DOI:
10.1039/C9SC03715B
(Edge Article)
Chem. Sci., 2019,
10, 9836-9840
Chemoselective formal β-functionalization of substituted aliphatic amides enabled by a facile stereoselective oxidation event†
Received
28th July 2019
, Accepted 29th August 2019
First published on 10th September 2019
Abstract
Aliphatic C–H functionalization is a topic of current intense interest in organic synthesis. Herein, we report that a facile and stereoselective dehydrogenation event enables the functionalization of aliphatic amides at different positions in a one-pot fashion. Derivatives of relevant pharmaceuticals were formally functionalized in the β-position in late-stage manner. A single-step synthesis of incrustoporine from a simple precursor further showcases the potential utility of this approach.
Introduction
The carbonyl functional group remains one of the most important synthetic handles in organic chemistry.1,2 While the α-carbon atom and the ipso-position of this ubiquitous structural motif can be modified in many ways,2 the direct modification of the β-position is more elusive. With the advent of C–H functionalization, transformation of molecular moieties which are traditionally perceived as unreactive became feasible.1–3 The past decades have witnessed an explosive wealth of accomplishments regarding this family of transformations.3 Relying on the transient generation of internally chelated, cyclopalladated intermediates such as 2 (Scheme 1a), this mode of C–H functionalization has been effectively exploited particularly for β-methyl carbonyl derivatives.4 The emerging field of photoredox catalysis has also proven to be a powerful tool for the β-functionalization of cyclic ketones.5
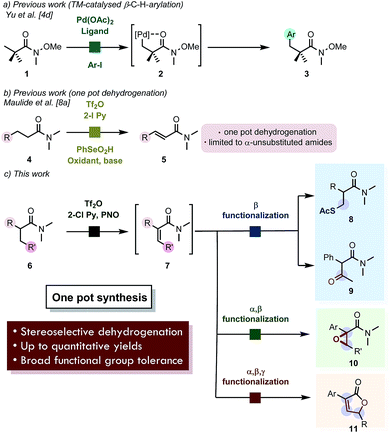 |
| Scheme 1 (a) Previous work on β-C–H functionalization of aliphatic, quaternary carboxamides. (b) Earlier report on dehydrogenation of carboxamides by electrophilic activation. (c) Chemoselective, formal remote functionalization of carboxamides enabled by stereoselective dehydrogenation. | |
More conventional approaches make use of classical Michael addition strategies. However, the requisite α,β-unsaturated carbonyl compound is not always readily available. While the oxidative dehydrogenation of α-branched chloroenamines has been previously realized by Ghosez et al.8b (though no E/Z selectivity was described), we have recently disclosed a selenium-mediated, one-pot α,β-dehydrogenation of amides (cf. – 4 → 5, Scheme 1b) in moderate to good yields. More recently a stepwise dehydrogenation using α-TEMPO (tetramethylpiperidinyloxyl) amides as intermediates for the dehydrogenation of linear amides has been reported.8c
This approach is predicated on the electrophilic activation of carboxamides, a reactivity mode that enables transformations ranging from the venerable [2 + 2] cycloaddition6a–d and ipso substitution6e–g reactions all the way to the functionalization of the α-position via rearrangement and Umpolung chemistry.7 However, the method depicted in Scheme 1b is limited to α-unsubstituted amides.8a In this manuscript, we report a stereoselective and mild α,β-dehydrogenation and how it enables not only the direct formal β-C(sp3)–H functionalization of simple amides under a metal-free regime, but also the functionalization of multiple carbon atoms in α,β and γ-positions.
Results and discussion
Preliminary results
During our studies on the Umpolung functionalization of amides,7 we observed that α-branched amides deviate from Umpolung reactivity at the α-carbon. Instead, treatment of α-branched amides with trifluoromethanesulfonic anhydride (Tf2O), 2-iodo pyridine and pyridine N-oxide (PNO) resulted in clean and high-yielding α,β-dehydrogenation rather than the Umpolung reactivity we had previously observed.9 Moreover, α-aryl-substituted amides gave quantitative yield with the exclusive formation of one alkene-isomer. Importantly, careful analysis revealed that the dehydrogenation of α-aryl substituted amides gave exclusively the Z-olefin (by NMR), whose structure was confirmed by NOESY-NMR spectroscopy (cf.Scheme 2a, 6a → 7a). On the other hand, α-branched dialkyl amides typified by 12 afforded an inseparable mixture of regio- and stereoisomers (Scheme 2b) with moderate selectivity. In particular, when the isopropyl-substituted, α-branched amide 14 was submitted to the reaction conditions we observed (Scheme 2c) equal amounts of the expected tetrasubstituted alkene 15a and the unexpected β,γ-dehydrogenated amide 15b by NMR. The latter product is presumably formed by a Wagner–Meerwein rearrangement of the putative unstable carbocation 16 as shown (Scheme 2c). It is noteworthy that 14 reacted sluggishly.
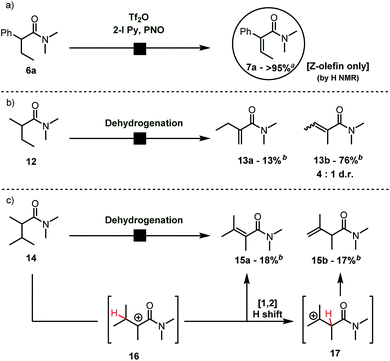 |
| Scheme 2 (a) Preliminary results. (b) Dehydrogenation of purely aliphatic α-branched amides. (c) Unexpected formation of a β,γ-dehydrogenated amide. a Yield refers to the pure isolated product. b 1H NMR yield with mesitylene as the internal standard. | |
The dehydrogenation reaction leads to very similar results regardless of whether 2-chloro- or 2-bromo pyridine is used instead of 2-iodo pyridine (a relevant piece of information for future developments, vide infra. See the ESI for further details†).
No reaction intermediates en route to dehydrogenation could be detected by NMR spectroscopy. We believe that the reaction proceeds by swift fragmentation of the enolonium species 19a to an α-carbocationic amide 20 (Scheme 3).14 A rationale for the high Z/E selectivity is provided by the model shown in Scheme 3. Efficient mesomeric stabilization of the putative carbocationic intermediate mandates perpendicular arrangement of the adjacent aromatic moiety, resulting in restricted rotation of the arene substituent.14 This exacerbates steric hindrance considerations, disfavoring a scenario where even a methyl group in the β-position is on the same side as the arene ring. As steric congestion increases during formation of the double bond, it is likely that elimination is greatly favoured from the thermodynamically favored conformer 20a.
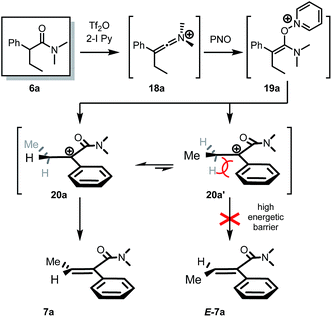 |
| Scheme 3 Proposed mechanism and stereochemical model for the observed high stereoselectivity of the dehydrogenation event.14 | |
One pot α,β-epoxidation
This surprisingly stereoselective and efficient result (quantitative conversion within minutes at room temperature) encouraged us to leverage in situ oxidation in order to achieve formal remote functionalization reactions. In particular, we were looking to draw on the high diastereoselectivity of the dehydrogenation event. In our hands, Prilezhaev oxidation using meta-chloroperbenzoic acid (commercial grade mCPBA) proceeded smoothly in quantitative yield at room temperature (Scheme 4).10
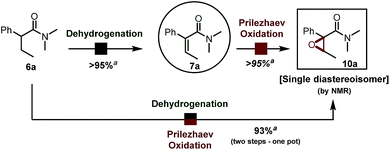 |
| Scheme 4 α,β-Epoxidation of a simple amide. See ESI† for detailed reaction conditions. a Yield refers to the pure isolated product. | |
When we investigated the possibility of a one pot procedure an unexpected hurdle was encountered, in that the original procedure using 2-iodo pyridine for the dehydrogenation step was not compatible with the Prilezhaev oxidation, most likely due to the easily oxidized iodine atom. This issue was readily circumvented by using 2-chloro pyridine instead. Pivotal to the one-pot procedure with 2-chloro pyridine was the addition of an acid quencher (water or an aqueous NaHCO3 solution) before adding mCPBA, owing to the high acidity of protonated 2-chloropyridine present in the solution (pKa = 0.49 in H2O).12
By use of this procedure, formal α,β-oxygenation of simple amide 6a was achieved in 93% isolated yield in one pot. The reaction proceeds with a very similar yield on a gram scale. As shown in Scheme 5a, this transformation has some generality and tolerates a range of functional groups, such as esters (10d, 10f), nitriles (10e) and silyl ethers (10c). Electron-withdrawing (such as pCF3, 10n) and -donating groups (such as OMe, cf.10j) are well tolerated at diverse positions of the aryl substituent (Scheme 5a). Heating to 40 °C was found to be generally beneficial during the epoxidation event to enhance conversion in cases were the aromatic ring is electronically impoverished.11 The use of aqueous NaHCO3 instead of water enables successful reaction for acid-sensitive substrates (e.g. TBS protected alcohol, cf.10c) or sensitive products (electron rich aromatics in proximity to the formed epoxide, cf.10j). Diastereoselectivity was found to be excellent in all cases: the only case in which the other diastereoisomer was detected in the crude reaction mixture (in traces by 1H NMR, d.r. > 25
:
1) is the sterically congested product 10b. These epoxides can serve as precursors to more elaborated architectures as depicted in Scheme 5b. NaHMDS-mediated deprotonation of 10e, which was obtained in good yield using the present protocol, led to diastereoselective intramolecular epoxide opening.13 The resulting tertiary alcohol 22, which contains three contiguous stereogenic centers, was obtained as a single diastereoisomer.
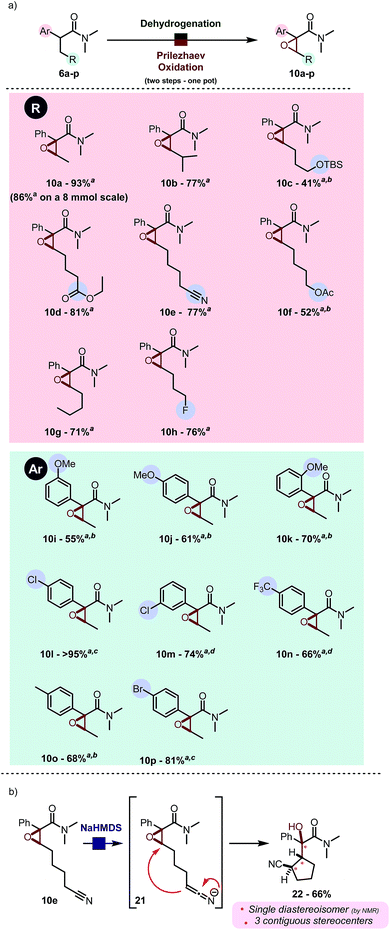 |
| Scheme 5 (a) Product scope of the one pot desaturation–epoxidation reaction. Reactions were carried out on a 0.2 mmol scale in DCM (0.2 M) with Tf2O (1.1 eq.), 2-Cl Py (2.2 eq.), PNO (1.3 eq.) at 0 °C then r.t., H2O and mCPBA (3.0 eq.) at r.t. a Yield refers to the pure isolated product. b Saturated aqueous NaHCO3 used instead of water. c Reaction was heated to 40 °C during epoxidation d Reaction was carried out in 1,2-DCE instead of DCM and heated to 60 °C during epoxidation. (b) Application of one of the products. | |
One pot β-oxidation and oxidative C–C bond cleavage
Interestingly (Scheme 6a), when the acid scavenger was omitted in the domino α,β-oxidation of amide 6a, α-ketoamide 23a was obtained as the major product, accompanied by trace amounts of α-acetoxyamide 24a and β-ketoamide 9a. Selectivity can be steered exclusively towards β-ketoamide 9a by adding first a non-aqueous proton-scavenger (Na2HPO4) and then acid (trifluoroacetic acid, TFA).15 When the purified β-ketoamide 9a (isolated in 88% yield) was treated with mCPBA in DCM, we observed α-ketoamide 23a as the main product with the α-acetoxy derivative 24a as a side product. We thus propose a mechanistic scenario (Scheme 6b) whereby acid-induced Meinwald rearrangement15 of epoxide 10a delivers ketone 9a. Baeyer–Villiger oxidation of the latter with mCPBA (preferential migration of the benzylic substituent)16 combined with nucleophilic attack of mCPBA anion/Kornblum–DeLaMare rearrangement17 accounts for the formation of products 23a and 24a. In particular, the β-oxidation of an amide to a β-ketoamide (6a → 9a) is, to the best of our knowledge, an unknown transformation with potential synthetic utility.
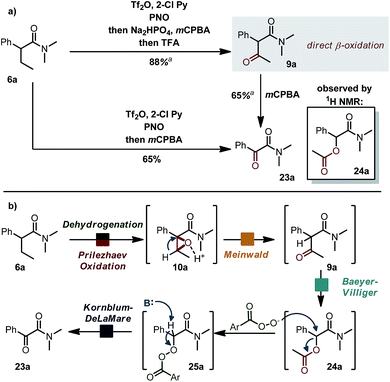 |
| Scheme 6 (a) Alternative pathway towards different ketoamides. See ESI† for detailed reaction conditions. (b) Proposed mechanism. | |
Synthesis of 2-furanones
When combined with allylic oxidation,18 the mild dehydrogenation chemistry described herein results in a one-step synthesis of butenolides from simple carboxamides (Scheme 7). The oxidation is most likely followed by nucleophilic displacement of the R–O–Se–OH functionality via the amide carbonyl.19 The stereoretention of the double bond geometry during oxidation is noteworthy, and the absence of products with inversion of the double bond configuration can be rationalised by the transition state of the [2,3] rearrangement of intermediate 26 (ref. 20). The reaction enables, for instance, the single-step preparation of the antifungal natural product incrustoporine 11q (or analogues such as 11g).21
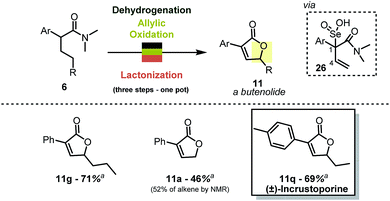 |
| Scheme 7 One-pot synthesis of butenolides. Reactions were carried out on a 0.2 mmol scale in 1,2-DCE (0.1 M) with Tf2O (1.1 eq.), 2-I Py (2.2 eq.), PNO (1.3 eq.) at 0 °C then r.t., 1,4-dioxane and SeO2 (2.0 eq.) at 80 °C. a Yield refers to the pure, isolated product. | |
One pot β-thiolation
Finally, when combined with the addition of thioacetic acid under basic conditions, the chemistry reported herein results in formal direct β-thiolation.22 As shown in Scheme 8, several derivatives of relevant drugs and biologically active compounds could be thus converted into their β-thiolated analogues. Namely, the dimethylamides of Ibruprofen, Naproxen, Flubriprofen and Ketoprofen all underwent β-thiolation in good to quantitative yields (cf.Scheme 6, 8r,s,t,v). Moreover, this chemistry allows interconversion of proteogenic aminoacid derivatives: an amide derivative of alanine 6u was converted into the corresponding cysteine derivative 8u in 76% yield. The metal-free character of these transformations and the substrate complementarity to metal-catalysed processes are worthy of note.
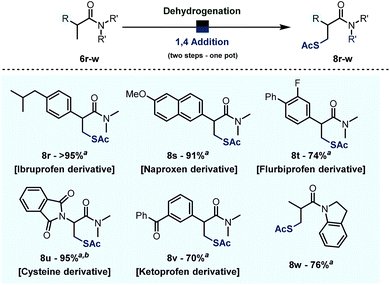 |
| Scheme 8 Product scope of the desaturation – 1,4 addition reaction on biologically relevant substrates. Reactions were carried out on a 0.2 mmol scale in 1,2-DCE (0.2 M) with Tf2O (1.1 eq.), 2-I Py (2.2 eq.), PNO (1.3 eq.) at 0 °C then 60 °C, AcSH (4.0 eq.), Et3N (2.0 eq.). a Yield refers to the isolated product. b Reaction was heated to 60 °C during desaturation. | |
Conclusion
In conclusion, we have shown herein that a facile and chemoselective dehydrogenation event can be leveraged to achieve a plethora of one-pot oxidations/functionalisations of carboxamides. This results in formal direct α,β-epoxidation, direct conversion to α- or β-ketoamides at will and the one-step interconversion of a simple linear amide into butenolides by formal α,β,γ-oxidation. The ability to deploy this chemistry in a formal β-thiolation hints at its potential utility for late-stage derivatization.
Conflicts of interest
There are no conflicts to declare.
Acknowledgements
Funding of this work by the Austrian Science Fund (FWF, P30226) and the European Research Council (ERC, CoG 682002 VINCAT) is acknowledged. We thank the University of Vienna for its continued and generous support of our research programs. Dr J. Li (Univ. Vienna) is acknowledged for the discovery of the dehydrogenation reaction. Dr W. Zawodny and Dr M. Vayer (both Univ. Vienna) are gratefully acknowledged for proofreading and editing.
References
-
D. W. Knight, in Reference Module in Chemistry, Molecular Sciences and Chemical Engineering: Organic Methodology and Organic Synthesis, Elsevier Inc., 2013 Search PubMed.
-
S. Caron, in Practical synthetic organic chemistry, Wiley & Sons Inc., 2011 Search PubMed.
- For recent reviews on C–H functionalization see:
(a) P. Gandeepan, T. Müller, D. Zell, G. Cera, S. Warratz and L. Ackermann, Chem. Rev., 2019, 119, 2192–2452 CrossRef CAS PubMed;
(b) C. Ma, P. Fang and T.-S. Mei, ACS Catal., 2018, 8, 7179–7189 CrossRef CAS;
(c) H. Yi, G. Zhang, H. Wang, Z. Huang, J. Wang, A. K. Singh and A. Lei, Chem. Rev., 2017, 117, 9016–9085 CrossRef CAS PubMed;
(d) D. J. Abrams, P. A. Provencher and E. J. Sorensen, Chem. Soc. Rev., 2018, 47, 8925–8967 RSC.
-
(a) P.-X. Shen, L. Hu, Q. Shao, K. Hong and J.-Q. Yu, J. Am. Chem. Soc., 2018, 140, 6545–6549 CrossRef CAS PubMed;
(b) M. Wasa, K. M. Engle and J.-Q. Yu, J. Am. Chem. Soc., 2009, 131, 9886–9887 CrossRef CAS PubMed;
(c) Z. Huang and G. Dong, Tetrahedron Lett., 2014, 55, 5869–5889 CrossRef CAS;
(d) H. Park, N. Chekshin, P.-X. Shen and J.-Q. Yu, ACS Catal., 2018, 8, 9292–9297 CrossRef CAS;
(e) M. Wasa and J.-Q. Yu, Tetrahedron, 2010, 66, 4811–4815 CrossRef CAS PubMed;
(f) T. G. Saint-Denis, R. Y. Zhu, G. Chen, Q.-F. Wu and J.-Q. Yu, Science, 2018, 359, eaao4798 CrossRef PubMed;
(g) Q.-F. Wu, P.-X. Shen, J. He, X.-B. Wang, F. Zhang, Q. Shao, R.-Y. Zhu, C. Mapelli, J. X. Qiao, M. A. Poss and J.-Q. Yu, Science, 2017, 355, 499–503 CrossRef CAS.
-
(a) F. R. Petronijević, M. Nappi and D. W. C. MacMillan, J. Am. Chem. Soc., 2013, 135, 18323–18326 CrossRef PubMed;
(b) J. Ma, A. R. Rosales, X. Huang, K. Harms, R. Riedel, O. Wiest and E. Meggers, J. Am. Chem. Soc., 2017, 139, 17245–17248 CrossRef CAS PubMed.
- For reviews on the activation of amides with chapters including their functionalization see:
(a) D. Kaiser, A. Bauer, M. Lemmerer and N. Maulide, Chem. Soc. Rev., 2018, 47, 7899–7925 RSC;
(b) L. Ghosez, Angew. Chem., Int. Ed. Engl., 1972, 11, 852–853 CrossRef CAS;
(c) A. Sidani, J. Marchand-Brynaert and L. Ghosez, Angew. Chem., Int. Ed. Engl., 1974, 13, 267–268 CrossRef;
(d) R. Bisceglia and C. J. Cheer, J. Chem. Soc., Chem. Commun., 1973, 165–166 RSC;
(e) O. Wallach, Liebigs Ann. Chem., 1877, 184, 1–127 CrossRef;
(f) J. v. Braun and A. Heymons, Chem. Ber., 1929, 62, 409–413 CrossRef;
(g) L. Ghosez, B. Haveaux and H. G. Viehe, Angew. Chem., Int. Ed. Engl., 1969, 8, 454–455 CrossRef CAS.
-
(a) P. Adler, C. J. Teskey, D. Kaiser, M. Holy, H. H. Sitte and N. Maulide, Nat. Chem., 2019, 11, 329–334 CrossRef CAS PubMed;
(b) D. Kaiser, C. J. Teskey, P. Adler and N. Maulide, J. Am. Chem. Soc., 2017, 139, 16040–16043 CrossRef CAS PubMed;
(c) D. Kaiser, A. de la Torre, S. Shaaban and N. Maulide, Angew. Chem., Int. Ed., 2017, 56, 5921–5925 CrossRef CAS PubMed.
-
(a) C. J. Teskey, P. Adler, C. R. Gonçalves and N. Maulide, Angew. Chem., Int. Ed., 2019, 58, 447–451 CrossRef CAS PubMed;
(b) R. Da Costa, M. Gillard, J. B. Falmagne and L. Ghosez, J. Am. Chem. Soc., 1979, 101, 4381–4383 CrossRef CAS;
(c) M.-M. Wang, G.-H. Sui, X.-C. Cui, H. Wang, J.-P. Qu and Y.-B. Kang, J. Org. Chem., 2019, 84, 8267–8274 CrossRef CAS PubMed.
- The reaction proceeds well with 2-halo pyridines (except for 2-fluoro pyridine) and 2-OMe pyridine, allowing quantitative dehydrogenation within 60 minutes. For optimization details, see the ESI.†.
- N. Prilezhaev, Ber. Dtsch. Chem. Ges., 1909, 42, 4811–4815 CrossRef.
- The ortho-Cl analogue was observed in reasonable NMR yields, but the product could not be isolated in satisfactory yields due to difficult purification.
-
(a)
D. D. Perrin, in Dissociation constants of organic bases in aqueous solution, Butterworths, London, 1965 Search PubMed;
(b) After addition of water to the reaction mixture, the pH of the aqueous layer was qualitatively measured to be ≤1.
-
(a) G. Stork, L. D. Cama and D. R. Coulson, J. Am. Chem. Soc., 1974, 96, 5268–5270 CrossRef CAS;
(b) G. Stork and J. F. Cohen, J. Am. Chem. Soc., 1974, 96, 5270–5272 CrossRef CAS;
(c) S. G. Levine and M. P. Bonner, Tetrahedron Lett., 1989, 30, 4767–4770 CrossRef CAS;
(d) K. Takahashi, K. Komine, Y. Yokoi, J. Ishihara and S. Hatakeyama, J. Org. Chem., 2012, 77, 7364–7370 CrossRef CAS PubMed.
- Anchimeric stabilization, as previously reported for similar species, probably results in an equilibrium with the corresponding 3-membered iminium ether. For related references, see:
(a) D. J. Pasto and M. P. Serve, J. Am. Chem. Soc., 1965, 87, 1515–1521 CrossRef CAS;
(b) J. P. Bégué and M. Charpentier-Morize, Acc. Chem. Res., 1980, 13, 207–212 CrossRef.
-
(a)
Z. Wang, Meinwald Rearrangement, in Comprehensive Organic Name Reactions and Reagents, Wiley, Hoboken, NJ, 2010, pp. 1880–1882 CrossRef;
(b) J. Meinwald, S. S. Labana and M. S. Chadha, J. Am. Chem. Soc., 1963, 85, 582–585 CrossRef CAS.
-
(a) A. Baeyer and V. Villiger, Ber. Dtsch. Chem. Ges., 1899, 32, 3625–3633 CrossRef;
(b) G.-J. ten Brink, I. W. C. E. Arends and R. A. Sheldon, Chem. Rev., 2004, 104, 4105–4124 CrossRef.
- N. Kornblum and H. E. DeLaMare, J. Am. Chem. Soc., 1951, 73, 880–881 CrossRef CAS.
-
(a) H. L. Riley, J. F. Morley and N. A. C. Friend, J. Chem. Soc., 1932, 1875–1883 RSC;
(b) A. Guillemonat, Ann. Chim. Appl., 1939, 11, 143–211 CAS.
- For examples of domino allylic oxidation/lactonization see:
(a) N. Danieli, Y. Mazur and F. Sondheimer, Tetrahedron Lett., 1961, 2, 310–312 CrossRef;
(b) R. M. Patel, V. G. Puranik and N. P. Argade, Org. Biomol. Chem., 2011, 9, 6312–6322 RSC.
-
(a) H. Rapoport and U. T. Bhalerao, J. Am. Chem. Soc., 1971, 93, 4835–4840 CrossRef CAS;
(b) C. S. Ra and G. Park, Tetrahedron Lett., 2003, 44, 1099–1102 CrossRef CAS;
(c) D. A. Singleton and C. Hang, J. Org. Chem., 2000, 65, 7554–7560 CrossRef CAS.
- A. Lu, J. Wang, T. Liu, J. Han, Y. Li, M. Sin, J. Chen, H. Zhang, L. Wang and Q. Wang, J. Agric. Food Chem., 2014, 62, 8799–8807 CrossRef CAS PubMed.
-
(a) A. U. Rajshekhar, N. K. Rana and V. K. Singh, Tetrahedron Lett., 2013, 54, 1911–1915 CrossRef;
(b) L. Hintermann and A. Turočkin, J. Org. Chem., 2012, 77, 11345–11348 CrossRef CAS PubMed.
Footnote |
† Electronic supplementary information (ESI) available. See DOI: 10.1039/c9sc03715b |
|
This journal is © The Royal Society of Chemistry 2019 |