DOI:
10.1039/C9SC03710A
(Edge Article)
Chem. Sci., 2019,
10, 10556-10561
Sustainable ppm level palladium-catalyzed aminations in nanoreactors under mild, aqueous conditions†
Received
27th July 2019
, Accepted 19th September 2019
First published on 20th September 2019
Introduction
Ligated palladium has been and continues to be a privileged catalyst in the realm of organic synthesis.1 In particular, the widespread application of Heck, Suzuki–Miyaura, and Negishi coupling reactions earned them the highest appreciation in chemistry.2 Palladium-catalyzed aminations have also been pursued enthusiastically in fields ranging from pharmaceuticals and agrochemicals to others in the fine chemical industry.3 The versatility and utility of such aminations has also made it among the more reliable methods for construction of C–N bonds that are so prevalent in many target molecules.4 Since the mid 1990's, extensive ligand design as well as mechanistic investigations have emerged, leading to commonly employed phosphine ligands that tend to be monodentate, bidentate, or dialkylbiarylphosphines.5 Development of pre-catalysts that generate active Pd(0) species in the reaction mixture have been also actively investigated.6 Notable examples include Pd/phosphine ligand complexes, palladacycles, and NHCs, among others.6 Several generations of pre-catalysts have been developed, with each system allowing a seemingly broader substrate scope.7 Nonetheless, much of the literature suggests that conditions typically used for these aminations are generally harsh, involving high boiling organic solvents (e.g., toluene, dioxane, DMF, etc.) as the reaction medium, oftentimes requiring extended reaction times.3 Moreover, catalyst loadings in the 2–10 mol% range of Pd are not uncommon,4e representing a non-sustainable scenario given the endangered status of platinoids.8 Hence, a procedure that is not only general and mild but can also be used under environmentally responsible conditions would be highly desirable, perhaps encouraging further use of this approach to C–N bond formation. Towards this goal, we and others have identified ligand scaffolds that complex Pd forming catalysts that mediate such couplings under mild, micellar conditions in water.9 However, the crucial reaction parameters involving reduction in the amount of endangered metal required for catalysis to a sustainable level had yet to be achieved; indeed, in addition to high loadings being the norm, even greater amounts of privileged ligands are required. To begin to convert Pd-catalyzed aminations to truly sustainable processes, we have endeavored to develop, and this contribution report on, a general protocol that takes place in water, requires little-to-no investment in energy other than that available between room temperature and 45 °C, and is particularly mindful of the endangered status of palladium.8,10
Reducing the loading of a Pd catalyst by 1–2 orders of magnitude requires a significant net increase in reagent activity. Realization of this goal becomes all the more challenging in that high levels of reaction conversion must be realized within reasonable timeframes. Among the many catalysts screened for this study, some of which are shown in Scheme 1, we took special note of reports by Colacot and co-workers describing two new classes of π-allylpalladium complexes containing biarylphosphine ligands.6d,f
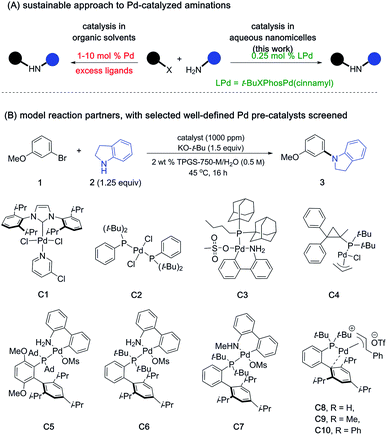 |
| Scheme 1 Defined LPd complexes used at 1000 ppm loading level (see ESI† for the complete list of pre-catalysts screened). | |
Though mechanistic aspects of these studies were not investigated in aqueous media, in organic solvents it was shown that active “L-Pd(0)” species could be generated from either neutral or cationic ligated frameworks that are both air- and moisture-stable.6f These preformed monoligated-Pd complexes are particularly appealing as they avoid use of excess ligands which are formally unnecessary for the catalytic cycle, ultimately generate organic waste, and can add cost to the overall process. Moreover, such catalyst precursors help ensure consistent results between batches, which has obvious advantages over in situ preparations both at an early stage of discovery as well as in future applications, especially at scale.11
Results and discussion
A model reaction between 3-bromoanisole (1) and indoline (2) was initially used to screen for catalyst activity, where only 1000 ppm (i.e., 0.10 mol%) of the Pd-containing catalyst was invested. All reactions were run using nanomicelles derived from TPGS-750-M in water between rt and 45 °C. While several pre-catalysts failed to deliver any detectable product, systems featuring a biarylphosphine scaffold proved more active, as noted previously by the Schmitt group.9e Among t-BuXPhos-based complexes, the three cationic species [t-BuXPhos(Pd-π-allyl)]OTf (C8), [t-BuXPhos(Pd-π-crotyl)]OTf (C9) and [t-BuXPhos(Pd-π-cinnamyl)]OTf (C10) each led with high efficiency to the desired amination product. The most lipophilic pre-catalyst, C10, afforded the diarylamine 3 in close to quantitative yield. Use of triflate salts was crucial, as the corresponding chlorides were less effective, presumably due to their tendency to form dimeric complexes via bridging chloride ions, species that are apparently catalytically inferior.6d–f
Having identified C10 as the most effective pre-catalyst under micellar conditions at the 1000 ppm level of Pd, we evaluated the scope of this C–N coupling chemistry between anilines/aniline-like amines and aryl bromides (Scheme 2). Primary and secondary anilines reacted efficiently between rt and 45 °C with aryl bromides bearing electron-donating, -withdrawing, or neutral substituents (products 3–20). A highly functionalized heterocyclic bromide from the Merck Informer Library X3 (ref. 12) also participated to give the desired product 18.13 Aryl chlorides were totally inert under these conditions (e.g., 8), and hence, complete selectivity for a bromide over chloride is assured based on formation of products 15, 23, 24, and 27. For reactions that proved to be particularly sluggish, an increase in the loading of Pd (from 0.10 to 0.25 mol%) led to the desired C–N bonds, as shown for products 21–27. Among these, secondary alkyl amino compounds such as morpholine (to give 21) and pyrrolidine (to give 25) could be used, though an excess of inorganic base was needed to achieve reasonable levels of conversion in both cases. Multiple C–N cross-couplings in 1-pot could be effected leading to both C2-, C3-symmetric, and axially chiral amination products 28–30, each in high overall yield. The latter two products required an increase to 5000 ppm of Pd catalyst, although multiple C–N bonds were made in each case.
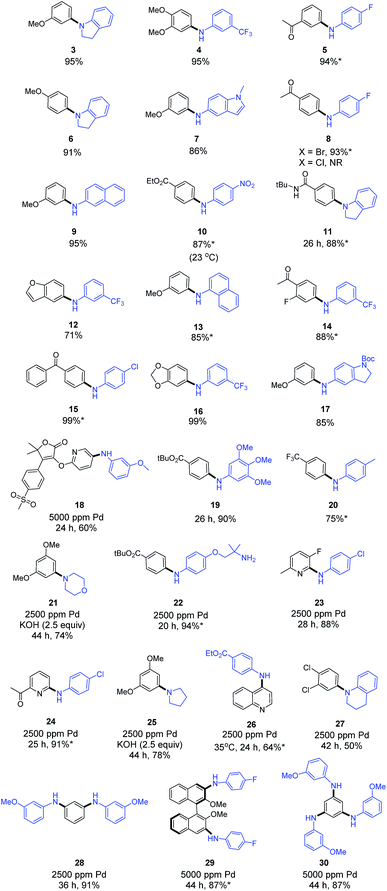 |
| Scheme 2 Representative examples of aminations under sustainable aqueous micellar conditions. Unless otherwise mentioned: X = Br, [LPd] = 1000 ppm [t-BuXPhosPd(cinnamyl)]OTf, temp = 45 °C, time = 16 h; KO-t-Bu or K3PO4·H2O used as inorganic base in 2 wt% TPGS-750-M at 0.5 M global concentration (see ESI† for additional details). *Product isolated by simple filtration. | |
Procedurally, this new protocol involves a very straightforward benchtop setup, requires no glovebox operations, and the catalysts used (e.g., C8 and C10) are easily prepared and stored.6f Moreover, in many of the cases shown in Scheme 2, the solid products formed precipitate out of the aqueous medium necessitating only filtration, thereby avoiding any formal workup. Subsequent trituration leads to pure product without reliance on column chromatography, further minimizing both organic solvent and aqueous waste streams.
As aniline coupling partners are susceptible to oxidation, they are best purified prior to use. On the other hand, the corresponding nitro group-containing derivatives, as precursors, are often stable solids at ambient temperatures. Combining, therefore, a nitro group reduction followed (without isolation) by a ppm level Pd-catalyzed amination would expand the appeal of this methodology, especially for industrial applications. We have previously developed a nitro group reduction protocol using the same aqueous micellar medium, where commercially available and inexpensive carbonyl iron powder (CIP) has been shown to be amenable to scale-up, delivering grams of amination products.8g As illustrated in Scheme 3A, the crude amine product from the reduction of 31, following filtration through Celite, could be used directly in the amination protocol to afford the cross-coupling product 32 in good overall yield.
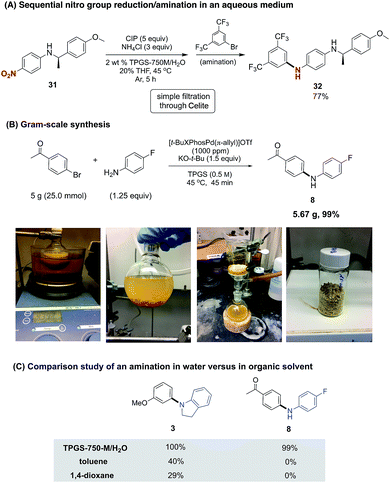 |
| Scheme 3 (A) Sequential sustainable micellar catalysis leading to 32. (B) Gram scale synthesis of 8 in TPGS-750-M with easy product isolation. (C) Comparison study on the formation of 3 and 8 in TPGS-750-M vs. in organic solvent. Conditions: 45 °C, 1000 ppm LPd catalyst loading, 0.5 M, 16 h. | |
A gram-scale amination led to the preparation of diarylamine 8, starting with 4-fluoroaniline and 4-bromoacetophenone (Scheme 3B). The almost equally effective pre-catalyst [t-BuXPhos(Pd-π-allyl)]OTf (C8)14 was used due to its commercial availability. Without alteration from our optimized conditions (vide supra), the reaction run at 100 times its original scale was complete in less than one hour. More than five grams of pure product could be collected via filtration, with an astonishingly low E factor (<1), as a measure of waste created (see ESI† for details).15
While the synthesis of 8 was highly efficient at the ppm level of catalyst under aqueous conditions, such was not the case when performed in organic solvents commonly used for these aminations.4e Thus, in either toluene or 1,4-dioxane under otherwise identical conditions, none of the desired product was detected (Scheme 3C). NMR analyses showed a combination of the corresponding imine had formed, along with unreacted bromoacetophenone in ca. a 1
:
1 ratio. A similar solvent comparison study was performed leading to amine 3, although low yields were obtained in this case as well. In the composite, these observations highlight the potentially significant (e.g., chemoselectivity) differences that can be found between traditional and micellar catalysis.
Although increasing the loading (e.g., from 0.10 to 0.25 mol%; see Scheme 2) for especially difficult C–N coupling reactions leads to formation of the desired amination, the same net outcome could be obtained using 1250 ppm Pd simply by a 10 °C increase in reaction temperature. Comparison studies leading to product 23 clearly showed that this was indeed the case (Scheme 4A).
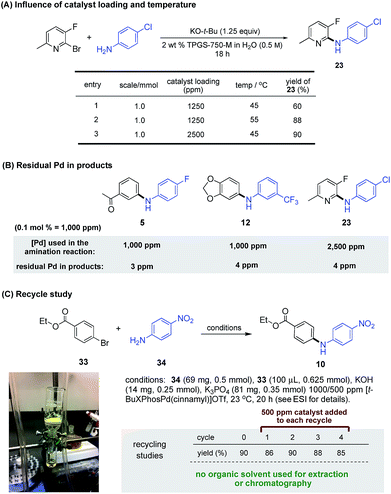 |
| Scheme 4 (A) Influence of reaction scale, catalyst loading and temperature on micellar amination (B) ICP analysis of residual Pd detected in compounds prepared by ppm LPd micellar catalysis. (C) Recycle study of Buchwald–Hartwig amination at rt (see ESI† for details). | |
By virtue of the ppm level of Pd catalyst associated with these aminations, the amount of residual metal to be found in the product amines, after standard workup and purification, is expected to be below the FDA-approved level of 10 ppm per dose.16 As test cases, products (e.g., 5, 12, and 23; Scheme 4B) were randomly selected and subjected to ICP-MS analyses for Pd content. Results (see ESI† for details) indicated that each has a Pd content ≤4 ppm, suggesting that additional time and cost associated with further purification, contrary to that based on traditional couplings done at 10–100 times the loading of Pd, should not be needed for products formed using this technology. Further reduction in the amount of Pd invested can be achieved by recycling of the aqueous reaction medium. As shown in the coupling between ethyl p-bromobenzoate (33) and p-nitroaniline (34; Scheme 4C), the solid diarylamine product 35 is isolated by simple gravity filtration. The filtrate is then reused as the reaction medium after supplementing with 500 ppm of ligated Pd. As summarized in this scheme, amine 35 is formed in good yields consistently over four additional cycles, with an average input of only 600 ppm (i.e., 0.06 mol%) of catalyst C10 per coupling.
To document the applicability of this aqueous coupling chemistry, direct comparisons were made by focusing on six key intermediates associated with medicinally relevant natural products or APIs (Scheme 5A).17 Aminations that arrive at key intermediates 35–40 reveal that this methodology operates at much lower catalyst loadings and temperatures, and is typically faster than reactions in organic media. Moreover, yields tend to be comparable to, or better than, those reported previously. Another recent example providing further testimony to the potential savings of precious metal is shown in Scheme 5B.18 Amination at C-2 in a 3-substituted estrone derivative afforded product 42 using 40 times less palladium and ligand (10 mol% = 100
000 ppm), run at a lower temperature (45 vs. 110 °C), and in less time (16 vs. 24 h). The level of residual Pd in the product from both approaches led to the expected outcomes: from amination in toluene: 165 ppm Pd, while from this ppm level amination in water: 3 ppm Pd.
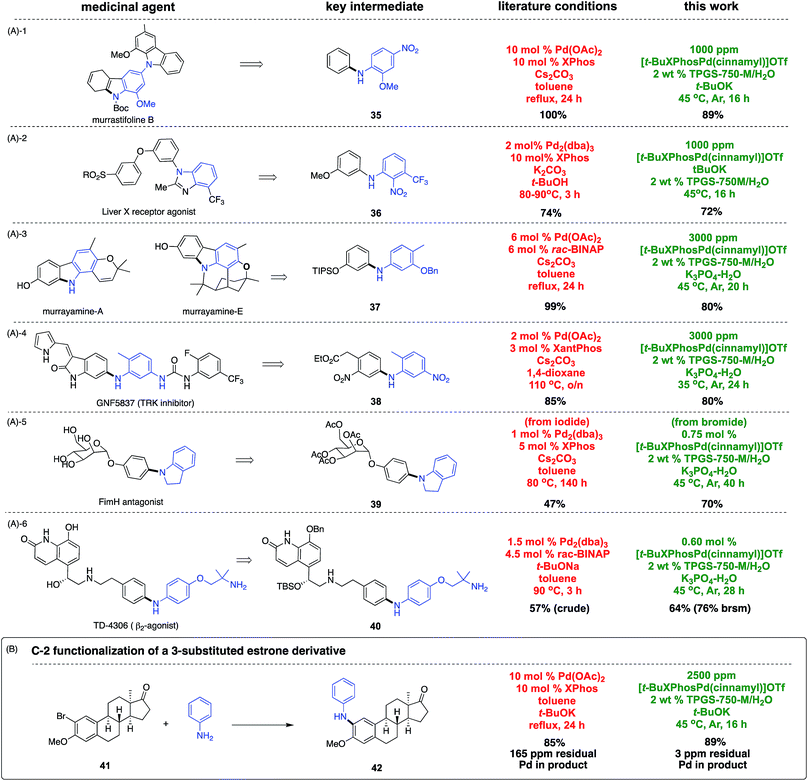 |
| Scheme 5 (A) Direct comparisons between literature conditions and those using ppm level Pd catalysis in water. (B) Comparison with recent amination of an estrone derivative. Yields refer to isolated material. | |
Conclusions
Overall, this work provides a significant advance in Pd-catalyzed C–N cross-coupling chemistry. Specifically, it offers the synthetic community, based on a known and well defined 1
:
1 Pd
:
L complex, aminations that: (1) rely on ppm levels of palladium; (2) are glove-box-free and operationally simple; (3) are general, highly efficient, and offer gram-scale capability; (4) perform under very mild conditions, with temperatures at 45 °C or below; (5) utilize an aqueous reaction medium that avoids organic solvents, yet involve very little water; (6) allow for recycling of the surfactant, water, and catalyst; and (7) lead to ppm levels of residual palladium in the amination products. The process further illustrates the synthetic potential of pre-complexing a lipophilic ligand with Pd that can lead to pre-catalyst loadings at the ppm level. Extending the current technology to additional C–N cross couplings as well as use of Fe/ppm Pd nanoparticles8a for similar synthetic purposes are currently under study.
Conflicts of interest
There are no conflicts to declare.
Acknowledgements
Financial support provided by Novartis and the NSF (GOALI 1566212) is warmly acknowledged. The palladium used in this study was generously supplied by Dr Thomas Colacot, formerly at Johnson Matthey. Y. Z. would like to express thanks to Prof. David M. Chenoweth for the inspiring mentoring in the past.
Notes and references
-
(a)
A. DeAngelis and T. J. Colacot, in New Trends in Cross-Coupling: Theory and Applications, The Royal Society of Chemistry, London, 2015, pp. 20–90 Search PubMed;
(b)
C. C. C. Johansson Seechurn, H. Li and T. J. Colacot, in New Trends in Cross-Coupling: Theory and Applications, The Royal Society of Chemistry, London, 2015, pp. 91–138 Search PubMed;
(c) Q. Yao, E. P. Kinney and C. Zheng, Org. Lett., 2004, 6, 2997 CrossRef CAS PubMed;
(d) N. T. S. Phan, M. Van Der Sluys and C. W. Jones, Adv. Synth. Catal., 2006, 348, 609 CrossRef CAS.
-
(a) T. J. Colacot, Platinum Met. Rev., 2011, 55, 84 CrossRef;
(b) C. C. C. Johansson Seechurn, M. O. Kitching, T. J. Colacot and V. Snieckus, Angew. Chem. Int. Ed., 2012, 51, 5062 (
Angew. Chem.
, 2012
, 124
, 5150
) CrossRef CAS PubMed;
(c)
G. Molander, J. P. Wolfe and M. Lerhed, Science of synthesis: cross-coupling and Heck-type reactions, Thieme, Stuttgart, Workbench edn, 2013 Search PubMed;
(d) P. G. Gildner and T. J. Colacot, Organometallics, 2015, 34, 5497 CrossRef CAS.
-
(a) B. Schlummer and U. Scholz, Adv. Synth. Catal., 2004, 346, 1599 CrossRef CAS;
(b) J.-P. Corbet and G. Mignani, Chem. Rev., 2006, 106, 2651 CrossRef CAS PubMed;
(c) C. Torborg and M. Beller, Adv. Synth. Catal., 2009, 351, 3027 CrossRef CAS;
(d) M. M. Heravi, Z. Kheilkordi, V. Zadsirjan, M. Heydari and M. J. Malmir, Organomet. Chem., 2018, 861, 17 CrossRef CAS.
-
(a) J. F. Hartwig, Nature, 2008, 455, 314 CrossRef CAS PubMed;
(b) D. S. Surry and S. L. Buchwald, Chem. Sci., 2011, 2, 27 RSC;
(c) R. J. Lundgren and M. Stradiotto, Chem.–Eur. J., 2012, 18, 9758 CrossRef CAS PubMed;
(d) J. Bariwal and E. Van der Eycken, Chem. Soc. Rev., 2013, 42, 9283 RSC;
(e) P. Ruiz-Castillo and S. L. Buchwald, Chem. Rev., 2016, 116, 12564 CrossRef CAS PubMed.
-
(a) J. P. Wolfe, S. Wagaw, J.-F. Marcoux and S. L. Buchwald, Acc. Chem. Res., 1998, 31, 805 CrossRef CAS;
(b) J. F. Hartwig, Angew. Chem., Int. Ed., 1998, 37, 2046 CrossRef CAS;
(c) E. A. B. Kantchev, C. J. O'Brien and M. G. Organ, Angew. Chem., Int. Ed., 2007, 46, 2768 (
Angew. Chem.
, 2007
, 119
, 2824
) CrossRef CAS PubMed;
(d) D. S. Surry and S. L. Buchwald, Angew. Chem., Int. Ed., 2008, 47, 6338 (
Angew. Chem.
, 2008
, 120
, 6438
) CrossRef CAS PubMed;
(e) S. Díez-González, N. Marion and S. P. Nolan, Chem. Rev., 2009, 109, 3612 CrossRef PubMed;
(f) C. A. Fleckenstein and H. Plenio, Chem. Soc. Rev., 2010, 39, 694 RSC;
(g) G. C. Fortman and S. P. Nolan, Chem. Soc. Rev., 2011, 40, 5151 RSC;
(h) B. J. Tardiff, R. McDonald, M. J. Ferguson and M. Stradiotto, J. Org. Chem., 2012, 77, 1056 CrossRef CAS PubMed;
(i) S. M. Crawford, C. B. Lavery and M. Stradiotto, Chem.–Eur. J., 2013, 19, 16760 CrossRef CAS PubMed;
(j) M. Su, N. Hoshiya and S. L. Buchwald, Org. Lett., 2014, 16, 832 CrossRef CAS PubMed;
(k) F. Inoue, M. Kashihara, M. R. Yadav and Y. Nakao, Angew. Chem. Int. Ed., 2017, 56, 13307 (
Angew. Chem.
, 2017
, 129
, 13492
) CrossRef CAS PubMed;
(l) J. Richardson, J. C. Ruble, E. A. Love and S. Berritt, J. Org. Chem., 2017, 82, 3741 CrossRef CAS PubMed;
(m) X.-B. Lan, Y. Li, Y.-F. Li, D.-S. Shen, Z. Ke and F.-S. Liu, J. Org. Chem., 2017, 82, 2914 CrossRef CAS PubMed;
(n) J. Mao, J. Zhang, S. Zhang and P. J. Walsh, Dalton Trans., 2018, 47, 8690 RSC;
(o) J. M. Dennis, N. A. White, R. Y. Liu and S. L. Buchwald, J. Am. Chem. Soc., 2018, 140, 4721 CrossRef CAS PubMed . For a (phosphine or NHC) ligandless, intramolecular approach, see T.-S. Mei, X. Wang and J.-Q. Yu, J. Am. Chem. Soc., 2009, 131, 10806.
-
(a) D. Zim and S. L. Buchwald, Org. Lett., 2003, 5, 2413 CrossRef CAS PubMed;
(b) N. Marion and S. P. Nolan, Acc. Chem. Res., 2008, 41, 1440 CrossRef CAS PubMed;
(c) N. R. Biscoe, B. P. Fors and S. L. Buchwald, J. Am. Chem. Soc., 2008, 130, 6686 CrossRef PubMed;
(d) C. C. C. Johansson Seechurn, S. L. Parisel and T. J. Colacot, J. Org. Chem., 2011, 76, 7918 CrossRef CAS PubMed;
(e) C. Valente, M. Pompeo, M. Sayah and M. G. Organ, Org. Process Res. Dev., 2014, 18, 180 CrossRef CAS;
(f) A. J. DeAngelis, P. G. Gildner, R. Chow and T. J. Colacot, J. Org. Chem., 2015, 80, 6794 CrossRef CAS PubMed.
-
(a) J. F. Hartwig, Acc. Chem. Res., 2008, 41, 1534 CrossRef CAS PubMed;
(b) N. C. Bruno, M. T. Tudge and S. L. Buchwald, Chem. Sci., 2013, 4, 916 RSC;
(c) N. C. Bruno and S. L. Buchwald, Org. Lett., 2013, 15, 2876 CrossRef CAS PubMed;
(d) N. C. Bruno, N. Niljianskul and S. L. Buchwald, J. Org. Chem., 2014, 79, 4161 CrossRef CAS PubMed;
(e) For a review, see A. Bruneau, M. Roche, M. Alami and S. Messaoudi, ACS Catal., 2015, 5, 1386 CrossRef CAS.
- For prior studies on sustainable metal-catalyzed transformations by us, see
(a) S. Handa, Y. Wang, F. Gallou and B. H. Lipshutz, Science, 2015, 349, 1087 CrossRef CAS PubMed;
(b) S. Handa, M. P. Andersson, F. Gallou, J. Reilly and B. H. Lipshutz, Angew. Chem. Int. Ed., 2016, 55, 4914 (
Angew. Chem.
, 2016
, 128
, 4998
) CrossRef CAS PubMed;
(c) J. Feng, S. Handa, F. Gallou and B. H. Lipshutz, Angew. Chem. Int. Ed., 2016, 55, 8979 (
Angew. Chem.
, 2016
, 128
, 9125
) CrossRef CAS PubMed;
(d) C. M. Gabriel, M. Parmentier, C. Riegert, M. Lanz, S. Handa, B. H. Lipshutz and F. Gallou, Org. Process Res. Dev., 2017, 21, 247 CrossRef CAS;
(e) S. Handa, J. D. Smith, M. S. Hageman, M. Gonzalez and B. H. Lipshutz, ACS Catal., 2016, 6, 8179 CrossRef CAS;
(f) P. Klumphu, C. Desfeux, Y. Zhang, S. Handa, F. Gallou and B. H. Lipshutz, Chem. Sci., 2017, 8, 6354 RSC;
(g) N. R. Lee, A. A. Bikovtseva, M. Cortes-Clerget, F. Gallou and B. H. Lipshutz, Org. Lett., 2018, 19, 6518 CrossRef PubMed;
(h) S. Handa, J. B. Smith, Y. Zhang, T. Balaram, F. Gallou and B. H. Lipshutz, Org. Lett., 2018, 20, 542 CrossRef CAS PubMed.
-
(a) B. H. Lipshutz, D. W. Chung and B. Rich, Adv. Synth. Catal., 2009, 351, 1717 CrossRef CAS PubMed;
(b) B. J. Tardiff and M. Stadiotto, Eur. J. Org. Chem., 2012, 21, 3972 CrossRef;
(c) N. A. Isley, S. Dobarco and B. H. Lipshutz, Green Chem., 2014, 16, 1480 RSC;
(d) C. Salomé, P. Wagner, M. Bollenbach, F. Bihel, J.-J. Bourguignon and M. Schmitt, Tetrahedron, 2014, 70, 3413 CrossRef;
(e) P. Wagner, M. Bollenbach, C. Doebelin, F. Bihel, J.-J. Bourguignon, C. Salomé and M. Schmitt, Green Chem., 2014, 16, 4170 RSC.
-
(a) E. Davies and R. Renner, Chem. World, 2011, 1, 50 Search PubMed;
(b) P. Mike, New Sci., 2011, 2, 2799 Search PubMed.
- See
(a) H. Li, C. C. C. J. Seechurn and T. J. Colacot, ACS Catal., 2012, 2, 1147 CrossRef CAS and references therein;
(b) C. Amatore, G. Broeker, A. Jutland and F. Khalil, J. Am. Chem. Soc., 1997, 119, 5176 CrossRef CAS;
(c) I. J. S. Fairlamb, A. R. Kapdi and A. F. Lee, Org. Lett., 2004, 6, 4435 CrossRef CAS PubMed;
(d) C. S. Wei, G. H. M. Davies, O. Soltani, J. Albrecht, Q. Gao, C. Pathirana, Y. Hsiao, S. Tummala and M. D. Eastgate, Angew. Chem., Int. Ed., 2013, 52, 5822 CrossRef CAS PubMed.
-
(a) P. S. Kutchukian, J. F. Dropinski, K. D. Dykstra, B. Li, D. A. DiRocco, E. C. Streckfuss, L.-C. Campeau, T. Cernak, P. Vachal, I. W. Davies, S. W. Krska and S. D. Dreher, Chem. Sci., 2016, 7, 2604 RSC;
(b) M. R. Uehling, R. P. King, S. W. Krska, T. Cernak and S. L. Buchwald, Science, 2019, 363, 405 CrossRef CAS PubMed.
- Other compounds from the Merck informer library, such as X1 (Sigma catalog 901753), X14 (Sigma catalog 901749), and X17 (Sigma catalog 901753) failed to give the desired products of amination. Rather, the presence of readily hydrolysable functionality (e.g., esters, lactones, carbamates, etc.) present in each appeared to be the major side reactions. This was not unexpected in light of the recent work by Merck and co-workers that required pre-formed Pd(II) intermediates arrived at using stoichiometric levels of palladium; see ref. 12b.
- [t-BuXPhos(Pd-π-allyl)]OTf is commercially available through Johnson Matthey (catalogue number: Pd-174). The other two analogues, [t-BuXPhos(Pd-π-crotyl)]OTf and [t-BuXPhos(Pd-π-cinnamyl)]OTf can be prepared following a two-step sequence from ready available material, as described in ref. 6f.
- Treatment of the waste water associated with use of aqueous TPGS-750-M has been fully addressed at Novartis, the procedure having been first disclosed at the recent Workshop on Chemistry in Water, UCSB June–July, 2019. This study was alluded to in an earlier report by the same group in Basel (i.e., Dr Fabrice Gallou); see F. Gallou, N. A. Isley, A. Ganic, U. Onken, M. Parmentier, Green Chem., 2016, 18, 14. Details will be disclosed in a forthcoming publication by Novartis.
-
F.D.A., Q3D Elemental Impurities Guidance for Industry, https://www.fda.gov/downloads/drugs/guidances/ucm371025.pdf Search PubMed.
-
(a) C. Börger, A. W. Schmidt and H.-J. Knölker, Synlett, 2014, 25, 1381 CrossRef;
(b) J. M. Travins, R. C. Bernotas, D. H. Kaufman, E. Quinet, P. Nambi, I. Feingold, C. Huselton, A. Wilhelmsson, A. Goos-Nilsson and J. Wrobel, Bioorg. Med. Chem. Lett., 2010, 20, 526 CrossRef CAS PubMed;
(c) C. Schuster, K. K. Julich-Gruner, H. Schnitzler, R. Hesse, A. Jäger, A. W. Schmidt and H.-J. Knölker, J. Org. Chem., 2015, 80, 5666 CrossRef CAS PubMed;
(d) P. Albaugh, Y. Fan, Y. Mi, F. Sun, F. Adrian, N. Li, Y. Jia, Y. Sarkisova, A. Kreusch, T. Hood, M. Lu, G. Liu, S. Huang, Z. Liu, J. Loren, T. Tuntland, D. S. Karanewsky, H. M. Seidel and V. Molteni, ACS Med. Chem. Lett., 2012, 3, 140 CrossRef CAS PubMed;
(e) X. Jiang, D. Abgottspon, S. Kleeb, S. Rabbani, M. Scharenberg, M. Wittwer, M. Haug, O. Schwardt and B. Ernst, J. Med. Chem., 2012, 55, 4700 CrossRef CAS PubMed;
(f) R. M. McKinnell, U. Klein, M. S. Linsell, E. J. Moran, M. B. Nodwell, J. W. Pfeiffer, R. Thomas, C. Yu and J. R. Jacobsen, Bioorg. Med. Chem. Lett., 2014, 24, 2871 CrossRef CAS PubMed.
- I. Bacsa, D. Szemerédi, J. Wölfling, G. Schneider, L. Fekete and E. Mernyák, Beilstein J. Org. Chem., 2018, 14, 998 CrossRef CAS PubMed.
Footnote |
† Electronic supplementary information (ESI) available. See DOI: 10.1039/c9sc03710a |
|
This journal is © The Royal Society of Chemistry 2019 |