+ supplemented in A549 cells.
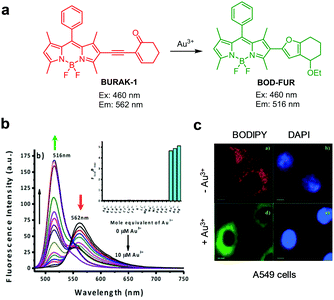 |
| Fig. 54 (a) A proposed mechanism for sensing Au3+ by BURAK-1; (b) Au3+ concentration-dependent fluorescence changes of BURAK-1 (Ex: 460 nm); (c) its application for detection of Au3+ in A549 cells (reproduced from ref. 96 with permission from Wiley-VCH, copyright 2015). | |
3. Ratiometric fluorescent probes for anions
3.1 Detection of bisulfite and sulfite ions
In water, sulfur oxide (SO2) is converted into bisulfite (HSO3−) and sulfite anions (SO32−) that are closely related to neuron damage in cells, lung cancer and cardiovascular diseases.98 Endogenous SO2 is generated by aspartate aminotransferase 2 in mitochondria and then converted to HSO3− and SO32−.99 Accordingly, ratiometric sensing of bisulfite and sulfite anions has become an active area of the study.100
Most of the ratiometric fluorescent probes for HSO3−/SO32− have been developed based on nucleophilic addition reactions that disrupt π-conjugate systems and cause a blue-shift of fluorescence maxima. For example, Feng and coworkers reported the results of studies on the ratiometric fluorescent probe 15, which is composed of 7-aminocoumarin and indolium moieties (Fig. 55).101 Addition of HSO3−/SO32− to 15 (20 mM PBS/CH3OH = 1
:
1, pH 7.4) gives rise to a new emission band at 485 nm with a decrease in emission at 667 nm when excited at 450 nm. The detection limit of 15 for HSO3−/SO32− is 27 nM. This probe was applied to detect HSO3− in food and serum samples, filter paper and a dipstick system, and to image exogenous bisulfite in HeLa cells.
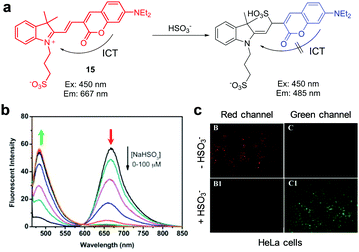 |
| Fig. 55 (a) A proposed mechanism for sensing HSO3− by 15; (b) HSO3− concentration-dependent fluorescence changes of 15 (Ex: 450 nm); (c) its application for detection of HSO3− in HeLa cells (reproduced from ref. 101 with permission from Elsevier, copyright 2015). | |
The same research group designed and prepared the closely related fluorescent probe 16, which consists of 7-amino-4-azacoumarin and indolium moieties, for ratiometric detection of bisulfite (Fig. 56).102 Probe 16 (10 mM PBS/DMF = 9
:
1, pH 7.4) undergoes a ratiometric fluorescence change in the form of a decrease in emission at 717 nm and an increase in fluorescence at 560 nm (excitation at 500 nm) upon addition of bisulfite. A linear relationship was observed to exist between the magnitude of this change and the concentration of HSO3− in the range of 0–4 μM, and the probe had a detection limit of 87 nM. Probe 16 was applied to detect bisulfite in food samples and to monitor exogenous bisulfite as well as endogenously generated bisulfite in HeLa cells.
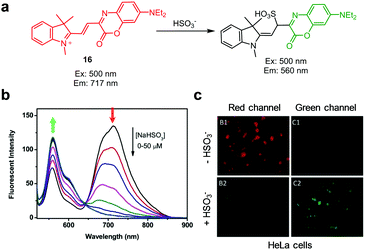 |
| Fig. 56 (a) A proposed mechanism for sensing HSO3−/SO32− by 16; (b) HSO3− concentration-dependent fluorescence changes of 16 (Ex: 500 nm); (c) its application for detection of HSO3− in HeLa cells (reproduced from ref. 102 with permission from Elsevier, copyright 2017). | |
Yu and coworkers developed the carbazole-linked benzoindolium derivative CZ-Id for ratiometric detection of HSO3−/SO32− in mitochondria of cells (Fig. 57).103 This probe itself (10 mM PBS/DMF = 7
:
3, pH 7.4) displays emission at 590 nm with excitation at 350 nm. However, upon addition of bisulfite to the probe, a decrease in the intensity of emission at 590 nm occurs in concert with an increase in a fluorescence band at 490 nm. The detection limit of CZ-Id for bisulfite is 0.15 μM and a linear relationship exists between the magnitude of the emission change and a concentration of HSO3− in the 0–50 μM range. CZ-Id has the ability to detect endogenous and exogenous bisulfite in mitochondria of HeLa cells.
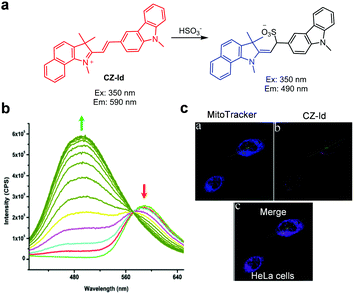 |
| Fig. 57 (a) A proposed mechanism for sensing HSO3− by CZ-Id; (b) HSO3− concentration-dependent fluorescence changes of CZ-Id (Ex: 350 nm); (c) its application for detection of HSO3− in HeLa cells (reproduced from ref. 103 with permission from the Royal Society of Chemistry, copyright 2015). | |
Liu et al. described a similar type of a ratiometric fluorescent probe, Cl-2, which can be used to image mitochondrial bisulfite in cells (Fig. 58).104Cl-2 (10 mM PBS, pH 7.4) shows emission at 625 nm with excitation at 405 nm. Addition of bisulfite (or SO2) to a solution of the probe causes a decrease in the intensity of emission at 625 nm and a concomitant increase in fluorescence at 463 nm. A linear correlation exists between the magnitude of this change and bisulfite concentration in the 0–20 μM range. The probe has a detection limit of 58 nM. Cl-2 was employed to monitor mitochondrial bisulfite. Furthermore, by using 2,4-dinitrophenyl sulfonamide as a synthetic SO2 donor, this probe was utilized to demonstrate that the level of SO2 in mitochondria of HeLa cells is 4.32 μM.
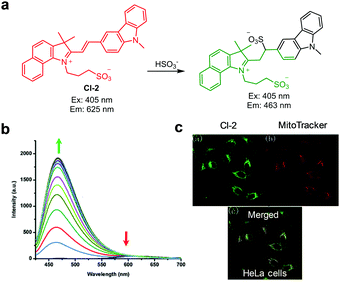 |
| Fig. 58 (a) A proposed mechanism for sensing HSO3− by Cl-2; (b) HSO3− concentration-dependent fluorescence changes of Cl-2 (Ex: 405 nm); (c) its application for detection of HSO3− in HeLa cells (reproduced from ref. 104 with permission from the Royal Society of Chemistry, copyright 2016). | |
Another related ratiometric fluorescent probe CZBI, bearing carbazole and benzoindolium moieties, was devised for detection of bisulfite (Fig. 59).105 In the presence of HSO3−, the original fluorescence band of CZBI (EtOH/PBS = 1
:
9, pH 7.4) at 588 nm decreases with an increase in fluorescence at 462 nm. Here again, the response linearly correlates with HSO3− concentration in the range of 0–16 μM, and the probe has a detection limit of 10 nM. Probe CZBI displays excellent mitochondria-localization. As a result, it was employed to determine that the level of SO2, generated using N-benzyl-2,4-dinitrophenyl sulfonamide as a synthetic SO2 donor, in mitochondria of HeLa cells is 2.6 μM.
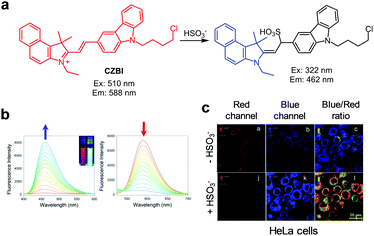 |
| Fig. 59 (a) A proposed mechanism for sensing HSO3− by CZBI; (b) HSO3− concentration-dependent fluorescence changes of CZBI ((left) Ex: 510 nm before treatment with HSO3−; (right) Ex: 322 nm after treatment with HSO3−); (c) its application for detection of HSO3− in HeLa cells (reproduced from ref. 105 with permission from Elsevier, copyright 2016). | |
A benzo[e]indolium derivative, 17, was also shown to be a ratiometric fluorescent probe for bisulfite (Fig. 60).106 This probe itself (10 mM PBS, pH 7.4) displays emission at 578 nm with excitation at 400 nm, but a new emission band at 466 nm arises with a decrease in the original emission upon addition of bisulfite. The detection limit of 17 for bisulfite is 0.15 μM and a linear correlation exists between the emission change and bisulfite concentration in the 0–24 μM range. Probe 17 was applied to detect bisulfite present in real samples, such as various types of sugars and a paper strip. Finally, exogenous bisulfite in HeLa cells was imaged using 17.
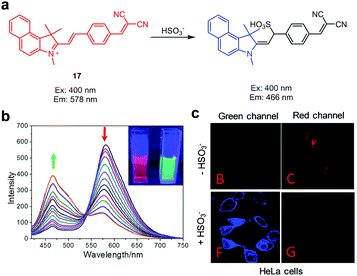 |
| Fig. 60 (a) A proposed mechanism for sensing HSO3− by 17; (b) HSO3− concentration-dependent fluorescence changes of 17 (Ex: 400 nm); (c) its application for detection of HSO3− in HeLa cells (reproduced from ref. 106 with permission from the Royal Society of Chemistry, copyright 2016). | |
Wang et al. devised the hemicyanine derivative NBD-Id as a ratiometric fluorescent probe for HSO3− (Fig. 61).107 Addition of HSO3− to NBD-Id (50 mM PBS, pH 7.4) induces a dramatic blue-shift of the fluorescence from 593 nm to 476 nm when excited at 404 nm. The response of the probe to bisulfite is completed within 20 s. The detection limit of NBD-Id for HSO3− is 3.6 nM. Probe NBD-Id was employed to detect HSO3− in mitochondria of MCF-7 cells.
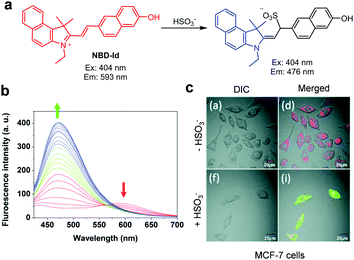 |
| Fig. 61 (a) A proposed mechanism for sensing HSO3− by NBD-Id; (b) HSO3− concentration-dependent fluorescence changes of NBD-Id (Ex: 404 nm); (c) its application for detection of HSO3− in MCF-7 cells (reproduced from ref. 107 with permission from the Royal Society of Chemistry, copyright 2017). | |
A carbazole–indolium based fluorescent probe, DCI, for bisulfite was developed by Wang et al. (Fig. 62).108 The intact probe (10 mM PBS, pH 7.4) displays strong fluorescence at 628 nm when excited at 350 nm. Addition of bisulfite to the probe induces an increase in a new emission band at 507 nm and a simultaneous decrease in the original emission band at 628 nm. A linear relationship was observed between 0–40 μM bisulfite with a detection limit of 30 μM. DCI detects bisulfite in mitochondria of HeLa cells.
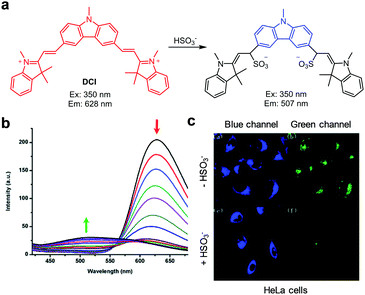 |
| Fig. 62 (a) A proposed mechanism for sensing HSO3− by DCI; (b) HSO3− concentration-dependent fluorescence changes of DCI (Ex: 350 nm); (c) its application for detection of HSO3− in HeLa cells (reproduced from ref. 108 with permission from the Royal Society of Chemistry, copyright 2016). | |
Probes EIM and EIS display selective fluorescent responses to HSO3−/SO32− (Fig. 63).109 Upon addition of HSO3− to these probes (PBS, pH 7.4), their emission at ca. 580 nm decreases and a great increase in the fluorescence band at ca. 480 nm occurs. The ratiometric fluorescence changes undergone by these probes are attributed to the disruption of the conjugated double bond network. The detection limits of EIM and EIS for bisulfite are 0.20 μM and 0.11 μM, respectively. The two probes were applied to image exogenous HSO3− and endogenous HSO3−, generated by using 2,4-dinitrophenyl sulfonamide as a SO2 donor, in HepG2 cells. It was found that EIM and EIS locate mostly in mitochondria.
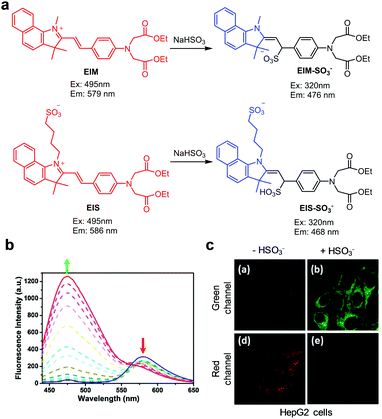 |
| Fig. 63 (a) A proposed mechanism for sensing HSO3− by EIM; (b) HSO3− concentration-dependent fluorescence changes of EIM; (c) its application for detection of HSO3− in HepG2 cells (reproduced from ref. 109 with permission from Elsevier, copyright 2018). | |
The dual-hemicyanine derivative SHC (PBS, 1% DMSO, pH 7.4) displays fluorescence at 600 nm with excitation at 380 nm (Fig. 64).110 However, addition of bisulfite to the probe leads to an increase in emission at 480 nm with a decrease in fluorescence at 600 nm. The detection limit of SHC for bisulfite is 0.1 μM with a linear response to bisulfite concentration in the range of 0–10 μM. This probe was utilized to image bisulfite in HepG2 cells. It was found that this probe is located mainly in mitochondria.
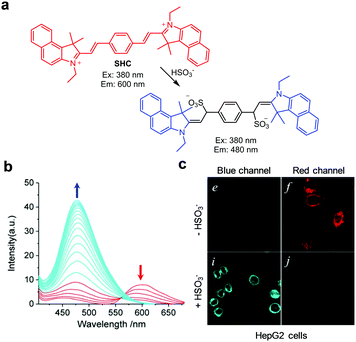 |
| Fig. 64 (a) A proposed mechanism for sensing HSO3− by SHC; (b) HSO3− concentration-dependent fluorescence change of SHC (Ex: 380 nm); (c) its application for detection of exogenous HSO3− in HepG2 cells (reproduced from ref. 110 with permission from the American Chemical Society, copyright 2018). | |
Zhang et al. reported studies leading to the development of the ratiometric NIR fluorescent probe 18 for HSO3−, in which naphthopyran and benzothiazolium moieties are conjugated (Fig. 65).111 Probe 18 (10 mM PBS/DMSO = 9
:
1, pH 7.4) displays emission at 630 nm when excited at 400 nm, but upon addition of HSO3− a new band at 520 nm increases with a decrease in emission at 630 nm. The detection limit of 18 for SO32−/HSO3− is 95 nM. Interestingly, addition of H2O2 to the adduct, formed by reactions of 18 with bisulfite, induces sulfite elimination leading to the recovery of the original fluorescence pattern. This probe was used to detect bisulfite supplemented in MCF-7 cells and zebrafish.
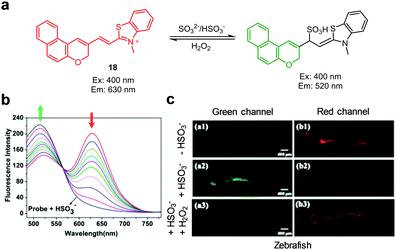 |
| Fig. 65 (a) A proposed mechanism for sensing HSO3− and SO32− by 18; (b) HSO3− concentration-dependent fluorescence changes of 18 (Ex: 400 nm); (c) its application for detection of HSO3− in zebrafish (reproduced from ref. 111 with permission from the American Chemical Society, copyright 2017). | |
Probe CZBT (EtOH/PBS = 1
:
4, pH 7.4), which is composed of benzothiazolium and carbazole moieties, exhibits a ratiometric fluorescence change from 552 nm to 460 nm (excitation at 335 nm) in the presence of HSO3− (Fig. 66).112 The detection limit of CZBT for bisulfite is 28 nM with a linear response to bisulfite in the range of 0–12 μM. This probe was employed to image HSO3− in HeLa cells and monitor this ion in water samples.
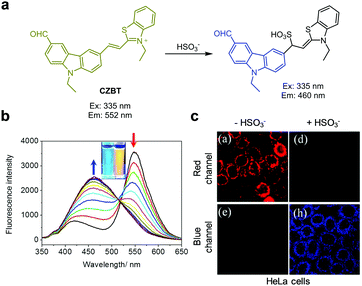 |
| Fig. 66 (a) A proposed mechanism for sensing HSO3− by CZBT; (b) HSO3− concentration-dependent fluorescence changes of CZBT (Ex: 335 nm); (c) its application for detection of HSO3− in HeLa cells (reproduced from ref. 112 with permission from Elsevier, copyright 2018). | |
The human serum albumin (HSA)-based ratiometric fluorescent probe HE-HSA-B1 was developed for detection of bisulfite by Ma et al. (Fig. 67).113 This probe consists of HE serving as a HSO3−-sensitive fluorophore and microenvironment-sensitive BODIPY B1 located inside HSA. The two dyes act as a FRET pair. Intact HE-HSA-B1 displays fluorescence at 695 nm when excited at 450 nm as a consequence of FRET from B1 to HE. However, addition of bisulfite to the probe causes an increase in emission associated with B1 at 517 nm with a decrease in the original emission arising from HE at 695 nm. This change is attributed to quenching of the FRET process promoted by the addition of bisulfite to HE in the probe. The detection limit of HE-HSA-B1 for HSO3− is calculated to be 99 nM. This system was applied to detect bisulfite supplemented in MCF-7 cells.
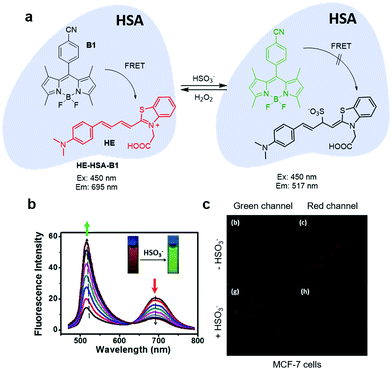 |
| Fig. 67 (a) A proposed mechanism for sensing HSO3− by HE-HSA-B1; (b) HSO3− concentration-dependent fluorescence changes of HE-HSA-B1 (Ex: 450 nm); (c) its application for detection of HSO3− in MCF-7 cells (reproduced from ref. 113 with permission from Elsevier, copyright 2018). | |
Zhen et al. reported another type of ratiometric fluorescent probe, APCT, for bisulfite-selective detection (Fig. 68).114 Probe APCT (Tris–HCl/DMSO = 3
:
7, pH 7.4) has a fluorescence band at 560 nm, which shifts to 510 nm upon addition of bisulfite. The shift is attributed to the disruption of the π-conjugated system in the probe induced by addition of bisulfite. The detection limit of APCT for bisulfite is 6.1 μM with a linear response to bisulfite in the range of 20–120 μM. This probe was applied to image exogenous bisulfite in HeLa cells.
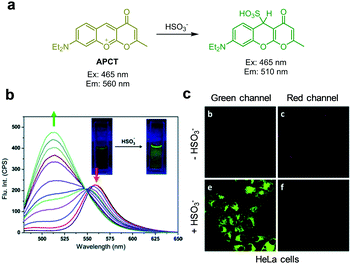 |
| Fig. 68 (a) A proposed mechanism for sensing HSO3− by APCT; (b) HSO3− concentration-dependent fluorescence changes of APCT (Ex: 465 nm); (c) its application for detection of HSO3− in HeLa cells (reproduced from ref. 114 with permission from the Royal Society of Chemistry, copyright 2017). | |
Lia et al. developed the ratiometric fluorescent probe QPCT that possesses a core structure similar to that of APCT (Fig. 69).115 The fluorescence of QPCT (PBS/DMSO = 3
:
7, pH 7.4) is hysochromically shifted from 590 nm to 537 nm (excitation at 490 nm) after treatment with bisulfite. QPCT displays high selectivity toward bisulfite because it does not respond to other anions and biothiols. A linear correlation of bisulfite concentration was observed in the range of 0–120 μM with a detection limit of 0.44 μM. QPCT was also applied to monitor HSO3− in A549 cells.
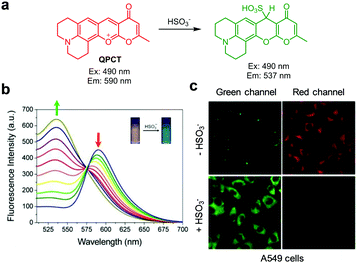 |
| Fig. 69 (a) A proposed mechanism for sensing HSO3− by QPCT; (b) HSO3− concentration-dependent fluorescence changes of QPCT (Ex: 490 nm); (c) its application for detection of HSO3− in A549 cells (reproduced from ref. 115 with permission from Elsevier, copyright 2019). | |
Probe BICO, consisting of coumarin and benzimidazole moieties, displays emission at 605 nm with excitation at 415 nm (Fig. 70).116 However, when bisulfite is added to the probe (PBS, 1 mM cetyltrimethyl ammonium bromide (CTAB), pH 7.4), a new emission band at 458 nm arises. The fluorescence wavelength shift results from the disruption of the π-conjugated system promoted by the addition of bisulfite to the probe. The detection limit of BICO for bisulfite is 53 nM with a linear bisulfite concentration range of 1–21 μM. This probe was applied to detect bisulfite in real samples, such as dry white wine, sugar and test strips. In addition, bisulfite ions exogenously supplemented in A549 cells were imaged using BICO.
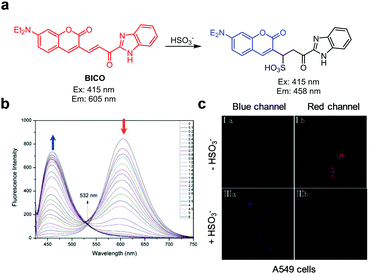 |
| Fig. 70 (a) A proposed mechanism for sensing HSO3− by BICO; (b) HSO3− concentration-dependent fluorescence changes of BICO (Ex: 415 nm); (c) its application for detection of HSO3− in A549 cells (reproduced from ref. 116 with permission from Elsevier, copyright 2015). | |
The coumarin derivative SPH, containing an unsaturated ketone moiety, was designed as a bisulfite-selective ratiometric fluorescent probe (Fig. 71).117 The fluorescence of SPH (20 mM PBS, 1 mM CTAB, pH 7.4) shifts from 630 nm to 488 nm when bisulfite is added. The blue-shift is caused by the disruption of the π-conjugated system by 1,4-addition of bisulfite to the probe. The detection limit of SPH for bisulfite is 0.23 μM with a linear bisulfite concentration range of 0–80 μM. The probe was applied to monitor HSO3− supplemented in HeLa cells.
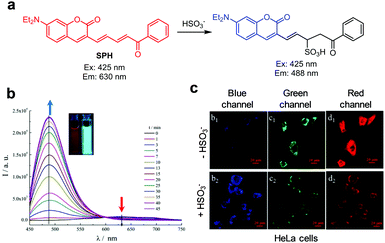 |
| Fig. 71 (a) A proposed mechanism for sensing HSO3− by SPH; (b) HSO3− concentration-dependent fluorescence changes of SPH (Ex: 425 nm); (c) its application for detection of HSO3− in HeLa cells (reproduced from ref. 117 with permission from Elsevier, copyright 2017). | |
Probe DSPT (DMSO/PBS = 1
:
1, pH 7.4), which is composed of dansyl and benzothiazolium moieties linked via a piperazine bridge, emits at 590 nm upon excitation at 390 nm arising from FRET from dansyl to benzothiazolium groups (Fig. 72).118 However, addition of bisulfite to the probe results in a blue-shift of the fluorescence to 540 nm. The detection limit of DSPT for bisulfite is 69 nM. Probe DSPT was applied to detect bisulfite in tap water and dry white wine. This probe was further utilized to image exogenous bisulfite in mitochondria of HeLa cells and endogenous bisulfite, generated by using glutathione (GSH) and Na2S2O3, in HepG2 cells.
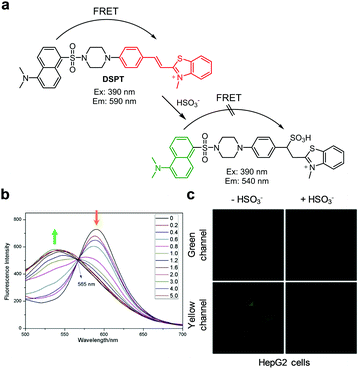 |
| Fig. 72 (a) A proposed mechanism for sensing HSO3− by DSPT; (b) HSO3− concentration-dependent fluorescence changes of DSPT (Ex: 390 nm); (c) its application for detection of HSO3− in HepG2 cells (reproduced from ref. 118 with permission from Elsevier, copyright 2017). | |
The benzocoumarin derivative P1, containing an aldehyde group, was devised as a two-photon ratiometric fluorescent probe for bisulfite (Fig. 73).119 Probe P1 (PBS, 1% DMSO, pH 7.4) is composed of a pyrrolidine moiety serving as the electron-donating group and formyl and lactone moieties as electron-withdrawing groups. When bisulfite is added to the aldehyde group in the probe, a blue-shift of the fluorescence from 635 nm to 562 nm takes place with one-photon excitation at 460 nm or two-photon excitation at 950 nm. The detection limit of P1 for bisulfite is 0.08 μM. Probe P1 was utilized to detect endogenous bisulfite in HeLa cells by using one-photon microscopy (excitation at 458 nm) and two-photon microscopy (950 nm).
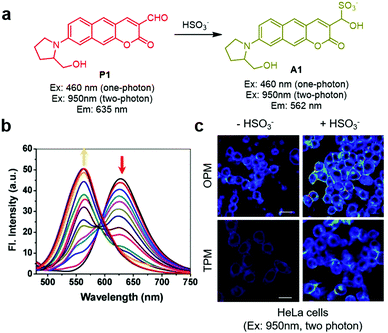 |
| Fig. 73 (a) A proposed mechanism for sensing HSO3− by P1; (b) HSO3− concentration-dependent fluorescence change of P1 (Ex: 460 nm); (c) its application for detection of HSO3− in HeLa cells (reproduced from ref. 119 with permission from Elsevier, copyright 2018). | |
The pyrene-based ratiometric fluorescent probe 19 bearing a 4,5-dibromothiophene moiety exhibits a ratiometric fluorescence response to bisulfite (Fig. 74).120 In this probe, the pyrene moiety acts as an electron donor and a thiophene moiety is the electron acceptor. Addition of bisulfite to the double bond of the probe (H2O/EtOH = 9
:
1) results in a blue-shift of the fluorescence from 530 nm to 420 nm upon excitation at 366 nm. A linear relationship exists between the magnitude of the emission change and bisulfite concentration in the 0–35 μM range, and the probe has a detection limit of 0.77 μM. Probe 19 was applied to image HSO3− in A549 cells, C. elegans and zebrafish.
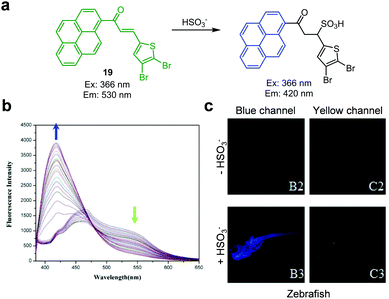 |
| Fig. 74 (a) A proposed mechanism for sensing HSO3− by 19; (b) HSO3− concentration-dependent fluorescence changes of 19 (Ex: 366 nm); (c) its application for detection of HSO3− in zebrafish (reproduced from ref. 120 with permission from Elsevier, copyright 2018). | |
Liu et al. designed and prepared the BODIPY-based, ratiometric fluorescent probe BSP1 for selective detection of sulfite (Fig. 75).121 Upon addition of sulfite to the probe (20 mM PBS, pH 7.4), the fluorescence band at 554 nm increases with a simultaneous decrease in the original band at 618 nm (excitation at 512 nm). The large blue-shift of emission is attributed to the addition of sulfite to the conjugated double bond of the probe. The detection limit of BSP1 for sulfite is 6.4 μM and the fluorescence changes linearly correlate with sulfite concentration in the range of 0–300 μM. BSP1 was applied to sense sulfite added in HeLa cells.
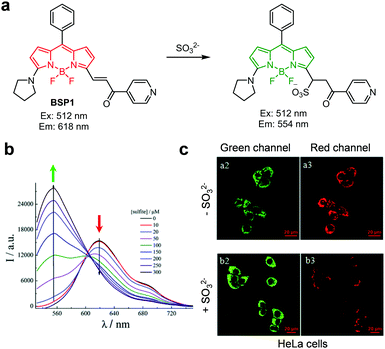 |
| Fig. 75 (a) A proposed mechanism for sensing SO32− by BSP1; (b) SO32− concentration-dependent fluorescence changes of BSP1 (Ex: 512 nm); (c) its application for detection of SO32− in HeLa cells (reproduced from ref. 121 with permission from the Royal Society of Chemistry, copyright 2015). | |
Feng's group developed the 2-(2′-hydroxyphenyl)benzothiazole (HBT)-hemicyanine derivative HH as a bisulfite-selective ratiometric fluorescent probe (Fig. 76).122 Addition of HSO3− to the probe (20 mM PBS/DMSO = 1
:
1, pH 7.4) induces a large blue-shift of the fluorescence maximum from 610 nm to 485 nm when excited at 400 nm. This shift is attributed to the addition of bisulfite to the conjugated double bond and interruption of the ESIPT process. The emission response linearly correlates with bisulfite concentration in the range of 0–25 μM with a detection limit of 56 nM. This probe was employed to detect HSO3− in a food sample and HeLa cells.
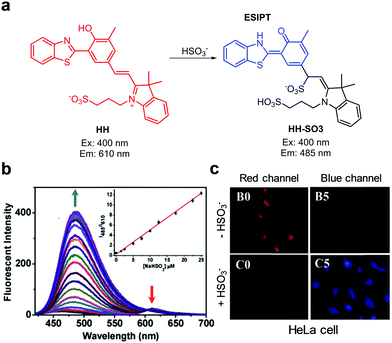 |
| Fig. 76 (a) A proposed mechanism for sensing HSO3− by HH; (b) HSO3− concentration-dependent fluorescence changes of HH (Ex: 400 nm); (c) its application for detection of HSO3− in HeLa cells (reproduced from ref. 122 with permission from Elsevier, copyright 2016). | |
The ICT- and FRET-based ratiometric fluorescent probe ZCn-hy, which consists of a coumarin–hemicyanine hybrid, was designed and prepared for detection of sulfite (Fig. 77).123 The intact probe (10 mM PBS, pH 7.4) displays fluorescence at 589 nm (excitation at 430 nm) through FRET from the coumarin to the hemicyanine dye. However, upon addition of sulfite to the probe, the intensity of fluorescence from the hemicyanine dye decreases as a consequence of blocking the FRET process. As a result, the emission maximum changes from 589 to 476 nm. Endogenous sulfite, generated by using GSH with Na2S2O3, was detected mainly in mitochondria of HepG2 and L-02 cells.
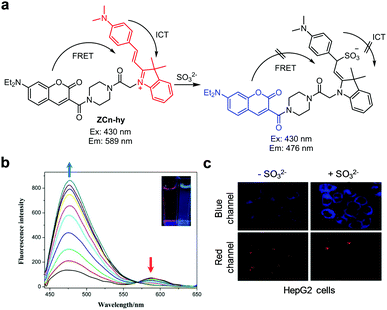 |
| Fig. 77 (a) A proposed mechanism for sensing SO32− by ZCn-hy; (b) SO32− concentration-dependent fluorescence changes of ZCn-hy (Ex: 430 nm); (c) its application for detection of SO32− in HeLa cells (reproduced from ref. 123 with permission from Elsevier, copyright 2017). | |
3.2 Detection of cyanide
Salts containing cyanide anions (CN−) are among the most dangerous toxins to living organisms. The cyanide anion acts as an inhibitor of mitochondrial cytochrome c oxidase and thus it disrupts the electron transport chain leading to eventual blockage of cellular respiration.124 It is also known that the cyanide anion is associated with the production of ROS such as hydroxyl radicals in mitochondria, which leads to oxidative damage.125 Although the cyanide anion results in severe health problems because of its extreme toxicity, it is still widely used in many industrial processes such as those used for polymer production, electroplating and gold mining.126
The Wang and Zhang groups created the ICT-based ratiometric fluorescent probe BCB for detection of CN− (Fig. 78).127 Excitation of the probe (DMSO/H2O = 1
:
9) at 330 nm leads to emission at 589 nm. However, addition of CN− to the probe leads to a blue-shift of the fluorescence from 589 nm to 424 nm. This change is attributed to the disruption of the ICT process by addition of CN− to an iminium moiety and consequent interruption of the conjugated electron network in the probe. The detection limit of BCB for CN− is 0.09 μM and it has good selectivity toward CN− over other anions. BCB was applied to detect CN− exogenously added in HeLa cells.
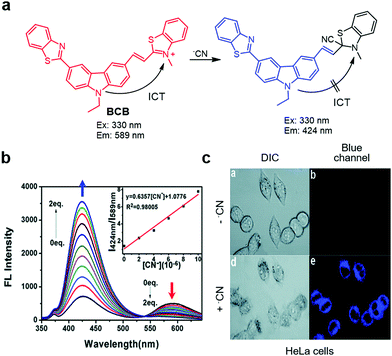 |
| Fig. 78 (a) A proposed mechanism for sensing CN− by BCB; (b) CN− concentration-dependent fluorescence changes of BCB (Ex: 330 nm); (c) its application for detection of CN− in HeLa cells (reproduced from ref. 127 with permission from the Royal Society of Chemistry, copyright 2016). | |
The ratiometric fluorescent probe 20, which is similar to BCB, was developed for detection of cyanide by Yin and coworkers (Fig. 79).128 The intact probe (MeCN/PBS = 1
:
1) displays emission at 653 nm with excitation at 510 nm. Addition of CN− leads to a blue-shift in the fluorescence maximum from 653 nm to 542 nm owing to the disruption of the ICT process by CN− addition to an iminium moiety in the probe. The detection limit of the probe for cyanide is 2.41 μM and 20 shows selectivity toward CN− over other anions. The probe was used to detect CN− supplemented in HepG2 cells.
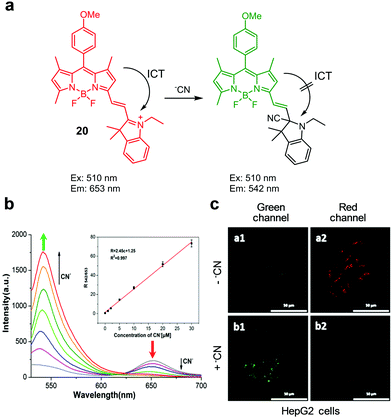 |
| Fig. 79 (a) A mechanism for sensing CN− by 20; (b) CN− concentration-dependent fluorescence changes of 20 (Ex: 510 nm); (c) its application for detection of CN− in HepG2 cells (reproduced from ref. 128 with permission from Elsevier, copyright 2019). | |
Li and coworkers prepared the ratiometric fluorescent probe 21, which contains a conjugated π-electron system that displays differential fluorescence responses to cyanide and sulfide (Fig. 80).129 The free probe (10 mM PBS/DMSO = 1
:
9) exhibits emission at 678 nm when excited at 470 nm. In the presence of CN−, the fluorescence maximum blue-shifts from 678 nm to 575 nm. On the other hand, addition of S2− to the probe causes a shift of the emission from 678 nm to 500 nm. The differential fluorescence response of the probe is attributed to addition of CN− and S2− to different positions of the probe. The probe shows selectivity toward CN− and S2− over other anions, and it was employed for imaging CN− and S2− supplemented in HeLa cells at different channels.
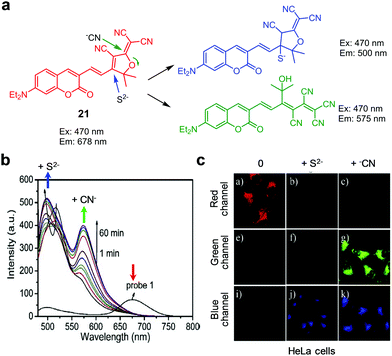 |
| Fig. 80 (a) Proposed mechanisms for sensing CN− and S2− by 21; (b) CN− and S2− concentration-dependent fluorescence changes of 21 (Ex: 470 nm); (c) its application for detection of CN− and S2− in HeLa cells (reproduced from ref. 129 with permission from Elsevier, copyright 2017). | |
Yin and coworkers synthesized the ESIPT-based, ratiometric fluorescent probe EP1 for CN− (Fig. 81).130 Intact EP1 (100% EtOH) exhibits emission at 616 nm upon excitation at 480 nm. Addition of CN− to the probe leads to a decrease in the intensity of the band at 616 nm and a concomitant increase in fluorescence at 657 nm. This phenomenon is attributed to the ESIPT mechanism caused by the intramolecular hydrogen bonding of the cyanohydrin oxygen with the phenolic OH groups in the product formed by cyanide addition to the carbonyl group in EP1. This probe displays high selectivity toward cyanide over other anions and has a detection limit of 270 nM for CN−. EP1 was utilized to detect exogenous CN− in A549 cells.
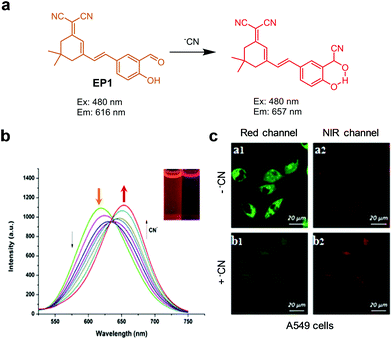 |
| Fig. 81 (a) A mechanism for sensing CN− by EP1; (b) CN− concentration-dependent fluorescence changes of EP1 (Ex: 480 nm); (c) its application for detection of CN− in A549 cells (reproduced from ref. 130 with permission from Elsevier, copyright 2017). | |
Chattopadhyay and coworkers synthesized a chromone-based fluorescent probe, DBTC, for ratiometric detection of CN− (Fig. 82).131 The free probe (20 mM HEPES/DMSO = 3
:
1, pH 7.4) exhibits emission at 540 nm upon excitation at 370 nm. However, when CN− is added, the intensity of the emission band at 540 nm gradually decreases with a simultaneous increase in a fluorescence band at 430 nm. This change is attributed to the nucleophilic addition of CN− to the electrophilic C-9 position and subsequent dimerization of the resultant product. DBTC responds selectively to CN− but not to other anions and has a detection limit of 5.76 nM. DBTC was applied for detecting CN− supplemented in MDA cells.
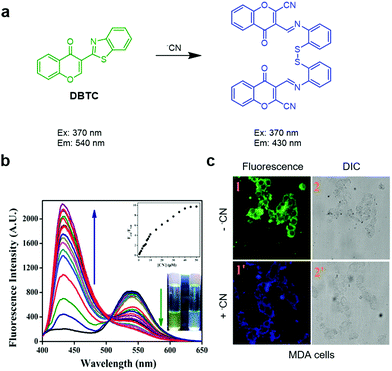 |
| Fig. 82 (a) A mechanism for sensing CN− by DBTC; (b) CN− concentration-dependent fluorescence changes of DBTC (Ex: 370 nm); (c) its application for detection of CN− in MDA cells (reproduced from ref. 131 with permission from the American Chemical Society, copyright 2018). | |
3.3 Detection of phosphate and pyrophosphate
Phosphate (Pi) and phosphate containing species play key roles in various biological processes.132 However, it is very challenging to selectively recognize and/or sense phosphates in aqueous solutions.133 Zhang et al. developed the ratiometric fluorescent probe 22 for detection of phosphate (Fig. 83).134 The intact probe (20 mM DMSO/HEPES = 7
:
3, pH 7.4) displays fluorescence at 453 nm with excitation at 380 nm. However, addition of phosphate results in the formation of a new emission band at 532 nm with a decrease in emission at 453 nm. This fluorescence change is attributed to an enhancement of the internal charge transfer property caused by removal of the oxalate group from the probe by phosphate. Probe 22 responds selectively to phosphate. It was applied to sense exogenous and endogenous phosphate, generated in situ using ATP and apyrase, in CHO cells and C. elegans. In addition, phosphate ions generated in hemichannel-closed Sf9 cells were also monitored using this probe.
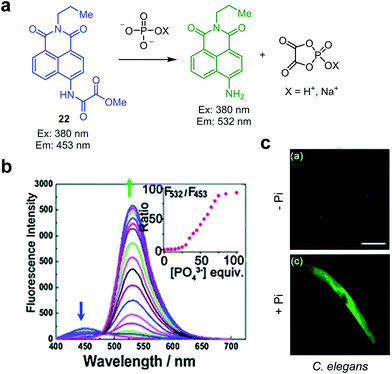 |
| Fig. 83 (a) A proposed mechanism for sensing phosphate by 22; (b) phosphate concentration-dependent fluorescence changes of 22 (Ex: 380 nm); (c) its application for detection of phosphate in C. elegans nematodes (reproduced from ref. 134 with permission from Wiley-VCH, copyright 2015). | |
Jiao et al. devised the ratiometric fluorescent probe L1, which is composed of an anthracene fluorophore and terpyridine serving as a binding receptor for Cd2+ (Fig. 84).135L1 (10 mM CH3CN/HEPES = 1
:
1, pH 7.4) displays fluorescence at 431 nm with excitation at 326 nm. Addition of Zn2+ or Cd2+ to the probe leads to the formation of a new fluorescence maximum at 560 nm and a decrease in the intensity of the emission band at 431 nm. Analysis of X-ray crystal structures indicates the formation of a 2
:
2 complex of L1 with Cd2+. Upon addition of pyrophosphate (PPi) to the L1–Cd2+ complex, the intensity of the fluorescence band at 431 nm increases with a simultaneous decrease in emission at 560 nm (excitation at 326 nm). L1–Cd2+ responds selectively to pyrophosphate with a detection limit of 3.39 μM. The displacement approach was further applied to sense pyrophosphate in RAW264.7 cells.
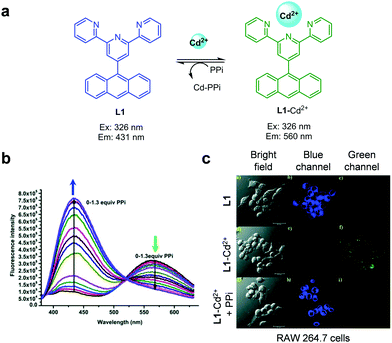 |
| Fig. 84 (a) Structure and formation of L1–Cd2+; (b) pyrophosphate concentration-dependent fluorescence changes of L1–Cd2+ (Ex: 326 nm); (c) its application for detection of pyrophosphate in RAW 264.7 cells (reproduced from ref. 135 with permission from the Royal Society of Chemistry, copyright 2015). | |
3.4 Detection of fluoride
The fluoride anion is widely used as an additive to toothpaste and is an essential ingredient in pharmaceuticals.136 Overexposure to fluoride or intake of excess fluoride is known to cause urinary stones as well as stomach and kidney disorders.137,138 The World Health Organization (WHO) has recommended that the allowable daily intake of fluoride should be less than 10 mg,139 and the US Environmental Protection Agency (USEPA) has set the concentration limit for fluoride in drinking water to be 4 ppm (211 μM).140 As a result, it is important to develop efficient methods for sensitive and accurate detection of fluoride in biological systems.141
Most of the ratiometric fluorescent probes for fluoride have been designed based on Si–O bond cleavage processes. For instance, Tang and Wu described ratiometric fluorescent probes, Mito-F and Lyso-F, which contain triphenylphosphonium and dimethylamino moieties for targeting mitochondria and lysosomes, respectively (Fig. 85).142 In the presence of fluoride (20 mM HEPES/DMSO = 1
:
1, pH 7.4), t-butyldimethylsilyl (TBS) groups are removed from Mito-F or Lyso-F to generate the corresponding N-substituted 4-aminonaphthalimide via self-immolative cleavage. This process leads to a decrease in the fluorescence band at 475 nm and an increase in a band at 540 nm (excitation at 405 nm). The detection limits of Mito-F and Lyso-F for fluoride are 0.067 ppm and 0.073 ppm, respectively. Both probes exhibit high selectivity toward F− among other anions. Mito-F and Lyso-F were employed to image fluoride in mitochondria and lysosomes of HeLa cells, respectively. In addition, Mito-F was also applied to detect fluoride in mice.
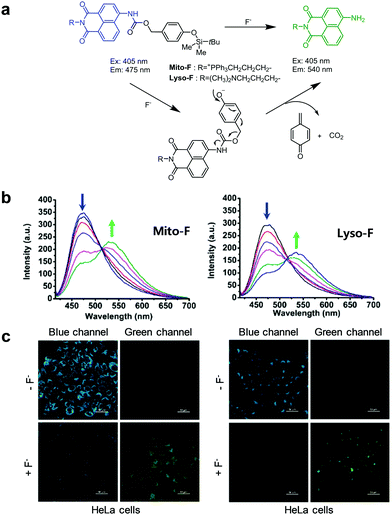 |
| Fig. 85 (a) Proposed mechanisms for sensing F− by Mito-F and Lyso-F; (b) F− concentration-dependent fluorescence changes of Mito-F and Lyso-F (Ex: 405 nm); (c) their applications for detection of F− in HeLa cells (reproduced from ref. 142 with permission from the American Chemical Society, copyright 2015). | |
Li and colleagues devised the two-photon ratiometric fluorescent probe QF for effective detection of fluoride (Fig. 86).143 Addition of fluoride to the probe (50 mM PBS, 25 μM CTAB, pH 7.4) leads to a decrease in the fluorescence band at 404 nm and an increase in emission at 533 nm with two-photon excitation at 730 nm or one-photon excitation at 366 nm. This fluorescence change is a consequence of fluoride-promoted cleavage of the t-butyldiphenylsilyl (TBDPS) group from QF to generate a free phenolic OH group, which is in equilibrium with the corresponding phenolate anion at neutral pH. Changes in the ratio of fluorescence intensities at 533 nm and 404 nm (F533nm/F404nm) show an excellent linear relationship with the concentration of fluoride, and the detection limit of QF for fluoride is 0.5 μM. This probe was used for two-photon fluorescence imaging of fluoride in HeLa cells and zebrafish.
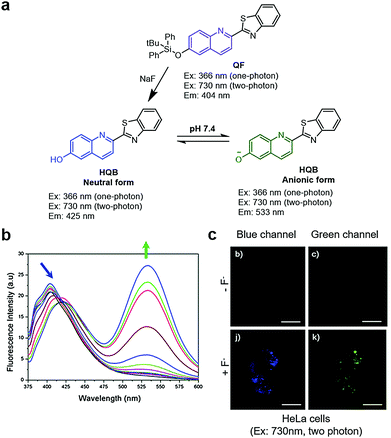 |
| Fig. 86 (a) A proposed mechanism for sensing F− by QF; (b) F− concentration-dependent fluorescence changes of QF (Ex: 366 nm); (c) its application for detection of F− in HeLa cells (reproduced from ref. 143 with permission from the Royal Society of Chemistry, copyright 2016). | |
Zhang and colleagues reported the ratiometric fluorescent fluoride probe Z2, which is designed based on the operation of the ICT mechanism (Fig. 87).144 When the TBDPS group in Z2 is removed by fluoride (20 mM HEPES/DMSO = 7
:
3, pH 7.4), the fluorescence emission at 440 nm is reduced and an increase in emission at 540 nm occurs upon one-photon excitation at 365 nm or two-photon excitation at 780 nm. Z2 was applied to image fluoride in HeLa cells and tumor tissues.
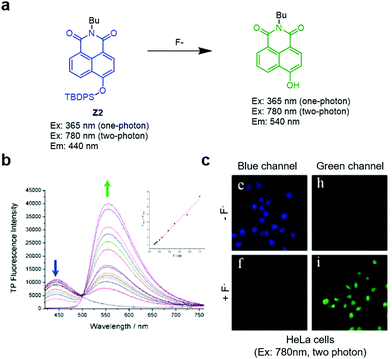 |
| Fig. 87 (a) A proposed mechanism for sensing F− by Z2; (b) F− concentration-dependent fluorescence changes of Z2 (Ex: 365 nm); (c) its application for detection of F− in HeLa cells (reproduced from ref. 144 with permission from MDPI, copyright 2015). | |
Chen and his colleagues designed the ratiometric fluorescent probe 23 that has the ability to detect fluoride in mitochondria (Fig. 88).145 The pyridinium cation is introduced to the probe for targeting mitochondria. When probe 23 (50 mM PBS/DMF = 7
:
3, pH 7.4) is treated with fluoride, its fluorescence at 539 nm increases with a concomitant decrease in the emission band at 639 nm. The detection limit of 23 for fluoride is 1.2 × 10−8 M, indicating its high sensitivity. Probe 23 was applied for ratiometric fluorescence imaging of fluoride in mitochondria of HepG2 cells.
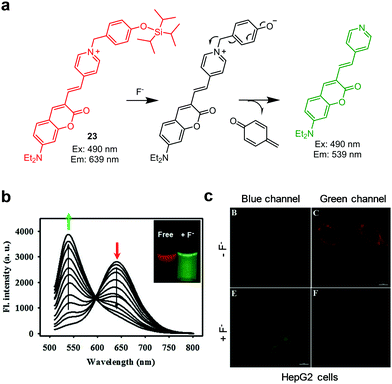 |
| Fig. 88 (a) A proposed mechanism for sensing F− by 23; (b) F− concentration-dependent fluorescence changes of 23 (Ex: 490 nm); (c) its application for detection of F− in HepG2 cells (reproduced from ref. 145 with permission from Elsevier, copyright 2018). | |
Yang and colleagues developed the ratiometric NIR fluorescent probe 24 for detection of fluoride (Fig. 89).146 In the presence of fluoride, the original emission band of the probe (10 mM PBS/DMSO = 2
:
8, pH 7.4) at 690 nm decreases with an increase in fluorescence intensity at 740 nm (excitation at 600 nm). Probe 24 displays a linear ratiometric fluorescence response (F740nm/F690nm) to fluoride concentration and it has a low detection limit of 0.2 μm. Probe 24 was used to quantitatively determine the amount of fluoride in drinking water and white flour. This probe was also employed for fluorescence imaging of fluoride in HepG2 cells and in mice.
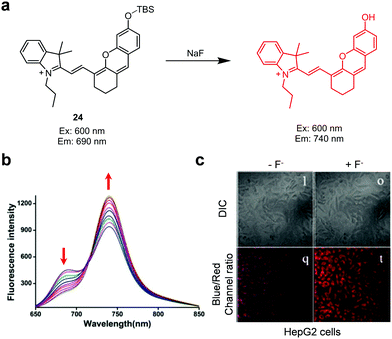 |
| Fig. 89 (a) A proposed mechanism for sensing F− by probe 24; (b) F− concentration-dependent fluorescence change of probe 24 (Ex: 600 nm); (c) its application for detection of F− in HepG2 cells (reproduced from ref. 146 with permission from the American Chemical Society, copyright 2018). | |
Fang and coworkers reported the flavone-based ratiometric fluorescent fluoride probe 25 (Fig. 90).147 Addition of fluoride to 25 (CH3CN/H2O = 95
:
5) results in a reduction of the fluorescence at 400 nm and an increase in an emission band at 530 nm with excitation at 350 nm. The change is a consequence of fluoride-promoted Si–O bond cleavage that generates hydroxyflavone in which strong ESIPT occurs in association with a large Stokes shift. Probe 25 displays a fast response to fluoride and its detection limit is 0.68 μM. Probe 25 was utilized to monitor exogenous fluoride in HGC-27 cells.
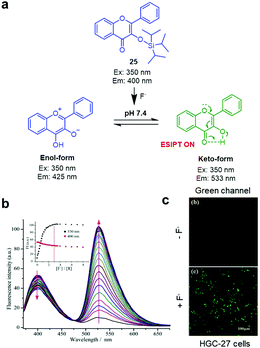 |
| Fig. 90 (a) A proposed mechanism for sensing F− by probe 25; (b) F− concentration-dependent fluorescence changes of probe 25 (Ex: 350 nm); (c) its application for detection of F− in HGC-27 cells (reproduced from ref. 147 with permission from Elsevier, copyright 2019). | |
3.5 Detection of periodate
Periodate (IO4−) is a powerful oxidizing agent and is used in industrial, food and medical care applications. This oxidant is also widely used in organic chemistry to prepare aldehydes and ketones from 1,2-diols. The Huang and Jia groups developed the ESIPT-based fluorescent probe PDS-2 to ratiometrically detect periodate (Fig. 91).148 This probe is composed of a vicinal diol group as a reactive site for periodate and an ESIPT fluorophore. The fluorescence spectrum of PDS-2 in PBS changes as the concentration of added periodate increases. Specifically, upon addition of periodate, a decrease in emission at 406 nm occurs with an increase in fluorescence at 462 nm. This response is almost fully completed within 15 min and the detection limit of PDS-2 for periodate is 26 μM. PDS-2 shows high selectivity toward periodate without interference from other analytes, such as sodium borate, sodium fluoride, sodium citrate, potassium nitrate, sodium oxalate, sodium hypochlorite, H2O2, sodium iodide and sodium iodate. PDS-2 was used to image periodate supplemented in HeLa cells.
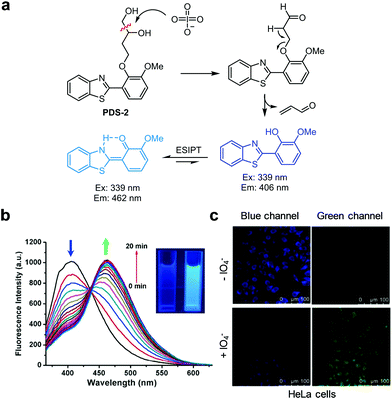 |
| Fig. 91 (a) A proposed mechanism for sensing IO4− by PDS-2; (b) IO4− concentration-dependent fluorescence changes of PDS-2 (Ex: 339 nm); (c) its application for detection of IO4− in HeLa cells (reproduced from ref. 148 with permission from Elsevier, copyright 2015). | |
3.6 Detection of selenite
Sodium selenite (Na2SeO3), one common selenium containing substance, is used for cancer treatment. Monitoring dynamic changes in the concentration of SeO32− in cells is highly challenging.149,150 Recently, the ESIPT-based ratiometric fluorescent probe HBTN-Se was developed for selective detection of selenite (Fig. 92).151 This probe is composed of a 1-hydroxy-2-(benzothiazol-2-yl)naphthalene (HBTN) group serving as an ESIPT moiety and a levulinyl group as the reactive site for selenite. Intact HBTN-Se (20 mM PBS/EtOH = 8
:
2, pH 7.4) displays enol-like blue emission at 410 nm when excited at 330 nm. Addition of selenite to the probe promotes removal of the levulinyl group to form a free phenol group. This event leads to keto green emission at 475 nm in association with a decrease in emission at 410 nm. HBTN-Se shows high selectivity toward selenite over other anions and seleno/sulfur species. The detection limit of this probe for selenite is 0.13 μM and a linear response to selenite occurs in the 0–25 μM range. HBTN-Se was applied to image selenite in HepG2 and L-02 cells.
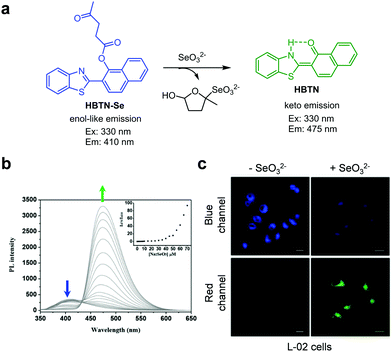 |
| Fig. 92 (a) A proposed mechanism for sensing SeO32− by HBTN-Se; (b) SeO32− concentration-dependent fluorescence change of HBTN-Se (Ex: 330 nm); (c) its application for detection of SeO32− in L-02 cells (reproduced from ref. 151 with permission from Elsevier, copyright 2019). | |
4. Conclusions
Owing to their advantageous features, ratiometric fluorescent probes can be used for quantitative detection of various analytes. In this review article, we highlighted advances made in the development of organic dye-based, ratiometric fluorescent probes for metal cations and anions reported since 2015. Specifically, we described synthetic ratiometric fluorescent probes that are utilized to detect and image a variety of ions in the biological systems. In addition, we illustrated the strategies used to design these probes and sensing mechanisms for their response to specific analytes and pointed their applications to study important biological systems. Although challenging, advance in this research field will be enhanced by interdisciplinary collaborations between chemists and biologists.
Great opportunities and challenges still remain in the development of synthetic ratiometric fluorescent probes that can be used for quantitative analysis of ions in biological systems. In particular, organic dye-based, ratiometric fluorescent probes for more biologically important ions, such as Na+, K+, Ca2+ and Cl−, as well as cellular organelle-targeting ratiometric fluorescent probes need to be developed to understand the functions of cellular ions. Clearly, the availability of versatile probes of this type will be the impetus behind studies targeted at gaining an understanding of effects of cellular ions on biological processes.
Conflicts of interest
There are no conflicts to declare.
Acknowledgements
This work was supported financially by the National Research Foundation of Korea (grant no. 2019R1H1A3037339 to I. S. and 2012R1A3A2048814 to J. Y.).
Notes and references
- D. Wu, A. C. Sedgwick, T. Gunnlaugsson, E. U. Akkaya, J. Yoon and T. D. James, Chem. Soc. Rev., 2017, 46, 7105–7123 RSC.
- J. Chan, S. C. Dodani and C. J. Chang, Nat. Chem., 2012, 4, 973–984 CrossRef CAS.
- Z. Guo, S. Park, J. Yoon and I. Shin, Chem. Soc. Rev., 2014, 43, 16–29 RSC.
- L. You, D. Zha and E. V. Anslyn, Chem. Rev., 2015, 115, 7840–7892 CrossRef CAS.
- H. Zhu, J. Fan, B. Wang and X. Peng, Chem. Soc. Rev., 2015, 44, 4337–4366 RSC.
- Z. Yang, A. Sharma, J. Qi, X. Peng, D. Y. Lee, R. Hu, D. Lin, J. Qu and J. S. Kim, Chem. Soc. Rev., 2016, 45, 4651–4667 RSC.
- J. Li, D. Yim, W.-D. Jang and J. Yoon, Chem. Soc. Rev., 2017, 46, 2437–2458 RSC.
- S. Singha, D. Kim, H. Seo, S. W. Cho and K. H. Ahn, Chem. Soc. Rev., 2015, 44, 4367–4399 RSC.
- J. Yan, S. Lee, A. Zhang and J. Yoon, Chem. Soc. Rev., 2018, 47, 6900–6916 RSC.
- G. Rijun, J. Hui, B. Xiangning, F. Yongxin, W. Zonghua and L. Qingyun, Coord. Chem. Rev., 2019, 383, 82–103 CrossRef.
- M. H. Lee, J. S. Kim and J. L. Sessler, Chem. Soc. Rev., 2015, 44, 4185–4191 RSC.
- Y. Chen, Y. Bai, Z. Han, W. He and Z. Guo, Chem. Soc. Rev., 2015, 44, 4517–4546 RSC.
- J. A. Cotruvo Jr., A. T. Aron, K. M. Ramos-Torresa and C. J. Chang, Chem. Soc. Rev., 2015, 44, 4400–4414 RSC.
- J. Yin, Y. Hu and J. Yoon, Chem. Soc. Rev., 2015, 44, 4619–4644 RSC.
- G. T. Sfrazzetto, C. Satriano, G. A. Tomaselli and E. Rizzarelli, Coord. Chem. Rev., 2016, 311, 125–167 CrossRef.
- D. Wu, L. Chen, W. Lee, G. Ko, J. Yin and J. Yoon, Coord. Chem. Rev., 2018, 354, 74–97 CrossRef CAS.
- X.-P. He, X.-L. Hu, T. D. James, J. Yoon and H. Tian, Chem. Soc. Rev., 2017, 46, 6687–6696 RSC.
- P. A. Gale and C. Caltagirone, Coord. Chem. Rev., 2018, 354, 2–27 CrossRef CAS.
- R. Martínez-Máñez and F. Sancanón, Chem. Rev., 2003, 103, 4419–4476 CrossRef PubMed.
- Z. Xu, S. K. Kim and J. Yoon, Chem. Soc. Rev., 2010, 39, 1457–1466 RSC.
- A. P. de Silva, H. Q. N. Gunaratne, T. Gunnlaugsson, A. J. M. Huxley, C. P. McCoy, J. T. Rademacher and T. E. Rice, Chem. Rev., 1997, 97, 1515–1566 CrossRef CAS.
- A. C. Sedgwick, L. Wu, H.-H. Han, S. D. Bull, X.-P. He, T. D. James, J. L. Sessler, B. Z. Tang, H. Tian and J. Yoon, Chem. Soc. Rev., 2018, 47, 8842–8880 RSC.
- P. Thrumbo, A. A. Yates, S. Schlicker and M. Poos, J. Am. Diet. Assoc., 2001, 101, 294–301 CrossRef.
- J. Tang, S. Ma, D. Zhang, Y. Liu, Y. Zhao and Y. Ye, Sens. Actuators, B, 2016, 236, 109–115 CrossRef CAS.
- V. Dujols, F. Ford and A. W. Czarnik, J. Am. Chem. Soc., 1997, 119, 7386–7387 CrossRef CAS.
- Y. Ge, X. Zheng, R. Ji, S. Shen and X. Cao, Anal. Chim. Acta, 2017, 965, 103–110 CrossRef CAS.
- X. Zheng, R. Ji, X. Cao and Y. Ge, Anal. Chim. Acta, 2017, 978, 48–54 CrossRef CAS.
- C. Liu, X. Jiao, S. He, L. Zhao and X. Zeng, Org. Biomol. Chem., 2017, 15, 3947–3954 RSC.
- W. N. Wu, H. Wu, R. B. Zhong, Y. Wang, Z. H. Xu, X. L. Zhao, Z. Q. Xu and Y. C. Fan, Spectrochim. Acta, Part A, 2019, 212, 121–127 CrossRef CAS.
- S. Y. Park, W. Kim, S. H. Park, J. Han, J. Lee, C. Kang and M. H. Lee, Chem. Commun., 2017, 53, 4457–4460 RSC.
- Y. Wang, H. Wu, W. N. Wu, Y. P. Yu, X. L. Zhao, Z. H. Xu, Z. Q. Xu and Y. C. Fan, J. Lumin., 2018, 204, 289–295 CrossRef CAS.
- P. K. Mehta, E. T. Oh, H. J. Park and K. H. Lee, Sens. Actuators, B, 2018, 256, 393–401 CrossRef CAS.
- B. Szewczyk, Front. Aging Neurosci., 2013, 5(33), 1–12 Search PubMed.
- K. Thambiayya, K. Wasserloos, V. E. Kagan, D. Stoyanovsky and B. R. Pitt, Am. J. Physiol.: Lung Cell. Mol. Physiol., 2012, 302, L1287–L1295 CrossRef CAS.
- Z. Xu, J. Yoon and D. R. Spring, Chem. Soc. Rev., 2010, 39, 1996–2006 RSC.
- D. Y. Zhang, M. Azrad, W. Demark-Wahnefried, C. J. Frederickson, S. J. Lippard and R. J. Radford, ACS Chem. Biol., 2015, 10, 385–389 CrossRef CAS.
- D. Bourassa, C. M. Elitt, A. M. McCallum, S. Sumalekshmy, R. L. McRae, M. T. Morgan, N. Siegel, J. W. Perry, P. A. Rosengerg and C. J. Fahrni, ACS Sens., 2018, 3, 458–467 CrossRef CAS.
- H. Santhakumar, R. V. Nair, D. S. Philips, S. J. Shenoy, A. Thekkuveettil, A. Ajayaghosh and R. S. Jayasree, Sci. Rep., 2018, 8, 9069 CrossRef.
- S. Sinha, P. Gaur, T. Mukherjee, S. Mukhopadhyay and S. Ghosh, J. Photochem. Photobiol., B, 2015, 148, 181–187 CrossRef CAS.
- P. K. Mehta, E. T. Oh, H. J. Park and K. H. Lee, Sens. Actuators, B, 2017, 245, 996–1003 CrossRef CAS.
- K. Dui, S. Niu, L. Qiao, Y. Dou, Q. Zhu, X. Chen and P. Zhang, RSC Adv., 2017, 7, 40615–40620 RSC.
- T. W. Price, G. Firth, C. J. Eling, M. Kinnon, N. J. Long, J. Sturge and G. J. Stasiuk, Chem. Commun., 2018, 54, 3227–3230 RSC.
- W. Li, B. Fang, M. Jin and Y. Tian, Anal. Chem., 2017, 89, 2553–2560 CrossRef CAS PubMed.
- H. Mehdi, W. Gong, H. Guo, M. Watkinson, H. Ma, A. Wajahat and G. Ning, Chemistry, 2017, 23, 13067–13075 CrossRef CAS.
- S. Hussain, A. Atkinson, S. Thompson and A. Khan, J. Environ. Sci. Health, Part B, 1999, 34, 645–660 CrossRef CAS.
- H. Liu, H. Ding, L. Zhu, Y. Wang, Z. Chen and Z. Tian, J. Fluoresc., 2015, 25, 1259–1266 CrossRef CAS PubMed.
- B. Zhang, P. Ma, D. Gao, X. Wang, Y. Sun, D. Song and X. Li, Spectrochim. Acta, Part A, 2016, 165, 99–105 CrossRef CAS.
- X. Yang, X. Qin, Y. Li, M. Yan, Y. Cui and G. Sun, Biosens. Bioelectron., 2018, 121, 62–71 CrossRef CAS.
- S. Ko, Y. Yang, J. Tae and I. Shin, J. Am. Chem. Soc., 2006, 128, 14150–14155 CrossRef CAS PubMed.
- M. Wang, J. Wen, Z. Qin and H. Wang, Dyes Pigm., 2015, 120, 208–212 CrossRef CAS.
- Y. Ge, X. Xing, A. Liu, R. Ji, S. Shen and X. Cao, Dyes Pigm., 2017, 146, 136–142 CrossRef CAS.
- R. Ji, A. Liu, S. Shen, X. Cao, F. Li and Y. Ge, RSC Adv., 2017, 7, 40829–40833 RSC.
- Y. Ge, A. Liu, R. Ji, S. Shen and X. Cao, Sens. Actuators, B, 2017, 251, 410–415 CrossRef CAS.
- Y. Chen, W. Zhang, Y. Cai, R. Kwok, Y. Hu, J. Lam, X. Gu, Z. He, Z. Zhao, X. Zheng, B. Chen, C. Gui and B. Tang, Chem. Sci., 2017, 8, 2047–2055 RSC.
- S. Bi, G. Zhang, Y. Wu, S. Wu and L. Wang, Dyes Pigm., 2016, 134, 586–592 CrossRef CAS.
- S. Pan, K. Li, L. Li, M. Li, L. Shi, Y. Liu and X. Yu, Chem. Commun., 2018, 54, 4955–4958 RSC.
- S. Qin, B. Chen, J. Huang and Y. Han, New J. Chem., 2018, 42, 12766–12772 RSC.
- L. Chen, S. Park, D. Wu, H. Kim and J. Yoon, Chem. Commun., 2019, 55, 1766–1769 RSC.
- X. Jiao, C. Liu, S. He, L. Zhao and X. Zeng, Dyes Pigm., 2019, 160, 86–92 CrossRef CAS.
- Y. Zhang, H. Chen, D. Chen, D. Wu, Z. Chen, J. Zhang, X. Chen, S. Liu and J. Yin, Sens. Actuators, B, 2016, 224, 907–914 CrossRef CAS.
- H. Wang, P. Zhang, J. Chen, Y. Li, M. Yu, Y. Long and P. Yi, Sens. Actuators, B, 2017, 242, 818–824 CrossRef CAS.
- B. Gu, L. Huang, N. Mi, P. Yin, Y. Zhang, X. Tu, X. Luo, S. Luo and S. Yao, Analyst, 2015, 140, 2778–2784 RSC.
- Y. Wang, M. Gao, Q. Chen, F. Yu, G. Jiang and L. Chen, Anal. Chem., 2018, 90, 9769–9778 CrossRef CAS.
- W. Breuer, M. Shvartsman and Z. I. Cabantchik, Int. J. Biochem. Cell Biol., 2008, 40, 350–354 CrossRef CAS PubMed.
- A. Goel, S. Umar, P. Nag, A. Sharma, L. Kumar, Shamsuzzama, Z. Hossain, J. R. Gayen and A. Nazir, Chem. Commun., 2015, 51, 5001–5004 RSC.
- J. Geng, Y. Liu, J. Li, G. Yin, W. Huang, R. Wang and Y. Quan, Sens. Actuators, B, 2016, 222, 612–617 CrossRef CAS.
- A. T. Aron, M. O. Loehr, J. Bogena and C. J. Chang, J. Am. Chem. Soc., 2016, 138, 14338–14346 CrossRef CAS.
- Z. Liu, S. Wang, W. Li and Y. Tian, Anal. Chem., 2018, 90, 2816–2825 CrossRef CAS.
- J. Kielhorn, C. Melber, D. Keller and I. Mangelsdorf, Int. J. Hyg. Environ. Health, 2002, 205, 417–432 CrossRef CAS PubMed.
- X. Wu, H. Neumann and M. Beller, Chem. Soc. Rev., 2011, 40, 4986–5009 RSC.
- C. Garrett and K. Prasad, Adv. Synth. Catal., 2004, 346, 889–900 CrossRef CAS.
- L. Zhou, Q. Wang, X. Zhang and W. Tan, Anal. Chem., 2015, 87, 4503–4507 CrossRef CAS.
- L. Wang, M. Ren, Z. Li, L. Dai and W. Lin, New J. Chem., 2019, 43, 552–555 RSC.
- W. Feng, L. Bai, S. Jia and G. Feng, Sens. Actuators, B, 2018, 260, 554–562 CrossRef CAS.
- Z. Liyi, H. Shunqin, W. Haifei, S. Hongyan and X. Zhang, Spectrochim. Acta, Part A, 2016, 166, 25–30 CrossRef.
- W. Luo, J. Li and W. Liu, Org. Biomol. Chem., 2017, 15, 5846–5850 RSC.
- R. B. Martin, Clin. Chem., 1986, 10, 1797–1806 Search PubMed.
- H. Xie, Y. Wu, J. Huang, F. Zeng, H. Wu, X. Xia, C. Yu and S. Wu, Talanta, 2016, 151, 8–13 CrossRef CAS.
- D. Wang, X. Fan, S. Sun, S. Du, H. Li, J. Zhu, Y. Tang, M. Chang and Y. Xu, Sens. Actuators, B, 2018, 264, 304–311 CrossRef CAS.
- N. Busschaert, S. H. Park, K. H. Baek, Y. P. Choi, J. Park, E. N. W. Howe, J. R. Hiscock, L. E. Karagiannidis, I. Marques, V. Felix, W. Namkung, J. L. Sessler, P. A. Gale and I. Shin, Nat. Chem., 2017, 7, 667–675 CrossRef.
- H. R. Pohl, J. S. Wheeler and H. E. Murray, Met. Ions Life Sci., 2013, 13, 29–47 Search PubMed.
- M. Taki, H. Ogasawara, H. Osaki, A. Fukazawa, Y. Sato, K. Ogasawara, T. Higashiyama and S. Yamaguchi, Chem. Commun., 2015, 51, 11880–11883 RSC.
- W. Maret and B. L. Vallee, Methods Enzymol., 1993, 226, 52–71 CAS.
- S. Zhang, M. Zhao, W. Zhu, Y. Xu and X. Qian, Dalton Trans., 2015, 44, 9740–9743 RSC.
- M. P. Waalkes, Mutat. Res., 2003, 533, 107–120 CrossRef CAS PubMed.
- Z. G. Sun, Z. Li, D. D. Yuan, J. F. Gao, L. Lin, J. Lin, M. L. Zhu, J. A. Makawana, Y. Qian and H. L. Zhu, Chem. Pharm. Bull., 2016, 64, 27–33 CrossRef.
- K. Krishnaveni, M. Iniya, D. Jeyanthi, A. Siva and D. Chellappa, Spectrochim. Acta, Part A, 2018, 205, 557–567 CrossRef CAS.
- J. L. Barriada, A. D. Tappin, E. H. Evans and E. P. Achterberg, Trends Anal. Chem., 2007, 26, 809–817 CrossRef CAS.
- H. Y. Tang, Y. Gao, B. Li, C. W. Li and Y. Guo, Sens. Actuators, B, 2018, 270, 562–569 CrossRef CAS.
- F. Faridbod, M. R. Ganjiali, B. Larijini, M. Hosseini and P. Norouzi, Mater. Sci. Eng., C, 2010, 30, 555–560 CrossRef CAS.
- H. Zhang, T. Liu, C. Yin, Y. Wen, J. Chao, Y. Zhang and F. Huo, Spectrochim. Acta, Part A, 2017, 174, 230–235 CrossRef CAS.
- N. Krause and C. Winter, Chem. Rev., 2011, 111, 1994–2009 CrossRef CAS.
- C. M. Goodman, C. D. McCusker, T. Yilmaz and V. M. Rotello, Bioconjugate Chem., 2004, 15, 897–900 CrossRef CAS.
- A. Habib and M. Tabata, J. Inorg. Biochem., 2004, 98, 1696–1702 CrossRef CAS.
- J. F. Zhang, Y. Zhou, J. Yoon and J. S. Kim, Chem. Soc. Rev., 2011, 40, 3416–3429 RSC.
- M. Üçüncü, E. Karakuş and M. Emrullahoğlu, Chem. – Eur. J., 2015, 21, 13201–13205 CrossRef.
- T. Yao, X. Zhang and R. C. Larock, J. Am. Chem. Soc., 2004, 126, 11164–11165 CrossRef CAS.
- N. Sang, Y. Yun, H. Li, L. Hou, M. Han and G. K. Li, Toxicol. Sci., 2010, 114, 226–236 CrossRef CAS PubMed.
- S. X. Du, H. F. Jin, D. F. Bu, X. Zhao, B. Geng, C. S. Tang and J. B. Du, Acta Pharmacol. Sin., 2008, 29, 923–930 CrossRef CAS.
- V. S. Lin, W. Chen, M. Xian and C. J. Chang, Chem. Soc. Rev., 2015, 44, 4596–4618 RSC.
- Q. Zhang, Y. Zhang, S. Ding, H. Zhang and G. Feng, Sens. Actuators, B, 2015, 211, 377–384 CrossRef CAS.
- M. Li, W. Feng., H. Zhang and G. Feng, Sens. Actuators, B, 2017, 243, 51–58 CrossRef CAS.
- Y. Liu, K. Li, M.-Y. Wu, Y.-H. Liu, Y.-M. Xie and X.-Q. Yu, Chem. Commun., 2015, 51, 10236–10239 RSC.
- Y. Liu, K. Li, K.-X. Xie, L.-L. Li, K.-K. Yu, X. Wang and X.-Q. Yu, Chem. Commun., 2016, 52, 3430–3433 RSC.
- J. Xu, J. Pan, X. Jiang, C. Qin, L. Zeng, H. Zhang and J. F. Zhang, Biosens. Bioelectron., 2016, 77, 725–732 CrossRef CAS.
- Y. Sun, L. Ying, M. Xiatao and D. Lian, RSC Adv., 2016, 6, 79830–79835 RSC.
- Y. Wang, Q. Meng, R. Zhang, H. Jia, X. Zhang and Z. Zhang, Org. Biomol. Chem., 2017, 15, 2734–2739 RSC.
- G. Wang, H. Chen, X. Chen, X. Xiuli and X. Yongmei, RSC Adv., 2016, 6, 18662–18666 RSC.
- M. Zhao, D. Liu, L. Zhou, B. Wu, X. Tian, Q. Zhang, H. Zhou, J. Yang, J. Wu and Y. Tian, Sens. Actuators, B, 2018, 255, 1228–1237 CrossRef CAS.
- X.-L. Zheng, H. Li, W. Feng, H.-C. Xia and Q.-H. Song, ACS Omega, 2018, 3, 11831–11837 CrossRef CAS.
- W. Zhang, T. Liu, F. Huo, P. Ning, X. Meng and C. Yin, Anal. Chem., 2017, 89, 8079–8083 CrossRef CAS.
- Z. Ye, C. Duan, R. Sheng, J. Xu, H. Wang and L. Zeng, Talanta, 2018, 176, 389–396 CrossRef CAS.
- W. Ma, J. Du, J. Yin, J. Fan, S. Long and X. Peng, Sens. Actuators, B, 2018, 267, 104–110 CrossRef CAS.
- C. Zhen, C. Fengzao, S. Yuanchao, L. Heng, H. Hanping, Z. Ziuhua and W. Shengfu, RSC Adv., 2017, 7, 2573–2577 RSC.
- J. Lia, Y. Gao, H. Guo, X. Li, H. Tang, J. Li and Y. Guo, Dyes Pigm., 2019, 163, 285–290 CrossRef.
- X. Dai, T. Zhang, Z. F. Du, X. J. Cao, M. Y. Chen, S. W. Hu, J. Y. Miao and B. X. Zhao, Anal. Chim. Acta, 2015, 888, 138–145 CrossRef CAS.
- Q. Sun, W. Zhang and J. Qian, Talanta, 2017, 162, 107–113 CrossRef CAS.
- W.-L. Wu, H.-L. Ma, M.-F. Huang, J.-Y. Miao and B.-X. Zhao, Sens. Actuators, B, 2017, 241, 239–244 CrossRef CAS.
- U. Tamim, S. Singh, H. R. Kim, Y. J. Reo, Y. W. Jun, A. Das and K. H. Ahn, Sens. Actuators, B, 2018, 277, 576–583 CrossRef.
- J. Chao, X. Wang, Y. Liu, Y. Zhang, F. Huo, C. Yin, M. Zhao, J. Sun and M. Xu, Sens. Actuators, B, 2018, 272, 195–202 CrossRef CAS.
- S. Liu, L. Song, Q. Sun, Z. Chen, Y. Ge, W. Zhang and J. Qian, RSC Adv., 2015, 5, 91863–91868 RSC.
- H. Zhang, Z. Huang and G. Feng, Anal. Chim. Acta, 2016, 920, 72–79 CrossRef CAS PubMed.
- L.-J. Zhang, Z.-Y. Wang, J.-T. Liu, J.-Y. Miao and B.-X. Zhao, Sens. Actuators, B, 2017, 253, 19–26 CrossRef CAS.
- J. L. Way, Annu. Rev. Pharmacol. Toxicol., 1984, 24, 451–481 CrossRef CAS.
- H. Yamamoto and P. V. Mohanan, Toxicology, 2002, 179, 29–36 CrossRef CAS.
- J. Kang, E. J. Song, H. Kim, Y.-H. Kim, Y. Kim, S.-J. Kim and C. Kim, Tetrahedron Lett., 2013, 54, 1015–1019 CrossRef CAS.
- X. Sun, Y. Wang, X. Deng, J. Zhang and Z. Zhang, RSC Adv., 2016, 6, 10266–10271 RSC.
- J. Kang, F. Huo, Y. Zhang, J. Chao, T. E. Glass and C. Yin, Spectrochim. Acta, Part A, 2019, 209, 95–99 CrossRef CAS.
- H. Li, P. Zhao, N. Zou, H. Wang and K. Sun, Tetrahedron Lett., 2017, 58, 30–34 CrossRef CAS.
- F. Huo, Y. Zhang, Y. Yue, J. Chao, Y. Zhang and C. Yin, Dyes Pigm., 2017, 143, 270–275 CrossRef CAS.
- S. Lohar, K. Dhara, P. Roy, S. P. Sinha Babu and P. Chattopadhyay, ACS Omega, 2018, 3, 10145–10153 CrossRef CAS.
- M. Doherty, C. Becher, M. Regan, A. Jones and J. Ledingham, Ann. Rheum. Dis., 1996, 66, 432–436 CrossRef.
- S. Lee, K. K. Y. Yuen, K. A. Jolliffe and J. Yoon, Chem. Soc. Rev., 2015, 44, 1749–1762 RSC.
- J. F. Zhang, L. E. Guo, T. N. Zang, Y. L. Duan, X. Y. Liu, Z. Yang, P. Verwilst, K. Luo, G. K. Wang, J. F. Kou, Y. Zhou and J. S. Kim, Chem. – Asian J., 2015, 10, 1165–1169 CrossRef CAS.
- S. Y. Jiao, K. Li, W. Zhang, Y. H. Liu, Z. Huang and X. Q. Yu, Dalton Trans., 2015, 44, 1358–1365 RSC.
- H. S. Horowitz, J. Public Health Dent., 2003, 63, 3–8 CrossRef.
- M.-L. Cittanova, B. Lelongt, M.-C. Verpont, M. Geniteau-Legendre, F. Wahbe, D. Prie, P. Coriat and P. M. Ronco, Anesthesiology, 1996, 84, 428–435 CrossRef CAS.
- E. B. Bassin, D. Wypij, R. B. Davis and M. A. Mittleman, Cancer Causes Control, 2006, 17, 421–428 CrossRef.
-
J. Fawell, K. Bailey, J. Chilton, E. Dahi and Y. Magara, Fluoride in drinking-water, IWA publishing, 2006 Search PubMed.
-
W. H. Organization, Guidelines for drinking-water quality, World Health Organization, 2004 Search PubMed.
- Y. Zhou, J. F. Zhang and J. Yoon, Chem. Rev., 2014, 114, 5511–5571 CrossRef CAS.
- Z. Wu and X. Tang, Anal. Chem., 2015, 87, 8613–8617 CrossRef CAS.
- W. Hu, L. Zeng, Y. Wang, Z. Liu, X. Ye and C. Li, Analyst, 2016, 141, 5450–5455 RSC.
- X. Zhu, J. Wang, J. Zhang, Z. Chen, H. Zhang and X. Zhang, Sensors, 2015, 15, 1611–1622 CrossRef CAS.
- Y. Shen, X. Zhang, Y. Zhang, H. Li and Y. Chen, Sens. Actuators, B, 2018, 258, 544–549 CrossRef CAS.
- X. Tian, X. Tong, Z. Li, D. Li, Q. Kong and X. Yang, J. Agric. Food Chem., 2018, 66, 11486–11491 CrossRef CAS.
- Y. Zhang, Y. Deng, N. Ji, J. Zhang, C. Fan, T. Ding, Z. Cao, Y. Li and Y. Fang, Dyes Pigm., 2019, 166, 473–479 CrossRef CAS.
- C. Huang, T. Jia, C. Yu, A. Zhang and N. Jia, Biosens. Bioelectron., 2015, 63, 513–518 CrossRef CAS.
- C. M. Weekley and H. H. Harris, Chem. Soc. Rev., 2013, 42, 8870–8894 RSC.
- D. Wu, L. Chen, N. Kwon and J. Yoon, Chem, 2016, 1, 674–698 CAS.
- Y. Tian, F. Xin, J. Jing and X. Zhang, Dyes Pigm., 2019, 164, 133–138 CrossRef CAS.
Footnote |
† These authors contributed equally to this work. |
|
This journal is © The Royal Society of Chemistry 2020 |