DOI:
10.1039/C9SC03672E
(Edge Article)
Chem. Sci., 2019,
10, 10089-10096
Rhodium(I)-catalyzed C6-selective C–H alkenylation and polyenylation of 2-pyridones with alkenyl and conjugated polyenyl carboxylic acids†
Received
24th July 2019
, Accepted 9th September 2019
First published on 9th September 2019
Abstract
A versatile Rh(I)-catalyzed C6-selective decarbonylative C–H alkenylation of 2-pyridones with readily available, and inexpensive alkenyl carboxylic acids has been developed. This directed dehydrogenative cross-coupling reaction affords 6-alkenylated 2-pyridones that would otherwise be difficult to access using conventional C–H functionalization protocols. The reaction occurs with high efficiency and is tolerant of a broad range of functional groups. A wide scope of alkenyl carboxylic acids, including challenging conjugated polyene carboxylic acids, are amenable to this transformation and no addition of external oxidant is required. Mechanistic studies revealed that (1) Boc2O acts as the activator for the in situ transformation of the carboxylic acids into anhydrides before oxidative addition by the Rh catalyst, (2) a decarbonylation step is involved in the catalytic cycle, and (3) the C–H bond cleavage is likely the turnover-limiting step.
Introduction
The 2-pyridone motif is found in numerous naturally occurring molecules and synthetic organic compounds that possess a broad spectrum of bioactivities.1 For example, A58365A, isolated from the fermentation broth of a soil bacterium, serves as an angiotensin-converting enzyme inhibitor;1f fredericamycin A, isolated from Streptomyces griseus, is a potent antitumor antibiotic;1g ciclopirox is a widely used synthetic antifungal agent;1h and milrinone is a phosphodiesterase 3 inhibitor used to treat heart failure (Fig. 1).1i 2-Pyridones are also valued as building blocks, because they can be converted to pyridines, piperidines, quinolizidines and indolizidines.1j As a result of their widespread utility, the construction of 2-pyridones has been a vibrant research area in the synthetic community, and numerous methods for their synthesis are available.2,3
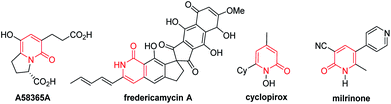 |
| Fig. 1 Biologically active 2(1H)-pyridone molecules. | |
Several approaches for the functionalization of 2-pyridones have employed transition metals. Early studies focused on transition-metal catalyzed cross-coupling of functionalized 2-pyridones.4 More recent efforts to elaborate the 2-pyridone motif have been devoted to their direct catalytic C–H functionalization.2b,c In this context, rapid progress in site-selective C–H functionalization at C3, C5 and C6 positions of 2-pyridones has been advanced.5–8 Notably, Miura and co-workers found that the use of easily attachable and detachable 2-pyridyl directing groups at the nitrogen of the 2-pyridones could effectively facilitate the copper-mediated C6-selective dehydrogenative heteroarylation with 1,3-azoles.7b Following this seminal work, transition-metal catalyzed directed alkynylation,6d arylation,7h,j,o alkylation,7d,n,w,x borylation,7g,m thiolation,7i annulation,7e,f,p,r allylation,7l,q and amidation7t–v of 1-(2-pyridyl)-2-pyridones at the C6 positions have been successfully accomplished. In general, installation of vinyl groups has proven considerably more challenging than aryl or alkyl substituents, and this holds true for the vinylation of 2-pyridones at the C-6 position. Nakao and co-workers reported an impressive C6-alkenylation of 2-pyridones via C–H hydroarylation of N-alkylated 2-pyriodnes with alkynes at the C6 position under Ni/Al cooperative catalysis, albeit with limited substrate scope and low functional group tolerance (Scheme 1a).8a Very recently, the group of Hirano and Miura reported Rh(III)-catalyzed (10 mol%) C6-selective alkenylation of 1-(2-pyridyl)-2-pyridones with acrylates and styrenes (Scheme 1b).8b
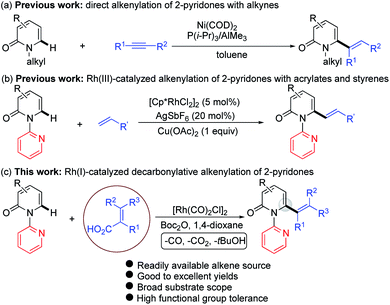 |
| Scheme 1 Catalytic direct C–H alkenylation of 2-pyridones at the C6 position: (a) alkenylation with alkynes, (b) alkenylation with acrylates and styrenes, and (c) decarbonylative alkenylation. | |
Recently, the use of readily available and inexpensive α,β-unsaturated carboxylic acids in transition metal catalyzed decarboxylative and decarbonylative alkenylation reactions has gained attention.9–11 We envisioned that 6-alkenylated 2-pyridones might be accessible from 1-(2-pyridyl)-2-pyridones and α,β-unsaturated acids under transition metal catalysis. In connection with our ongoing interests in direct alkenylation of C–H bonds,12 herein we report a Rh(I)-catalyzed C6-selective C–H alkenylation of 2-pyridones using alkenyl carboxylic acids as the vinyl source (Scheme 1c). This protocol features a simple and easy-to-handle catalytic system, high efficiency, very broad substrate scope and high functional group tolerance.
Results and discussion
Recent studies have revealed that catalytic systems based on Rh(III), Ru(II) and Pd(II) complexes perform well in directed alkenylation of relatively inert (hetero)arene and alkene C–H bonds.13 Inspired by these reports, we first attempted the alkenylation of the model substrate 1-(2-pyridyl)-2-pyridone (1a) with styrene using Rh(III), Ru(II) and Pd(II) complexes (ESI, Table S1†). Unfortunately, various catalytic systems, including those that have been shown to efficiently catalyze direct alkenylation of structurally similar 2-phenylpyrimidines, 1-(pyrimidin-2-yl)-1H-indoles and 2-(1H-pyrrol-1-yl)pyrimidines,14 did not furnish the desired products (Scheme 2a). Liu and co-workers recently described Rh(III)-catalyzed site-selective C–H alkylation and arylation of 1-(2-pyridyl)-2-pyridones at the C6 position with potassium trifluoroborates.7h Expanding the substrate scope of this reaction to include potassium vinyl trifluoroborates, however, was unsuccessful in our hands using a similar Rh(III) catalyst (Scheme 2b and ESI, Table S2†). Likewise, Ru(II)-catalyzed alkenylation of 1a with styrylboronic acids did not afford the desired alkenylation product (Scheme 2c and ESI, Table S2†).7o
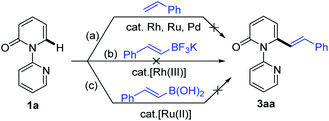 |
| Scheme 2 Unsuccessful catalytic direct alkenylations of 1-(2-pyridyl)-2-pyridone (1a). | |
We then turned our attention to the coupling reaction of vinyl carboxylic acids with 2-pyridones. We were pleased to discover that the reaction of 1a and trans-cinnamic acid (2a) in the presence of [Rh(CO)2Cl]2 (1.0 mol%) and Boc2O (1.5 equiv.) at 130 °C in 1,4-dioxane, provided the desired product 3aa in 92% yield after 6 h (Table 1, entry 1). A solvent screen revealed that 1,4-dioxane outperformed other frequently employed solvents, such as toluene, PhCl, p-xylene, THF, CH3CN, DCE, DMF and DME (Table 1, entries 2–9). Changing the rhodium source to [Rh(COD)Cl]2, [RhCl(PPh3)3], [Rh(COD)2BF4], or [Cp*RhCl2]2, did not lead to any improvement in the yield of 3aa (Table 1, entries 10–13). Other transition metal complexes such as [Ru(p-cymene)Cl2]2, [Cp*IrCl2]2 and Pd(OAc)2 were also ineffective in this transformation (Table 1, entries 14–16).
Table 1 Optimization of the reaction conditionsa
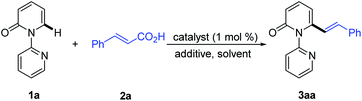
|
Reaction conditions: 1a (0.2 mmol), 2a (0.22 mmol), catalyst (1.0 mol%), activator (1.5 equiv.), solvent (2.0 mL), 130 °C, 6 h, in air.
Isolated yield.
Reaction temperature 120 °C.
[Rh(CO)2Cl]2 (0.5 mol%) was used.
1-(Pyrimidin-2-yl)pyridin-2(1H)-one was employed.
|
Entry |
Catalyst |
Activator |
Solvent |
Yield (%)b |
1 |
[Rh(CO)2Cl]2 |
Boc2O |
1,4-Dioxane |
93 |
2 |
[Rh(CO)2Cl]2 |
Boc2O |
Toluene |
15 |
3 |
[Rh(CO)2Cl]2 |
Boc2O |
PhCl |
11 |
4 |
[Rh(CO)2Cl]2 |
Boc2O |
p-Xylene |
15 |
5 |
[Rh(CO)2Cl]2 |
Boc2O |
THF |
NR |
6 |
[Rh(CO)2Cl]2 |
Boc2O |
CH3CN |
NR |
7 |
[Rh(CO)2Cl]2 |
Boc2O |
DCE |
10 |
8 |
[Rh(CO)2Cl]2 |
Boc2O |
DMF |
NR |
9 |
[Rh(CO)2Cl]2 |
Boc2O |
DME |
NR |
10 |
[Rh(COD)Cl]2 |
Boc2O |
1,4-Dioxane |
<5 |
11 |
[RhCl(PPh3)3] |
Boc2O |
1,4-Dioxane |
NR |
12 |
[Rh(COD)2BF4] |
Boc2O |
1,4-Dioxane |
NR |
13 |
[Cp*RhCl2]2 |
Boc2O |
1,4-Dioxane |
NR |
14 |
[Ru(p-cymene)2Cl2] |
Boc2O |
1,4-Dioxane |
NR |
15 |
[Cp*IrCl2]2 |
Boc2O |
1,4-Dioxane |
NR |
16 |
Pd(OAc)2 |
Boc2O |
1,4-Dioxane |
NR |
17 |
[Rh(CO)2Cl]2 |
(MeOCO)2O |
1,4-Dioxane |
22 |
18 |
[Rh(CO)2Cl]2 |
Tf2O |
1,4-Dioxane |
NR |
19 |
[Rh(CO)2Cl]2 |
(CF3CO)2O |
1,4-Dioxane |
NR |
20 |
[Rh(CO)2Cl]2 |
PivCl |
1,4-Dioxane |
39 |
21 |
[Rh(CO)2Cl]2 |
Piv2O |
1,4-Dioxane |
92 |
22c |
[Rh(CO)2Cl]2 |
Boc2O |
1,4-Dioxane |
55 |
23d |
[Rh(CO)2Cl]2 |
Boc2O |
1,4-Dioxane |
43 |
24 |
None |
Boc2O |
1,4-Dioxane |
NR |
25 |
[Rh(CO)2Cl]2 |
None |
1,4-Dioxane |
NR |
26e |
[Rh(CO)2Cl]2 |
Boc2O |
1,4-Dioxane |
31 |
We next screened different electrophiles to activate the unsaturated acid. Poor conversion was obtained with (MeOCO)2O (22%), Tf2O (NR), (CF3CO)2O (NR), or PivCl (39%) as the acid activators (Table 1, entries 17–20). In contrast, Piv2O was effective and gave 3aa in 92% yield (Table 1, entry 21). Considering the price and compatibility, however, more economical and milder Boc2O was preferred.
Further optimization involving decreasing the reaction temperature or the catalyst loading led to dramatically lowered yields (Table 1, entries 22 and 23). Notably, the reaction did not proceed in the absence of either a rhodium catalyst or acid activator (Table 1, entries 24 and 25). Finally, the effect of the N-directing group in this reaction was examined. No reaction occurred when free 2-pyridone or 2-pyridone substrates bearing other substituents on the nitrogen, such as Me, Bn, Ph, or 3-pyridyl. The 2-pyrimidyl resulted in only 31% yield (Table 1, entry 26). These results clearly indicated that the judicious choice of the N-directing group is critical for catalysis in this transformation.
With the optimized conditions in hand, we investigated the scope of 1-(2-pyridyl)-2-pyridones with 2a as the coupling partner (Table 2). It was found that a series of C3- and C4-substituted 1-(2-pyridyl)-2-pyridones (1b–1l) underwent smooth alkenylation with 2a exclusively at the C6-position to deliver the corresponding products (3ba–3la) in good to excellent yields (77–91%) with high tolerance of functional groups, including halides at the 3- or 4-positions. Notably, the C5-substituted 2-pyridones (1m–1q) were compatible with our Rh-catalyzed system to afford the C6-alkenylated products (3ma–3qa) in 67–83% yield, despite the increased steric hindrance on C5. The 3,4-disubstituted 2-pyridones (1r and 1s) were also readily engaged under the current conditions to give the corresponding products (3ra and 3sa) in 75 and 63% yields, respectively. Substrates bearing electron donating or electron withdrawing substituents on the pyridyl rings (1t–1v) coupled smoothly with 2a to generate the desired products (3ta–3va) in 83–89% yields. Moreover, this reaction could be readily extended to 4H-[1,2′-bipyridin]-4-one (1w) and 1-(pyridin-2-yl)quinolin-4(1H)-one (1x), thus producing 3wa and 3xa in 88 and 91% yields, respectively. It is notable that only the formation of the dialkenylated product was observed in the case of 1w.
Table 2 Catalytic alkenylation of various 2-pyridones with 2aa,b
Reaction conditions: 1a (0.2 mmol), 2a (0.22 mmol), [Rh(CO)2Cl]2 (1.0 mol%), Boc2O (1.5 equiv.), 1,4-dioxane (2.0 mL), 130 °C, 6 h, in air.
Isolated yield.
2a (0.44 mmol) was employed.
|
|
Subsequently, we explored the reactivity of various cinnamic acids with 1a. As shown in Table 3, a wide range of cinnamic acids (2b–2p) with mono-substituted aromatic rings efficiently participated in the alkenylation with 1a to exclusively furnish the desired C6-alkenlayed 2-pyridone products (3ab–3ap) in good to excellent yields (77–92%). The alkenylation proved to be insensitive to the nature of the substituents on the aryl ring, with various electron-withdrawing (NO2, CO2Me and CN) and donating substituents (alkyl, OMe and NMe2) participating. Sensitive functional groups, including OH, B(OH)2, and halogens, were all well tolerated. The structure of 3ap was confirmed by single-crystal X-ray diffraction (CCDC 1874166). Similarly, the more complex cinnamic acids (2q–2v), with polysubstituted aromatic rings, displayed good reactivity, affording the target products (3aq–3av) in 70–90% yields. Notably, a vinyl group bearing a pentafluoro phenyl provided the product (3au) in 70% yield. Heteroaryl groups are vital substructures in medicinal chemistry.15 We, therefore, examined the compatibility of heteroaryl cinnamic acids with 1a. Heteroaryl cinnamic acids bearing 3-pyridyl, 2-furanyl, and 2-thiofuranyl (2w–2y) reacted smoothly with 1a to give the desired products (3aw–3ay) in 82–90% yields. Importantly, the estrone-derived cinnamic acid 2z proved to be equally effective in this transformation, indicating the robustness of the current catalytic system.
Table 3 Direct olefination of 1a with cinnamic acidsa,b
Reaction conditions: 1a (0.2 mmol), 2a (0.22 mmol), [Rh(CO)2Cl]2 (1.0 mol%), Boc2O (1.5 equiv.), 1,4-dioxane (2.0 mL), 130 °C, 6 h, in air.
Isolated yield.
|
|
To further demonstrate the potential of our catalytic system, the reaction was extended to other substituted alkenyl carboxylic acids, and the results are summarized in Table 4. It was found that treatment of various β-alkylated acrylic acids (4a–4d) with 1a resulted in exclusive formation of C6-alkenylated 2-pyridone products (5aa–5ad) in 85–92% yields, irrespective of the nature of the β-alkyl groups. In the case of acid 4e containing a sensitive Cl group, the reaction furnished the desired product 5ae in 87% yield without dechlorination. Notably, the simple acrylic acid (4f) was also reactive, giving rise to the C6-vinylated 2-pyridone product 5af in 75% yield. Likewise, the α-substituted acrylic acids 4g and 4h were competent substrates, delivering 5ag and 5ah both in 80% yield. Furthermore, trisubstituted acrylic acids (4i–4n), including the naturally occurring geranic acid (4k), shikimic acid (4m) and perillic acid (4n), were good substrates, producing 5ai–5an in 63–91% yields. Potentially reactive groups, like OH and C
C, were not detrimental to the overall yields. Remarkably, a variety of conjugated polyene carboxylic acids were also efficient coupling partners in this transformation. More substituted and less sensitive conjugated dienyl carboxylic acids (4o–4s) formed the desired products (5ao–5as) in 65–86% yields. The formation of a mixture of Z/E isomers in the case of 5ar was due to the low stereochemical purity of the starting trienoic acid 4r (4Z/4E ratio 1
:
1). Surprisingly, both the bioactive retinoic acid (4t) and its derivative 4u containing a conjugated hexaene unit, formed the corresponding products (5at and 5au) in 72% and 65% yields, respectively. Application of 5-phenylpent-2-en-4-ynoic acid (4v) led to the formation of 5av in 67% yield, with the alkyne having no obvious adverse effect on the reaction outcome.
Table 4 Direct olefination of 1a with substituted alkenyl carboxylic acidsa,b
Reaction conditions: 1a (0.2 mmol), 2a (0.22 mmol), [Rh(CO)2Cl]2 (1.0 mol%), Boc2O (1.5 equiv.), 1,4-dioxane (2.0 mL), 130 °C, 6 h, in air.
Isolated yield.
Ratio of isomers (E/Z).
|
|
In order to explore the synthetic practicality of this transformation, a gram scale reaction of 1a and 2a was performed to deliver 3aa in 88% yield (Scheme 3a). Further transformations of the products were then explored. As depicted in Scheme 3b, hydrogenation of 3aa at room temperature favored the reduction of the alkene moiety to generate the C6-alkylated 2-pyridone product 6 in 84% yield. Increasing the reaction temperature to 50 °C, however, enabled formation of piperidin-2-one product 7 (92% yield). The pyridine directing group could be conveniently removed by treatment with MeOTf and KOtBu to give the C6-alkenylated 2-pyridone products in 68–73% yield (Scheme 3c).7h
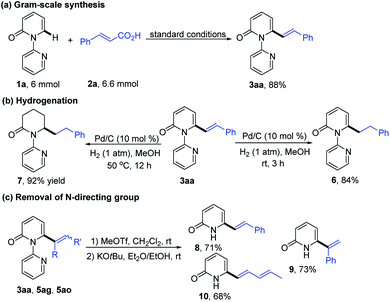 |
| Scheme 3 Synthetic applications: (a) gram-scale synthesis, (b) hydrogenation, and (c) deprotection. | |
We next desired to probe the basic steps of the reaction mechanism. Activation of the carboxylic acid was envisioned to proceed via an anhydride derivative.16 To test this hypothesis, a control experiment with cinnamic anhydride 11 and 1a demonstrated that the coupling worked equally (91% yield) as well as acid 2a with Boc2O (93% yield). Treatment of acid 2a with an equimolar amount of Boc2O in 1,4-dioxane at 130 °C for 6 h led to the predominant formation of cinnamic anhydride 11 in 85% yield. This observation supports the involvement of in situ generation of the anhydride in the vinylation reaction.16 The generation of CO gas during the reaction was confirmed by analyzing the head gas of the reaction mixture with GC-TDC (ESI, Fig. S1†). Moreover, employing [Rh(COD)Cl]2 as the catalyst also generated CO gas albeit with a longer reaction time (18 h) and lower yield of 3aa (50%) (ESI, Fig. S2†). These results rule out the possibility that CO gas might be derived from [Rh(CO)2Cl]2, thus indicating the presence of a decarbonylation step in the catalytic cycle. As shown in Scheme 4b, treatment of 1a with D2O (5 equiv.) under the standard conditions for 1 h, in the absence or presence of 2a resulted in approximately 38% and 27% deuteration at the C6-position, respectively, suggesting the reversibility of the C–H activation step under these conditions.
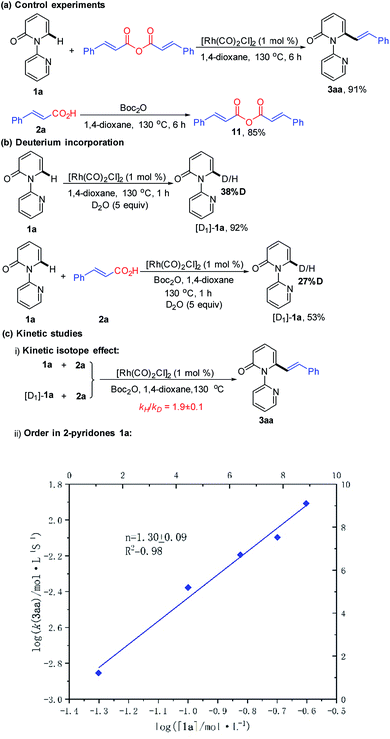 |
| Scheme 4 Mechanistic studies: (a) control experiments, (b) deuterium incorporation, and (c) kinetic studies. | |
To gain insight into the turnover-limiting step, we conducted initial rate studies and a parallel kinetic isotope effect (KIE) on 1a. The kinetic analyses highlighted a first-order (n = 1.30 ± 0.09) dependence on the concentration of 1a for the reaction (Scheme 4c and ESI†). In separate reaction vessels, 1a and [D1]-1a were subjected to identical reaction conditions (ESI†); it was observed that 1a was alkenylated to 3aa at a greater rate than the corresponding deuterium-labelled substrate. The KIE value determined from the average of five runs via the method of initial rates was 1.9 ± 0.1. This result implies that the C–H bond cleavage is likely involved in the turnover-limiting step.
Based on the aforementioned results and literature precedence,17 a plausible mechanism highlighting the key steps is presented in Scheme 5. First, solvent (S) or the substrate pyridine breaks up the dimer [Rh(CO)2Cl]2 to give the monomer and enter the catalytic cycle. Meanwhile, the acid reacts with Boc2O to generate the anhydride, which undergoes oxidative addition to a Rh(I) species A and leads to the formation of the Rh(III) intermediate B. In the event that S is solvent, ligand exchange for the substrate follows, giving intermediate C. Rather than a second oxidative addition, we prefer a concerted metalation deprotonation (CMD) by the carboxylate ligand via transition state D to generate the acid and the cyclometallated species with the key Rh–C bond. The liberated acid can react with the Boc2O to re-enter the cycle as the anhydride. E is envisioned to undergo loss of coordinated CO and then deinsertion of CO to afford the Rh–vinyl intermediate. Reductive elimination regenerates Rh(I) with the bound product G, which undergoes exchange with the solvent to liberate the product and close the catalytic cycle to form A. At this point, the exact ordering of the steps remains to be determined.
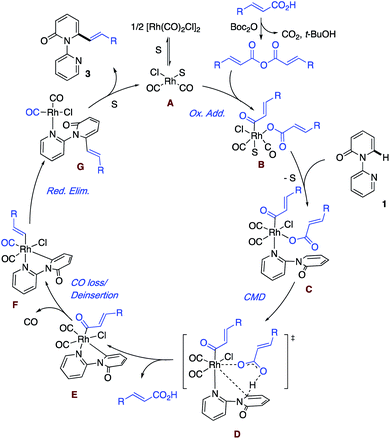 |
| Scheme 5 Plausible mechanism. | |
Conclusions
We have developed the first Rh(I)-catalyzed decarbonylative alkenylation at C6 of 2-pyridones using readily available and inexpensive alkenyl carboxylic acids. This C6 alkenylation of 2-pyridones is applicable to the coupling of a wide range of substituted acyclic acids and conjugated polyene carboxylic acids. The reaction proceeds under oxidant-free conditions, enabling facile access to C6-alkenylated 2-pyridones in high yields with a broad functional group tolerance. Mechanistic studies support the following steps: initial activation of the carboxylic acid in the form of an anhydride, oxidative addition of the activated acid, coordination of the substrate followed by CMD to cleave the C–H bond. Dissociation of CO is followed by decarbonylation of the acyl group to generate the Rh-bound vinyl, and finally reductive elimination and liberation of product closes the cycle. A turnover limiting C–H bond cleavage is likely based on the observed KIE. Further investigation of the mechanism of this reaction and synthetic applications are underway in our laboratories.
Conflicts of interest
There are no conflicts to declare.
Acknowledgements
H. Z. thanks the Program for China Scholarship Council (201806360122), National Natural Science Foundation of China (21372258) and the Beijing National Laboratory for Molecular Sciences (BNLMS201845) for financial support. P. J. W. thanks the US National Science Foundation (CHE-1902509).
Notes and references
-
(a) I. M. Lagoja, Chem. Biodiversity, 2005, 2, 1 CrossRef CAS PubMed;
(b) H. Jessen and J. K. Gademann, Nat. Prod. Rep., 2010, 27, 1168 RSC;
(c) S. Heeb, M. P. Fletcher, S. R. Chhabra, S. P. Diggle, P. Williams and M. Camara, FEMS Microbiol. Rev., 2011, 35, 247 CrossRef CAS PubMed;
(d) F. Christ, A. Voet, A. Marchand, S. Nicolet, B. A. Desimmie, D. Marchand, D. Bardiot, N. J. V. der Veken, B. V. Remoortel, S. V. Strelkov, M. D. Maeyer, P. Chaltin and Z. Debyser, Nat. Chem. Biol., 2010, 6, 442 CrossRef CAS PubMed;
(e) P. Schroder, T. Forster, S. Kleine, C. Becker, A. Richters, S. Ziegler, D. Rauh, K. Kumar and H. Waldmann, Angew. Chem., Int. Ed., 2015, 54, 12398 CrossRef PubMed;
(f) P. L. Wong and K. D. Moeller, J. Am. Chem. Soc., 1993, 115, 11434 CrossRef CAS;
(g) T. R. Kelly, S. H. Bell, N. Ohashi and R. J. Armstrong-Chong, J. Am. Chem. Soc., 1988, 110, 6471 CrossRef CAS;
(h) D. Monti, L. Saccomani, P. Chetoni, S. Burgalassi, S. Tampucci and F. Mailland, Br. J. Dermatol., 2011, 165, 99 CrossRef CAS PubMed;
(i) K. T. Santhosh, O. Elkhateeb, N. Nolette, O. Outbih, A. J. Halayko and S. Dakshinamurti, Br. J. Pharmacol., 2011, 163, 1223 CrossRef CAS PubMed;
(j)
W. Lwowski and A. R. Katritzky, in Comprehensive heterocyclic chemistry: the structure, reactions, synthesis and uses of heterocyclic compounds, ed. A. R. Katritzky and C. W. Rees, Pergamon Press, Oxford, 1984, vol. 8 Search PubMed.
-
(a) M. Torres, S. Gil and M. Parra, Curr. Org. Chem., 2005, 9, 1757 CrossRef CAS;
(b) K. Hirano and M. Miura, Chem. Sci., 2018, 9, 22–32 RSC;
(c) A. M. Prendergast and G. P. McGlacken, Eur. J. Org. Chem., 2018, 2018, 6068–6082 CrossRef CAS;
(d)
A. Brossi, Mammalian alkaloids II, in The alkaloids: chemistry and pharmacology, Elsevier, New York, NY, 1993; vol. 43, pp. 119–183 Search PubMed;
(e) In Alkaloids: Chemical and Biological Perspectives, ed. S. W. Pelletier, Wiley, New York, 1983, pp. 1–31 Search PubMed.
- For selected examples, see:
(a) I. Hachiya, K. Ogura and M. Shimizu, Org. Lett., 2002, 4, 2755 CrossRef CAS PubMed;
(b) Y. Yamamoto, K. Kinpara, T. Saigoku, H. Takagishi, S. Okuda, H. Nishiyama and K. Itoh, J. Am. Chem. Soc., 2005, 127, 605 CrossRef CAS PubMed;
(c) H. Imase, K. Noguchi, M. Hirano and K. Tanaka, Org. Lett., 2008, 10, 3563 CrossRef CAS PubMed;
(d) T. K. Hyster and T. Rovis, Chem. Sci., 2011, 2, 1606 RSC;
(e) Y. Su, M. Zhao, K. Han, G. Song and X. Li, Org. Lett., 2010, 12, 5462 CrossRef CAS PubMed;
(f) L. Ackermann, A. V. Lygin and N. Hofmann, Org. Lett., 2011, 13, 3278 CrossRef CAS PubMed;
(g) M. Fujii, T. Nishimura, T. Koshiba, S. Yokoshima and T. Fukuyama, Org. Lett., 2013, 15, 232 CrossRef CAS PubMed;
(h) M. S. Akhtar, J. J. Shim, S. H. Kim and Y. R. Lee, New J. Chem., 2017, 41, 13027 RSC;
(i) G. Hu, J. Xu and P. Li, Org. Biomol. Chem., 2018, 16, 4151–4158 RSC;
(j) D. Bai, X. Wang, G. Zheng and X. Li, Angew. Chem., Int. Ed., 2018, 57, 6633–6637 CrossRef CAS PubMed;
(k)
A. D. Elbein and R. J. Molyneux, in Alkaloids: Chemical and Biological Perspectives, ed. S. W. Pelletier, Wiley, New York, 1981, vol. 5, pp. 1–54 Search PubMed.
-
(a) L. A. Hasvold, W. Wang, S. L. Gwaltney, T. W. Rockway, L. T. G. Nelson, R. A. Mantei, S. A. Fakhoury, G. M. Sullivan, Q. Li, N.-H. Lin, L. Wang, H. Zhang, J. Cohen, W.-Z. Gu, K. Marsh, J. Bauch, S. Rosenberg and H. L. Sham, Bioorg. Med. Chem. Lett., 2003, 13, 4001 CrossRef CAS PubMed;
(b) D. Conreaux, E. Bossharth, N. Monteiro, P. Desbordes, J.-P. Vors and G. Balme, Org. Lett., 2007, 9, 271 CrossRef CAS PubMed;
(c) M. D. Hill and M. Movassaghi, Chem.–Eur. J., 2008, 14, 6836 CrossRef CAS PubMed;
(d) C. Bengtsson and F. Almqvist, J. Org. Chem., 2010, 75, 972 CrossRef CAS PubMed;
(e) D. Conreaux, S. Belot, P. Desbordes, N. Monteiro and G. Balme, J. Org. Chem., 2008, 73, 8619 CrossRef CAS PubMed;
(f) C. Bengtsson and F. Almqvist, J. Org. Chem., 2010, 75, 972 CrossRef CAS PubMed;
(g) S.-S. P. Chou, Y.-C. Chung, P.-A. Chen, S.-L. Chiang and C.-J. Wu, J. Org. Chem., 2011, 76, 692 CrossRef CAS PubMed;
(h) C. J. McElhinny Jr, F. I. Carroll and A. H. Lewin, Synthesis, 2012, 44, 57 CrossRef;
(i) D. S. Ziegler, R. Greiner, H. Lumpe, L. Kqiku, K. Karaghiosoff and P. Knochel, Org. Lett., 2017, 19, 5760 CrossRef CAS PubMed.
- For C3-selective C–H functionalization, see:
(a) A. Nakatani, K. Hirano, T. Satoh and M. Miura, Chem.–Eur. J., 2013, 19, 7691 CrossRef CAS PubMed;
(b) A. Nakatani, K. Hirano, T. Satoh and M. Miura, J. Org. Chem., 2014, 79, 1377 CrossRef CAS PubMed;
(c) E. E. Anagnostaki, A. D. Fotiadou, V. Demertzidou and A. L. Zografos, Chem. Commun., 2014, 50, 6879 RSC;
(d) A. Modak, S. Rana and D. Maiti, J. Org. Chem., 2015, 80, 296 CrossRef CAS PubMed;
(e) H. U. Lah, F. Rasoola and S. K. Yousuf, RSC Adv., 2015, 5, 78958–78961 RSC;
(f) A. Najib, S. Tabuchi, K. Hirano and M. Miura, Heterocycles, 2016, 92, 1187 CrossRef CAS;
(g) P. Chauhan, M. Ravi, S. Singh, P. Prajapati and P. P. Yadav, RSC Adv., 2016, 6, 109 RSC;
(h) T. Katsina, E. E. Anagnostaki, F. Mitsa, V. Sarlia and A. L. Zografos, RSC Adv., 2016, 6, 6978–6982 RSC.
- For C5-selective C–H functionalization, see:
(a) T. Itahara and F. Ouseto, Synthesis, 1984, 1984, 488–489 CrossRef;
(b) Y. Y. Chen, F. Wang, A. Q. Jia and X. W. Li, Chem. Sci., 2012, 3, 3231 RSC;
(c) U. Dutta, A. Deb, D. W. Lupton and D. Maiti, Chem. Commun., 2015, 51, 17744 RSC;
(d) Y. Li, F. Xie and X. Li, J. Org. Chem., 2016, 81, 715 CrossRef CAS PubMed;
(e) S. Maity, D. Das, S. Sarkar and R. Samanta, Org. Lett., 2018, 20, 5167–5171 CrossRef CAS PubMed.
- For C6-selective C–H functionalization, see:
(a) R. Tamura, Y. Yamada, Y. Nakao and T. Hiyama, Angew. Chem., Int. Ed., 2012, 51, 5679–5682 CrossRef CAS PubMed;
(b) R. Odani, K. Hirano, T. Satoh and M. Miura, Angew. Chem., Int. Ed., 2014, 53, 10784–10788 CrossRef CAS PubMed;
(c) P. A. Donets and N. Cramer, Angew. Chem., Int. Ed., 2015, 54, 633–637 CAS;
(d) D. Das, A. Biswas, U. Karmakar, S. Chand and R. Samanta, J. Org. Chem., 2016, 81, 842–848 CrossRef CAS PubMed;
(e) T. Li, Z. Wang, K. Xu, W. Liu, X. Zhang, W. Mao, Y. Guo, X. Ge and F. Pan, Org. Lett., 2016, 18, 1064–1067 CrossRef CAS PubMed;
(f) S. Yu, Y. Li, X. Zhou, H. Wang, L. Kong and X. Li, Org. Lett., 2016, 18, 2812–2815 CrossRef CAS PubMed;
(g) W. Miura, K. Hirano and M. Miura, Org. Lett., 2016, 18, 3742–3745 CrossRef CAS PubMed;
(h) P. Peng, J. Wang, H. Jiang and H. Liu, Org. Lett., 2016, 18, 5376–5379 CrossRef CAS PubMed;
(i) P. Peng, J. Wang, C. Li, W. Zhu, H. Jiang and H. Liu, RSC Adv., 2016, 6, 57441–57445 RSC;
(j) D. Das, P. Poddar, S. Maity and R. Samanta, J. Org. Chem., 2017, 82, 3612–3621 CrossRef CAS PubMed;
(k) J. Li, Y. Yang, Z. Wang, B. Feng and J. You, Org. Lett., 2017, 19, 3083–3086 CrossRef CAS PubMed;
(l) J. Ni, H. Zhao and A. Zhang, Org. Lett., 2017, 19, 3159–3162 CrossRef CAS PubMed;
(m) W. Miura, K. Hirano and M. Miura, Synthesis, 2017, 49, 4745–4752 CrossRef CAS;
(n) W. Miura, K. Hirano and M. Miura, J. Org. Chem., 2017, 82, 5337–5344 CrossRef CAS PubMed;
(o) K. A. Kumar, P. Kannaboina and P. Das, Org. Biomol. Chem., 2017, 15, 5457–5461 RSC;
(p) T. Li, C. Fu, Q. Ma, Z. Sang, Y. Yang, H. Yang, R. Lv and B. Li, J. Org. Chem., 2017, 82, 10263–10270 CrossRef CAS PubMed;
(q) S. Y. Chen, Q. Li and H. Wang, J. Org. Chem., 2017, 82, 11173–11181 CrossRef CAS PubMed;
(r) A. Biswas, D. Giri, D. Das, A. De, S. K. Patra and R. Samanta, J. Org. Chem., 2017, 82, 10989–10996 CrossRef CAS PubMed;
(s) M. Barday, C. Janot, N. R. Halcovitch, J. Muir and C. Assa, Angew. Chem., Int. Ed., 2017, 56, 13117–13121 CrossRef CAS PubMed;
(t) D. Das and R. Samanta, Adv. Synth. Catal., 2018, 360, 379–384 CrossRef CAS;
(u) L. Zhang, X. Zheng, J. Chen, K. Cheng, L. Jin, X. Jiang and C. Yu, Org. Chem. Front., 2018, 5, 2969–2973 RSC;
(v) F. Gao, X. Han, C. Li, L. Liu, Z. Cong and H. Liu, RSC Adv., 2018, 8, 32659–32663 RSC;
(w) M. Barday, C. Janot, N. R. Halcovitch, J. Muir and C. Aissa, Angew. Chem., Int. Ed., 2017, 56, 13117–13121 CrossRef CAS PubMed;
(x) J. Xia, Z. Huang, X. Zhou, X. Yang, F. Wang and X. Li, Org. Lett., 2018, 20, 740–743 CrossRef CAS PubMed;
(y) J. Diesel, A. M. Finogenova and N. Cramer, J. Am. Chem. Soc., 2018, 140, 4489–4493 CrossRef CAS PubMed.
- For C6-selective C–H alkenylation, see:
(a) Y. Nakao, H. Idei, K. S. Kanyiva and T. Hiyama, J. Am. Chem. Soc., 2009, 131, 15996–15997 CrossRef CAS PubMed;
(b) S. Hazra, K. Hirano and M. Miura, Asian J. Org. Chem., 2019, 8, 1097–1101 CrossRef CAS.
- For reviews, see:
(a) L. J. Goossen, N. Rodriguez and K. Goossen, Angew. Chem., Int. Ed., 2008, 47, 3100–3120 CrossRef CAS PubMed;
(b) N. Rodriguez and L. J. Goossen, Chem. Soc. Rev., 2011, 40, 5030–5048 RSC;
(c) W. I. Dzik, P. P. Lange and L. J. Goossen, Chem. Sci., 2012, 3, 2671–2678 RSC;
(d) K. Park and S. Lee, RSC Adv., 2013, 3, 14165–14182 RSC;
(e) A. J. Borah and G. Yan, Org. Biomol. Chem., 2015, 13, 8094–8115 RSC;
(f) Y. Wei, P. Hu, M. Zhang and W. Su, Chem. Rev., 2017, 117, 8864–8907 CrossRef CAS PubMed;
(g) G. J. P. Perry and I. Larrosa, Eur. J. Org. Chem., 2017, 2017, 3517–3527 CrossRef CAS PubMed;
(h) J. Schwarz and B. Konig, Green Chem., 2018, 20, 323–361 RSC;
(i) J. Buchspies and M. Szostak, Catalysts, 2019, 9, 53 CrossRef;
(j) C. Liu, C. L. Ji, X. Hong and M. Szostak, Angew. Chem., 2018, 130, 16963–16968 CrossRef.
- For catalytic decarboxylative C–H alkenyaltion with alkenyl carboxylica cids, see:
(a) H. Yang, P. Sun, Y. Zhu, H. Yan, L. Lu, X. Qu, T. Li and J. Mao, Chem. Commun., 2012, 48, 7847–7849 RSC;
(b) Z. Cui, X. Shang, X. F. Shao and Z. Q. Liu, Chem. Sci., 2012, 3, 2853–2858 RSC;
(c) Y. Yang, J. Yao and Y. Zhang, Org. Lett., 2013, 15, 3206–3209 CrossRef CAS PubMed;
(d) J. M. Neely and T. Rovis, J. Am. Chem. Soc., 2014, 136, 2735–2738 CrossRef CAS PubMed;
(e) K. Rousee, C. Schneider, S. Couve-Bonnaire, X. Pannecoucke, V. Levacher and C. Hoarau, Chem.–Eur. J., 2014, 20, 15000–15004 CrossRef CAS PubMed;
(f) X. Wang, S. Y. Li, Y. M. Pan, H. S. Wang, Z. F. Chen and K. B. Huang, J. Org. Chem., 2015, 80, 2407–2412 CrossRef CAS PubMed;
(g) N. Zhang, D. Yang, W. Wei, L. Yuan, F. Nie, L. Tian and H. Wang, J. Org. Chem., 2015, 80, 3258–3263 CrossRef CAS PubMed;
(h) S. Agasti, U. Sharma, T. Naveen and D. Maiti, Chem. Commun., 2015, 51, 5375–5378 RSC;
(i) B. Gao, Y. Xie, L. Yang and H. Huang, Org. Biomol. Chem., 2016, 14, 2399–2402 RSC;
(j) S. Agasti, A. Dey and D. Maiti, Chem. Commun., 2016, 52, 12191–12194 RSC;
(k) C. Wang, Y. Lei, M. Guo, Q. Shang, H. Liu, Z. Xu and R. Wang, Org. Lett., 2017, 19, 6412–6415 CrossRef CAS PubMed;
(l) J. B. E. Y. Rouchet, M. Hachem, C. Schneider and C. Hoarau, ACS Catal., 2017, 7, 5363–5369 CrossRef CAS;
(m) S. W. Youn, T. Y. Ko and Y. H. Jang, Angew. Chem., Int. Ed., 2017, 56, 6636–6640 CrossRef CAS PubMed;
(n) K. Xu, Z. Tan, H. Zhang, J. Liu, S. Zhang and Z. Wang, Chem. Commun., 2017, 53, 10719–10722 RSC;
(o) Z. Luo, X. Han, Y. Fang, P. Liu, C. Feng, Z. Li and X. Xu, Org. Chem. Front., 2018, 5, 3299–3305 RSC;
(p) J. F. Zhao, X. H. Duan, Y. R. Gu, P. Gao and L. N. Guo, Org. Lett., 2018, 20, 4614–4617 CrossRef CAS PubMed.
- For catalytic decarbonylative C–H alkenylation with alkenyl carboxylic acids, see:
(a) Z. Q. Lei, J. H. Ye, J. Sun and Z. J. Shi, Org. Chem. Front., 2014, 1, 634–638 RSC;
(b) L. Zhang, R. Qiu, X. Xue, Y. Pan, C. Xu, D. Wang, X. Wang, L. Xu and H. Li, Chem. Commun., 2014, 50, 12385–12388 RSC;
(c) S. Kwon, D. Kang and S. Hong, Eur. J. Org. Chem., 2015, 2015, 3671–3678 CrossRef CAS;
(d) R. Qiu, L. Zhang, C. Xu, X. Pan, H. Pang, L. Xu and H. Li, Adv. Synth. Catal., 2015, 357, 1229–1236 CrossRef CAS;
(e) C. Xu, L. Zhang, J. Xu, Y. Pan, F. Li, H. Li and L. Xu, ChemistrySelect, 2016, 4, 653–658 Search PubMed;
(f) J. Xu, C. Chen, H. Zhao, C. Xu, Y. Pan, X. Xu, H. Li, L. Xu and B. Fan, Org. Chem. Front., 2018, 5, 734–740 RSC;
(g) X. Qiu, P. Wang, D. Wang, M. Wang, Y. Yuan and Z. Shi, Angew. Chem., Int. Ed., 2019, 58, 3100–3120 Search PubMed.
-
(a) Z. Jiang, L. Zhang, C. Dong, Z. Cai, W. Tang, H. Li, L. Xu and J. Xiao, Adv. Synth. Catal., 2012, 354, 3225–3230 CrossRef CAS;
(b) X. Xue, J. Xu, L. Zhang, C. Xu, Y. Pan, L. Xu, H. Li and W. Zhang, Adv. Synth. Catal., 2016, 358, 573–583 CrossRef CAS;
(c) H. Zhao, J. Xu, C. Chen, X. Xu, Y. Pan, Z. Zhang, H. Li and L. Xu, Adv. Synth. Catal., 2018, 360, 985–994 CrossRef CAS;
(d) X. Yang, B. S. Kim, M. Li and P. J. Walsh, Org. Lett., 2016, 18, 2371–2374 CrossRef CAS PubMed;
(e) M. Li, O. Gutierrez, S. Berritt, A. Pascual-Escudero, A. Yeşilçimen, X. Yang, J. Adrio, G. Huang, E. Nakamaru-Ogiso, M. C. Kozlowski and P. J. Walsh, Nat. Chem., 2017, 9, 997 CrossRef CAS PubMed;
(f) S. Duan, M. Li, X. Ma, W. Chen, L. Li, H. Zhang, X. Yang and P. J. Walsh, Adv. Synth. Catal., 2018, 360, 4837–4842 CrossRef CAS;
(g) C. Wu, S. Berritt, X. Liang and P. J. Walsh, Org. Lett., 2019, 21, 960–964 CrossRef CAS PubMed.
- For reviews of transition-metal-catalyzed direct alkenylation of C–H bonds with alkenes, see:
(a) J. L. Bras and J. Muzart, Chem. Rev., 2011, 111, 1170–1214 CrossRef PubMed;
(b) S. I. Kozhushkov and L. Ackermann, Chem. Sci., 2013, 4, 886–896 RSC;
(c) Y. Wu, J. Wang, F. Mao and F. K. Kwong, Chem.–Asian J., 2014, 9, 26–47 CrossRef CAS PubMed;
(d) L. Zhou and W. Lu, Chem.–Eur. J., 2014, 20, 634–642 CrossRef CAS PubMed;
(e) C. Liu, J. Yuan, M. Gao, S. Tang, W. Li, R. Shi and A. Lei, Chem. Rev., 2015, 115, 12138–12204 CrossRef CAS PubMed;
(f) S. Bag and D. Maiti, Synthesis, 2016, 48, 804–815 CrossRef CAS;
(g) W. Ma, P. Gandeepan, J. Li and L. Ackermann, Org. Chem. Front., 2017, 4, 1435–1467 RSC;
(h) R. Manikandan and M. Jeganmohan, Chem. Commun., 2017, 53, 8931–8947 RSC.
-
(a) Y. Takahama, Y. Shibata and K. Tanaka, Chem.–Eur. J., 2015, 21, 9053–9056 CrossRef CAS PubMed;
(b) X. F. Yang, X. H. Hu, C. Feng and T. P. Loh, Chem. Commun., 2015, 51, 2532–2535 RSC;
(c) Y. Lu, H. W. Wang, J. E. Spangler, K. Chen, P. P. Cui, Y. Zhao, W. Y. Sun and J. Q. Yu, Chem. Sci., 2015, 6, 1923–1927 RSC;
(d) K. Muralirajan, R. Haridharan, S. Prakash and C. H. Cheng, Adv. Synth. Catal., 2015, 357, 761–766 CrossRef CAS;
(e) Z. Song, R. Samanta and A. P. Antonchick, Org. Lett., 2013, 15, 5662–5665 CrossRef CAS PubMed;
(f) B. Liu, Y. Fan, Y. Gao, C. Sun, C. Xu and J. Zhu, J. Am. Chem. Soc., 2012, 135, 468–473 CrossRef PubMed;
(g) C. Feng and T. P. Loh, Chem. Commun., 2011, 47, 10458–10460 RSC;
(h) C. Wang, H. Chen, Z. Wang, J. Chen and Y. Huang, Angew. Chem., Int. Ed., 2012, 51, 7242–7245 CrossRef CAS PubMed;
(i) X. Wei, F. Wang, G. Song, Z. Du and X. Li, Org. Biomol. Chem., 2012, 10, 5521–5524 RSC;
(j) D. Zhao, S. Vásquez-Céspedes and F. Glorius, Angew. Chem., Int. Ed., 2015, 54, 1657–1661 CrossRef CAS PubMed;
(k) S. Rakshit, C. Grohmann, T. Besset and F. Glorius, J. Am. Chem. Soc., 2011, 133, 2350–2353 CrossRef CAS PubMed;
(l) B. Gong, J. Shi, X. Wang, Y. Yan, Q. Li, Y. Meng, H. E. Xu and W. Yi, Adv. Synth. Catal., 2014, 356, 137–143 CrossRef CAS;
(m) F. W. Patureau, T. Besset and F. Glorius, Angew. Chem., Int. Ed., 2011, 50, 1064–1067 CrossRef CAS PubMed;
(n) H. Wang, B. Beiring, D. G. Yu, K. D. Collins and F. Glorius, Angew. Chem., Int. Ed., 2013, 52, 12430–12434 CrossRef CAS PubMed;
(o) B. Li, J. Ma, W. Xie, H. Song, S. Xu and B. Wang, Chem.–Eur. J., 2013, 19, 11863–11868 CrossRef CAS PubMed;
(p) P. Lu, C. Feng and T. P. Loh, Org. Lett., 2015, 17, 3210–3213 CrossRef CAS PubMed;
(q) J. Wen, A. Wu, M. Wang and J. Zhu, J. Org. Chem., 2015, 80, 10457–10463 CrossRef CAS PubMed;
(r) S. Rej and N. Chatani, ACS Catal., 2018, 8, 6699–6706 CrossRef CAS;
(s) M. D. Boele, G. P. van Strijdonck, A. H. De Vries, P. C. Kamer, J. G. de Vries and P. W. van Leeuwen, J. Am. Chem. Soc., 2002, 124, 1586–1587 CrossRef CAS PubMed;
(t) G. Cai, Y. Fu, Y. Li, X. Wan and Z. Shi, J. Am. Chem. Soc., 2007, 129, 7666–7673 CrossRef CAS PubMed;
(u) A. García-Rubia, R. G. Arrayás and J. C. Carretero, Angew. Chem., Int. Ed., 2009, 48, 6511–6515 CrossRef PubMed;
(v) L. Ackermann, L. Wang, R. Wolfram and A. V. Lygin, Org. Lett., 2012, 14, 728–731 CrossRef CAS PubMed;
(w) B. Li, J. Ma, N. Wang, H. Feng, S. Xu and B. Wang, Org. Lett., 2012, 14, 736–739 CrossRef CAS PubMed;
(x) R. Manikandan, P. Madasamy and M. Jeganmohan, ACS Catal., 2015, 6, 230–234 CrossRef.
-
(a) E. Vitaku, D. T. Smith and J. T. Njardarson, J. Med. Chem., 2014, 57, 10257–10274 CrossRef CAS PubMed;
(b) R. D. Taylor, M. MacCoss and A. D. Lawson, J. Med. Chem., 2014, 57, 5845–5859 CrossRef CAS PubMed.
-
(a) L. J. Goossen, J. Paetzold and L. Winkel, Synlett, 2002, 2002, 1721–1723 CrossRef;
(b) L. J. Goossen and A. Döhring, Synlett, 2004, 2004, 263–266 CrossRef;
(c) C. Zhao, X. Jia, X. Wang and H. Gong, J. Am. Chem. Soc., 2014, 136, 17645–17651 CrossRef CAS PubMed.
-
(a) R. Kakino, H. Narahashi, I. Shimizu and A. Yamamoto, Bull. Chem. Soc. Jpn., 2002, 75, 1333–1345 CrossRef CAS;
(b) L. J. Goossen and K. Ghosh, Eur. J. Org. Chem., 2002, 2002, 3254–3267 CrossRef;
(c) Y. T. Hong, A. Barchuk and M. J. Krische, Angew. Chem., Int. Ed., 2006, 45, 6885–6888 CrossRef CAS PubMed;
(d) X. D. Zhao and Z. Yu, J. Am. Chem. Soc., 2008, 130, 8136–8137 CrossRef CAS PubMed;
(e) W. Jin, Z. Yu, W. He, W. Ye and W. J. Xiao, Org. Lett., 2009, 11, 1317–1320 CrossRef CAS PubMed;
(f) X. Qi, Y. Li, R. Bai and Y. Lan, Acc. Chem. Res., 2017, 50, 2799–2808 CrossRef CAS PubMed.
Footnote |
† Electronic supplementary information (ESI) available. CCDC 1874166. For ESI and crystallographic data in CIF or other electronic format see DOI: 10.1039/c9sc03672e |
|
This journal is © The Royal Society of Chemistry 2019 |