DOI:
10.1039/C9RA07660C
(Paper)
RSC Adv., 2019,
9, 38271-38279
Facile synthesis of perovskite CeMnO3 nanofibers as an anode material for high performance lithium-ion batteries
Received
21st September 2019
, Accepted 14th November 2019
First published on 22nd November 2019
Abstract
A facile synthesis of perovskite-type CeMnO3 nanofibers as a high performance anode material for lithium-ion batteries was demonstrated. The nanofibers were prepared by the electrospinning technique. The characterization of CeMnO3 nanofibers was carried out by X-ray diffraction, Fourier transform infrared spectroscopy, scanning electron microscopy (SEM), transmission electron microscopy (TEM), and X-ray photoelectron spectroscopy. SEM images manifested nanofibers with a diameter of 470 nm having a rough surface with a porous structure. TEM images were consistent with the observations from the SEM images. The electrochemical properties of CeMnO3 perovskite in lithium-ion batteries were investigated. The CeMnO3 anode exhibited a discharge capacity of 2159 mA h g−1 with a coulombic efficiency of 93.79%. In addition, a high cycle stability and a capacity of 276 mA h g−1 at the current density of 1000 mA g−1 can be effectively maintained due to the high Li+ conductivity in the CeMnO3 anode. This study could provide an efficient and potential application of perovskite-type CeMnO3 nanofibers in lithium-ion batteries.
Introduction
With the rapid development of science and technology, electronic equipment has also undergone exponential evolution during the past 5 decades. The electrical power unit (EPU) makes people's life more convenient. In the meantime, it leads to many serious issues, such as the damage and pollution of the environment. It is extremely urgent to develop environmentally friendly and efficient energy storage systems for current electric vehicles.1 The lithium-ion battery (LIB) is one of the most desired electrochemical energy storage devices thanks to its high voltage, high energy density, small self-discharge, long cycle retention, no-memory effect, and eco-friendliness. At present, graphite is the most common anode material for the commercial LIBs with a relatively low theoretical specific capacity of 372 mA h g−1.2 The slow evolution of electrode materials seriously restricts the further development of LIBs commercialization. Therefore, in the cause of meeting market requirements, it is an emergency to develop anode materials having high energy density, high safety, and long cycle life for high performance energy storage system.3–9
So far, many researchers have studied a variety of materials for high performance LIB anodes. Silicon has attracted much attention thanks to the ultra-high theoretical specific capacity (4200 mA h g−1). However, there is a critical issue that the large volume expansion ratio limits its application in LIBs.10–12 It was reported that transition metal oxides exhibited favorable electrochemical performances as anode materials for LIBs, such as CoxOy,13–16 Fe3O4,17,18 NixOy,19,20 and MnxOy.21,22 Specially, MnxOy is regarded as a promising material for LIB anode owing to abundance, low cost, and eco-friendliness.23 But low conductivity gives rise to structural collapse of the anode material during Li+ ion insertion/deinsertion, leading to poor cycle stability.24–27 For purpose of solving this critical issue, researchers focused on improving the conductivity of anode material. Graphene-wrapped MnO2 nanoribbons synthesized by hydrothermal reaction showed a specific capacity of 890 mA h g−1 at a current density of 100 mA g−1.28 The chemically fabricated coaxial MnO2/CNT nanocomposites presented a specific capacity of 474 mA h g−1 at a current density of 1600 mA g−1.29 In addition, MnO2@TiO2 nanocomposites with core@shell structure, which greatly improved the anode stability, presented a specific capacity of 938 mA h g−1 at a current density of 300 mA g−1.30 As a stable oxide, CeO2 is used in the fields of electronic devices, catalysis, and electrochemical energy storage due to its favorably thermal stability, catalytic activity, and suitable valence states.31–33 The charge mass transfer resistance is greatly reduced, and the electrochemical performance is highly improved by constructing ternary perovskite structure.34 Till now, the application of CeMnO3 nanofibers with perovskite structure in lithium-ion batteries has not been studied.
In this work, a facile synthesis of perovskite-type CeMnO3 nanofibers by electrospinning technique as high performance anode material for lithium-ion batteries was demonstrated. This technique is easy to operate and applicable to synthesize many kinds of nanomaterials. Those nanofibers showed rough surface with mesoporous structure. The surface area and pore volume of CeMnO3 are 108.772 m2 g−1 and 0.176 cm3 g−1, respectively. The high surface area and mesoporous structure facilitate the penetration of electrolyte and the diffusion of Li+ to the active electrode material. CeMnO3 anode exhibited a discharge capacity of 2159 mA h g−1 with the coulombic efficiency of 93.79%. And a high reversible capacity of 395 mA h g−1 at 200 mA g−1 for CeMnO3 can be obtained even at a high discharge rate of 1000 mA g−1 after 60 cycles. The perovskite CeMnO3 with nanofiber-like structure exhibits favorable electrochemical behaviors. The layered structure from Mn–O octahedra in perovskite facilitates the insertion and extraction of Li+. In addition, CeMnO3 with perovskite structure improves the diffusion of Li+, alleviates the structure collapse resulting from Li+ insertion and extraction, and enhances the cycle stability. It indicates the potential application of perovskite-type CeMnO3 nanofibers for lithium-ion batteries.
Experimental
The CeMnO3 nanofibers were fabricated by effective electrospinning technique at room temperature and subsequent calcination process at 500 °C. Cerium nitrate hexahydrate (Ce(NO3)3·6H2O, 1.086 g) and manganese acetate (MnAc, 0.4326 g) were served as the sources of Ce and Mn. Polyvinylpyrrolidone (PVP, Mw ≈ 1
300
000, 2.25 g) was worked as nanofiber template. N,N-dimethylformamide (DMF, 16.5 mL) was used as the solvent. The precursor solution was prepared by mixing Ce(NO3)3·6H2O, MnAc, and PVP into DMF, and stirring for 14 h. Then, this brownish solution was loaded into a 10 mL plastic syringe and electrospun at 18 kV with the distance between a collector and a needle of 15 cm at the flow rate of 0.75 mL h−1. The as-spun Ce(NO3)3–MnAc/PVP nanofibers were dried at 55 °C overnight in a vacuum oven. The calcination process was performed at 500 °C for 2 h with a heating rate of 1 °C min−1 in a muffle furnace under air atmosphere. In addition, control samples of CeO2 and Mn3O4 nanofibers was fabricated under the same condition without Mn and Ce source, respectively.
Brunauer–Emmett–Teller and Barrett–Joyner–Halenda (BET and BJH, Quadrasorbsi) methods were performed to determine the specific surface area and pore size distribution of CeMnO3 NFs. The crystal structures of CeMnO3, CeO2, and Mn3O4 were studied using X-ray diffractometer (XRD, Bruker D8 Focus) at room temperature. The Fourier transforms infrared (FT-IR, Bruker Vector 22 Spectrometer) spectra of as-spun Ce(NO3)3–MnAc/PVP NFs and CeMnO3 NFs were detected in the range from 4000 to 400 cm−1 using KBr as dispersant. The microstructures and morphologies of CeMnO3 NFs were analyzed on a scanning electron microscope (SEM, Hitachi S-4800, 20 kV) and a transmission electron microscope (TEM, JEOL JEM 2100, 200 kV). X-ray photoelectron spectroscopy (XPS, Al Kα, Thermo Escalab 250) was carried out to analyze the valence states of CeMnO3 NFs.
The electrochemical characteristics of CeO2, Mn3O4, and CeMnO3 were performed by assembling 2032-type coin cells. Electrodes were fabricated by adding CeMnO3 (80 wt%), acetylene black (10 wt%), and polytetrafluoroethylene (PTFE, 10 wt%) into N-methyl-2-pyrrolidone (NMP) to form a homogeneous slurry. Then, the slurry was roll-pressed on aluminum foil and dried at 60 °C overnight in a vacuum oven. Electrochemical cells were fabricated with an active material as an anode, a lithium foil as a cathode, a Celgard 2400 microporous membrane as a separator, and 1 mol L−1 LiPF6 as electrolyte solution. Those cells were assembled in an Ar-filled glove box with both oxygen and moisture content below 1 ppm (Vigor, Co. Ltd., Suzhou, China). Galvanostatic charge/discharge behaviors of CeO2, Mn3O4, and CeMnO3 were detected using a battery testing system (Land Co. Ltd., Wuhan, China) with a voltage window of 0.01–3.0 V vs. Li/Li+ for setting current rates. Electrochemical impedance spectroscopy (EIS) measurements were implemented via an electrochemical workstation (Bio-Logic, Co. Ltd, Seyssinet-pariset, France). The AC perturbation signal was 5 mV with the frequency ranging from 0.01 to 105 Hz. Impedance data were analyzed using an electrochemical impedance software of EC-Lab.
Results and discussion
According to BET and BJH methods, the surface area and pore volume of CeMnO3 are 108.772 m2 g−1 and 0.176 cm3 g−1, respectively. In addition, the average pore diameter is 4.89 nm, indicating the mesoporous structure. The high surface area is of great importance to facilitate the diffusion of Li+ from electrolyte to electrode material. The schematic illustration of CeMnO3 with perovskite structure is depicted as shown in Fig. 1. The crystal structures of CeMnO3, CeO2, and Mn3O4 were recorded by XRD pattern which are displayed in Fig. 2(a). For CeO2 sample, the diffraction peaks are assigned to (111), (200), (220), (311), (222), (400), (331) and (420) planes of cubic fluorite CeO2. The XRD pattern matches well with the JCPDS card (34-0394) implying a face-centered cubic fluorite structure of CeO2 with space group Fm
m.35 The diffraction peaks of Mn3O4 are related to (112), (200), (103), (211), (004), (220), (105), (303), (321), (224), and (400), which are agree with the JCPDS card (24-0734).36 For CeMnO3 NFs, the diffraction peaks slightly transfer to higher Bragger angle and the intensities become weaker and broader. The four major diffraction peaks of CeMnO3 correspond to the (111) plane of CeO2 and the (022), (02-2), and (220) planes of CeMnO3.37–39 The FT-IR spectra of as-spun Ce(NO3)3–MnAc/PVP NFs and CeMnO3 NFs are depicted in Fig. 2(b). The peaks at 3434 and 1625 cm−1 correspond to the stretching and vibration mode of O–H. The peak at 2919 cm−1 is assigned to the stretching C–H vibration of alkyl groups. The peaks at 1651, 1438, and 1259 cm−1 are ascribed to C
O, C–H, and C–N functional groups.40–42 Those peaks from Ce(NO3)3–MnAc/PVP NFs after calcination were weakened or vanished. The peaks at 520 and 433 cm−1 refer to the metal–oxygen stretching vibrations of Ce–O and Mn–O.40,43
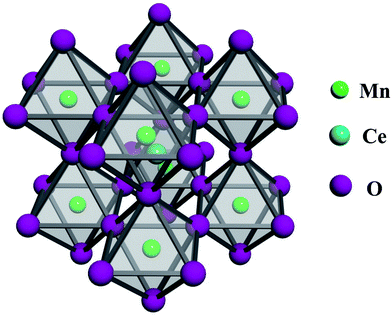 |
| Fig. 1 The schematic structure of perovskite-type CeMnO3. | |
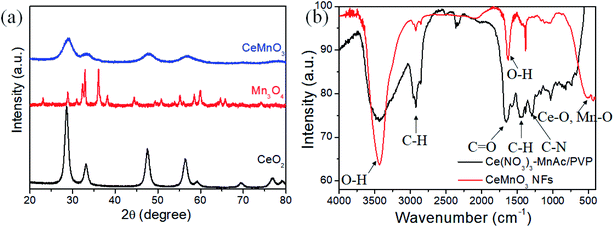 |
| Fig. 2 (a) XRD patterns of CeO2, Mn3O4, and CeMnO3 NFs. (b) FT-IR spectra of as-spun Ce(NO3)3–MnAc/PVP NFs and CeMnO3 NFs. | |
The SEM images of Ce(NO3)3–MnAc/PVP NFs and CeMnO3 NFs are shown in Fig. 3. The morphology of the precursor NFs was beige and uniform with the diameter of 550 nm. After calcination, the surfaces of CeMnO3 NFs were no longer smooth, and those nanofibers were broken up into sections which could be originated from the combustion of PVP, the decomposition of nitrate and acetate, and the crystallization of Ce–Mn–O.35,44 The diameter was reduced to 470 nm. The nanofiber-like structure provides a short diffusion path for ion transport.45 The detailed morphology and structure of an individual CeMnO3 NF were further investigated by TEM micrographs (Fig. 4). The TEM images indicate that the diameter of the individual nanofiber is 470 nm. The nanofiber shows rough surface with porous structure, which is greatly agree with the observations from SEM images.
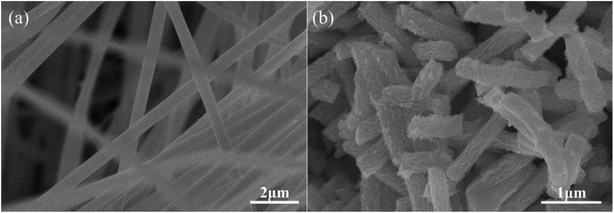 |
| Fig. 3 (a) SEM image of as-spun Ce(NO3)3–MnAc/PVP NFs. (b) SEM image of CeMnO3 NFs. | |
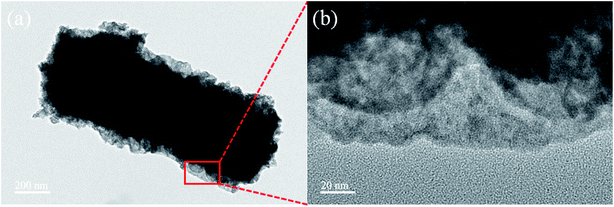 |
| Fig. 4 (a) TEM image and (b) high resolution TEM image of CeMnO3 NF. | |
The chemical bonding states and compositions of the fabricated CeMnO3 NFs have been detected as shown in Fig. 5. In the survey spectrum, the peaks of O 1s, Mn 2P, Ce 3d, and Ce 4d have been shown in Fig. 5(a). Ce 3d spectrum was analyzed as shown in Fig. 5(b). Six major peaks can be observed, including three peaks at 882.32, 888.73, and 898.1 eV corresponding to the component of Ce 3d5/2, and three peaks at 900.71, 907.33, and 916.49 eV being assigned to the component of Ce 3d5/2.46 It confirms the existence of Ce4+ in the CeMnO3 NFs.47 The detailed information of Ce 3d spectra is shown in Table 1. The binding energy peak at 108.6 eV with the component of Ce 4d5/2 from final state of 4d94f1 + 4d94f2 can be clearly seen in Ce 4d spectrum (Fig. 5(c)), indicating the presence of Ce3+.49 Mn 2p spectrum is exhibited in Fig. 5(d). Two major peaks at 642.18 and 653.99 eV are assigned to the component of Mn 2p3/2 and Mn 2p1/2, respectively, implying the existence of Mn3+.50 As shown in Fig. 5(e), the main O 1s peaks at 529.73 and 530.90 eV are related to the oxygen atoms in the lattice and the oxygen ions of the oxygen deficient region in CeMnO3 NFs, respectively.51
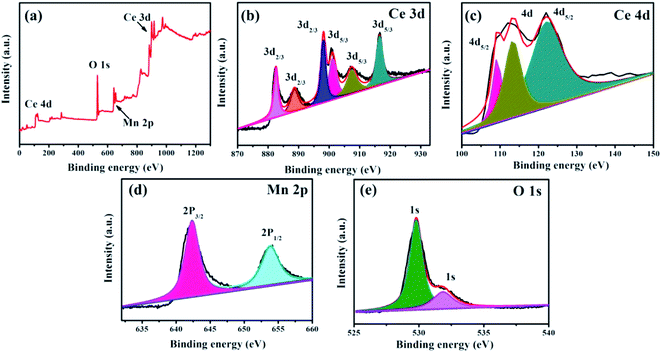 |
| Fig. 5 XPS spectra CeMnO3 NFs: (a) survey scan, (b) Ce 3d region, (c) Ce 4d region, (d) Mn 2p region, and (e) O 1s region. | |
Table 1 Information of the Ce 3d spectrum of XPS46–48
Ce contribution |
Peak position (eV) |
Peak characteristics |
Final state |
Ce(IV) |
882.32 |
3d5/2 |
3d94f2 |
Ce(IV) |
888.73 |
3d5/2 |
3d94f1 |
Ce(IV) |
898.1 |
3d5/2 |
3d94f0 |
Ce(IV) |
900.71 |
3d3/2 |
3d94f2 |
Ce(IV) |
907.33 |
3d3/2 |
3d94f1 |
Ce(IV) |
916.49 |
3d3/2 |
3d94f0 |
To further clarify the electrochemical performances of CeO2, Mn3O4, and CeMnO3 anodes, CV curves of electrodes for the 1st, 2nd and 5th cycles were analyzed. In Fig. 6(a), two reduction peaks V1 and V2 located at 0.2 and 0.6 V are detected in the first scan, which originated from the formation of solid electrolyte interphase (SEI) and the initial reduction of CeO2 to Ce (CeO2 + 4Li+ + 4e− = Ce + 2Li2O), respectively. The oxidation peak at about 2.5 V (V3) as a result of the reaction between Ce and Li2O (Ce + 2Li2O = CeO2 + 4Li+ + 4e−).52 In Fig. 6(b), the major cathodic peaks in the 1st cycle for Mn3O4 located at 0.2 and 1.35 V. And the peak at 0.2 V is related to the formation of SEI layer and the reduction of MnxOy with Li ions, which can be depicted by Mn3O4 + 8Li+ + 8e− = 3Mn + 4Li2O.53 After five repetitive cycles, the oxidation-reduction peaks decreased slightly, and a good stability can be carefully maintained. As show in Fig. 6(c), compared with CeO2 and Mn3O4, CeMnO3 shows relatively different peak positions. The oxidation peaks at 1.2 and 2.4 V are attributed to the oxidation of Ce3+ and Mn3+, and there is a wide plateau at 0.5–1.0 V, which originates from the reduction of Mn3+ to metallic Mn.54 A small CV curve was shown in the first cycle, which might be due to the super ion-conduction of perovskite CeMnO3.
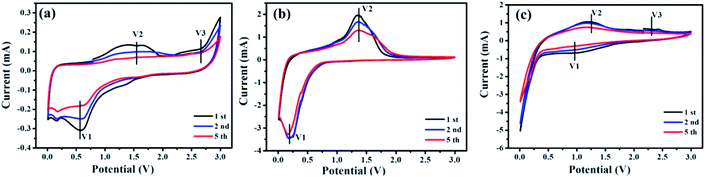 |
| Fig. 6 CV curves of electrodes for 1st, 2nd and 5th cycles at a scan rate of 0.05 mV s−1 of (a) CeO2, (b) Mn3O4, and (c) CeMnO3. | |
Fig. 7 displays the initial discharge profiles of CeO2, Mn3O4, and CeMnO3 anodes. The cells were charged and discharged at a current density of 200 mA g−1 in the voltage range of 0.01–3.0 V versus Li+/Li. The three fabricated samples exhibit discharge capacities of 338, 592, 2159 mA h g−1 with coulombic efficiencies of 94.67%, 84.63%, and 93.79%, respectively. The SEI layer formed between electrode and electrolyte leads to the irreversible capacity reduction in the first cycle.55 From the discharge curve, CeO2 and Mn3O4 cells exhibit considerably narrow discharge platforms. However, it is obvious that perovskite CeMnO3 has a relatively stable discharge platform at 0.5 V. As shown in Fig. 1, the layered structure from Mn–O octahedra is constructed by sharing one oxygen atom. Between the layers, the insertion and deinsertion of Li+ can achieve from this perovskite structure.33 Therefore, CeMnO3 NFs exhibit high reversible capacity compared with the other two samples.
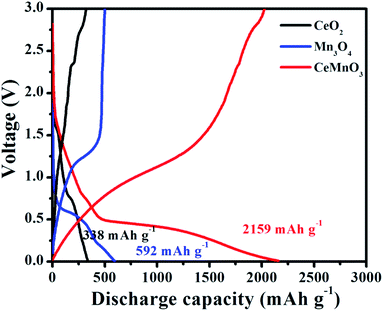 |
| Fig. 7 The initial charge–discharge profiles of CeO2, Mn3O4, and CeMnO3 in the voltage of 0.01–3.0 V. | |
The cycling performances of CeO2, Mn3O4, and CeMnO3 anodes under a current density of 200 mA g−1 with the voltage ranging from 0.01 to 3.0 V are presented in Fig. 8. Within 20 cycles, the capacities of the three samples rapidly declined. The discharge specific capacity of CeO2 reduces to lower than 200 mA h g−1 after ten cycles and that of Mn3O4 after 20 cycles is about 300 mA h g−1. Due to the Jahn–Teller effect, Mn3+ is gradually converted into Mn2+ and Mn4+, and then dispersed in the electrolyte, resulting in irreversible capacity reduction after consecutive cycling. A stable capacity can be achieved in CeMnO3 anode. For CeMnO3 anode, after 60 cycles the capacity gradually increases, because the anode undergoes a rapid activation process.56 The reaction of CeMnO3 + Li2O = CeO2 + MnO2 + 2Li+ + 2e− is gradually strengthened, which further improves the electrochemical performance. Compared with Mn3O4, the unique perovskite structure of CeMnO3 can improve the diffusion of Li+, alleviate the structure collapse resulting from Li+ insertion and extraction, and improve the cycle stability.33 After 200 cycles the capacities of CeO2, Mn3O4, and CeMnO3 anode are 175.6, 315 and 322.9 mA h g−1, respectively. In comparison with common CeO2 with the reversible capacity of 315 mA h g−1 after 50 cycles, the fabricated CeMnO3 as anode material exhibits more stable cycle life and higher capacity.57
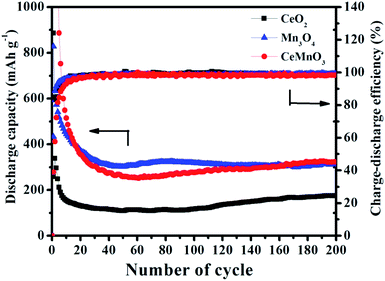 |
| Fig. 8 The cycle capabilities of CeO2, Mn3O4, and CeMnO3 at 200 mA g−1 in the voltage range of 0.01–3.0 V. | |
Fig. 9 exhibits the rate capabilities of CeO2, Mn3O4 and CeMnO3. The three kinds of assembled cells were measured at the current density of 200 mA g−1 in the first 10 cycles, and then measured at various current densities ranging from 400 to 1000 mA g−1. Among the three samples, CeMnO3 displays the best performance and the highest reversible capacity of 530 mA h g−1 at the current density of 200 mA g−1 after 10 cycles. When the current densities increased, the capacity slightly decreased. Particularly, after discharging at 1000 mA g−1, CeMnO3 anode still presents a reversible discharge capacity of 276 mA h g−1. Furthermore, an ultra-high reversible capacity of 395 mA h g−1 at 200 mA g−1 can be obtained even at a high discharge rate of 1000 mA g−1 after 60 cycles. As illustrated in Fig. 10, the sloping line in the low frequency region is ascribed to Warburg impedance (Zw).58 The Rct value of CeMnO3 is 36.78 Ω, which is much lower than those of CeO2 (49.18 Ω) and Mn3O4 (67.99 Ω), implying the high conductivity of the electrochemical system consisting of CeMnO3. In order to further clarify the Li+ diffusion performance, the Warburg impedance (Zw) for the equivalent circuit (as illustrated in the inset of Fig. 10), the exchange current density (i°) and the diffusion coefficient of Li+ (D) can be depicted by the following equation.59
|
|Z| = Re + Rct + σω−1/2
| (1) |
|
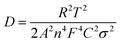 | (2) |
|
 | (3) |
where,
ω is the angular frequency,
R is ideal gas constant,
n is number of transfer charge,
F is the Faraday constant, and
C is the concentration of Li
+.
σ is the Warburg coefficient which can be calculated from the slope of the Bode plot (as show in
Fig. 11). CeMnO
3 exhibits the highest Li
+ diffusion coefficient of 6.7261 × 10
−16 cm
2 s
−1. It confirms that CeMnO
3 anode exhibits a favorable Li
+ diffusion performance. The detailed electrochemical impedance parameters of CeO
2, Mn
3O
4, and CeMnO
3 anodes were summarized in
Table 2.
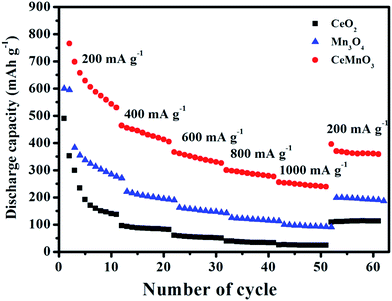 |
| Fig. 9 Rate performance of as-prepared CeO2, Mn3O4, and CeMnO3. | |
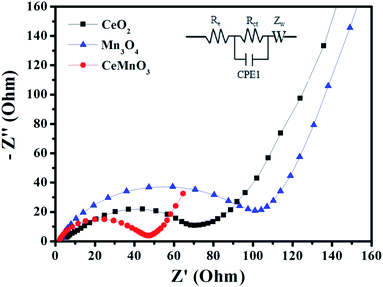 |
| Fig. 10 Impedance spectra of CeO2, Mn3O4, and CeMnO3 at open circuit voltage. Inset: equivalent circuit corresponding to the impedance diagrams. | |
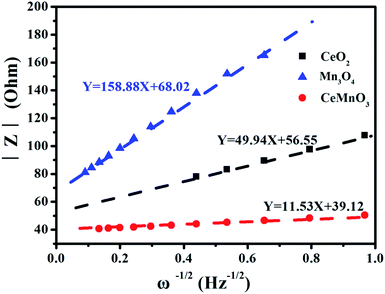 |
| Fig. 11 Fitting line of the |Z| vs. ω−1/2 relationship of CeO2, Mn3O4, and CeMnO3. | |
Table 2 Electrochemical impedance parameters of CeO2, Mn3O4, and CeMnO3
Sample |
Re (Ω) |
Rct (Ω) |
σ (Ω s−0.5) |
D (cm2 s−1) |
i° (mA cm2) |
CeO2 |
7.669 |
49.18 |
49.94 |
3.5876 × 10−17 |
5.2134 × 10−4 |
Mn3O4 |
2.99 |
67.99 |
158.88 |
3.5423 × 10−18 |
3.8293 × 10−4 |
CeMnO3 |
2.596 |
36.78 |
11.53 |
6.7261 × 10−16 |
7.0787 × 10−3 |
The perovskite CeMnO3 with nanofiber-like structure present favorable electrochemical behaviors. The perovskite structure facilitates the insertion of Li+ into Ce-site vacancies and stabilize the cycling performance after the initial discharge process.60 The nanofiber-like structure can effectively improve the contact area between electrolyte and anode and shorten the path of Li+ entering anode material, thus reducing the electrochemical impedance of the material.61
Conclusions
Perovskite-type CeMnO3 nanofibers as high performance anode material for lithium-ion batteries was effectively synthesized by electrospinning process. These nanofibers with a diameter of 470 nm present rough surface with mesoporous structure. The electrochemical properties of CeMnO3 perovskite in lithium-ion batteries were investigated. CeMnO3 anode exhibited a discharge capacity of 2159 mA h g−1 with a coulombic efficiency of 93.79%. A high reversible capacity of 395 mA h g−1 at 200 mA g−1 for CeMnO3 can be obtained even at a high discharge rate of 1000 mA g−1 after 60 cycles. This study provides the feasibility of perovskite-type CeMnO3 nanofibers for the application into lithium-ion batteries. This result opens a new path for a more efficient and convenient chemical storage method in the near future.
Conflicts of interest
There are no conflicts to declare.
Acknowledgements
This work was financially supported by the Doctoral Scientific Research Foundation of Inner Mongolia University for Nationalities (Project No: BS456), and the Scientific Research Program of Inner Mongolia University for Nationalities (Project No: NMDYB19045). The National Natural Science Foundation of China (21961024, 21961025), Inner Mongolia Natural Science Foundation (2018JQ05). Supported by Incentive Funding from Nano Innovation Institute (NII) of Inner Mongolia University for Nationalities (IMUN). Inner Mongolia Autonomous Region Funding Project for Science & Technology Achievement Transformation (CGZH2018156). Inner Mongolia Autonomous Region Incentive Funding Guided Project for Science & Technology Innovation (2016). Tongliao Funding Project for Application Technology Research & Development (2017).
References
- A. Fotouhi, D. J. Auger, K. Propp, S. Longo and M. Wild, A review on electric vehicle battery modelling: from lithium-ion toward lithium–sulphur, Renewable Sustainable Energy Rev., 2016, 56, 1008–1021 CrossRef CAS
. - J. Ma, F. Yu, Z. Wen, M. Yang, H. Zhou, C. Li, L. Jin, L. Zhou, L. Chen, Z. Yuan and J. Chen, A facile one-pot method for synthesis of low-cost iron oxide/activated carbon nanotube electrode materials for lithium-ion batteries, Dalton Trans., 2013, 42, 1356–1359 RSC
. - Y. Zhao, X. Li, B. Yan, D. J. Li, S. Lawes and X. L. Sun, Significant impact of 2D graphene nanosheets on large volume change tin-based anodes in lithium-ion batteries: a review, J. Power Sources, 2015, 274, 869–884 CrossRef CAS
. - Y. Deng, C. Fang and G. Chen, The developments of SnO2/graphene nanocomposites as anode materials for high performance lithium ion batteries: a review, J. Power Sources, 2016, 304, 81–101 CrossRef CAS
. - N. Nitta, F. Wu, J. T. Lee and G. Yushin, Li-ion battery materials: present and future, Mater. Today, 2015, 18, 252–264 CrossRef CAS
. - W. Shi, J. Mao, X. Xu, W. Liu, L. Zhang, X. Cao and X. Lu, An ultra-dense NiS2/reduced graphene oxide composite cathode for high-volumetric/gravimetric energy density nickel–zinc batteries, J. Mater. Chem. A, 2019, 7, 15654–15661 RSC
. - L. Zhang, W. Liu, W. Shi, X. Xu, J. Mao, P. Li, C. Ye, R. Yin, S. Ye, X. Liu, X. Cao and C. Gao, Boosting lithium storage properties of MOF derivatives through a wet-spinning assembled fiber strategy, Chem.–Eur. J., 2018, 24(52), 13792–13799 CrossRef CAS PubMed
. - W. Hong, P. Ge, Y. Jiang, L. Yang, Y. Tian, G. Zou, X. Cao, H. Hou and X. Ji, Yolk-shell structured bismuth@N-doped carbon anode for lithium-ion battery with high volumetric capacity, ACS Appl. Mater. Interfaces, 2019, 11(11), 10829–10840 CrossRef CAS PubMed
. - W. Liu, R. Yin, X. Xu, L. Zhang, W. Shi and X. Cao, Structural engineering of low-dimensional metal–organic frameworks: synthesis, properties, and applications, Adv. Sci., 2019, 1802373 CrossRef PubMed
. - Y. Jin, B. Zhu, Z. Lu, N. Liu and J. Zhu, Challenges and recent progress in the development of Si anodes for lithium-ion battery, Adv. Energy Mater., 2017, 7, 1700715 CrossRef
. - Q. Xu, J. Y. Li, Y. X. Yin, Y. M. Kong, Y. G. Guo and L. J. Wang, Nano/micro-structured Si/C anodes with high initial coulombic efficiency in Li-ion batteries, Chem.–Asian J., 2016, 11, 1205–1209 CrossRef CAS PubMed
. - D. A. Agyeman, K. Song, G. H. Lee, M. Park and Y. M. Kang, Carbon-coated Si nanoparticles anchored between reduced graphene oxides as an extremely reversible anode material for high energy-density Li-ion battery, Adv. Energy Mater., 2016, 6, 1600904 CrossRef
. - S. H. Cho, J. W. Jung, C. Kim and I. D. Kim, Rational design of 1-D Co3O4 nanofibers@low content graphene composite anode for high performance Li-ion batteries, Sci. Rep., 2017, 7, 45105 CrossRef CAS PubMed
. - H. Zhou, L. Zhang, D. Zhang, S. Chen, P. R. Coxon, X. He, M. Coto, H. K. Kim, K. Xi and S. Ding, A universal synthetic route to carbon nanotube/transition metal oxide nano-composites for lithium ion batteries and electrochemical capacitors, Sci. Rep., 2016, 6, 37752 CrossRef CAS PubMed
. - Y. Li, X. Hou, Y. Li, Q. Ru, S. Hu and K. Lam, The design and synthesis of polyhedral Ti-doped Co3O4 with enhanced lithium-storage properties for Li-ion batteries, J. Mater. Sci.: Mater. Electron., 2016, 27, 11439–11446 CrossRef CAS
. - L. Liu, L. Mou, J. Yu and S. Chen, Urchin-like CoO–C micro/nano hierarchical structures as high performance anode materials for Li-ion batteries, RSC Adv., 2017, 7, 2637–2643 RSC
. - J. Guo, Y. Yang, W. Yu, X. Dong, J. Wang, G. Liu and T. Wang, Synthesis of α-Fe2O3, Fe3O4 and Fe2N magnetic hollow nanofibers as anode materials for Li-ion batteries, RSC Adv., 2016, 6, 111447–111456 RSC
. - J. H. Kim, Y. J. Hong, Y. C. Kang, Y. J. Choi and Y. S. Kim, Superior electrochemical properties of α-Fe2O3 nanofibers with a porous core/dense shell structure formed from iron acetylacetonate–polyvinylpyrrolidone composite fibers, Electrochim. Acta, 2015, 154, 211–218 CrossRef CAS
. - U. Boesenberg, M. A. Marcus, A. K. Shukla, T. Yi, E. McDermott, P. F. Teh, M. Srinivasan, A. Moewes and J. Cabana, Asymmetric pathways in the electrochemical conversion reaction of NiO as battery electrode with high storage capacity, Sci. Rep., 2014, 4, 7133 CrossRef CAS PubMed
. - H. Lai, Q. Wu, J. Zhao, L. Shang, H. Li, R. Che, Z. Lyu, J. Xiong, L. Yang, X. Wang and Z. Hu, Mesostructured NiO/Ni composites for high-performance electrochemical energy storage, Energy Environ. Sci., 2016, 9, 2053–2060 RSC
. - S. H. Park and W. J. Lee, Hierarchically mesoporous carbon nanofiber/Mn3O4 coaxial nanocables as anodes in lithium ion batteries, J. Power Sources, 2015, 281, 301–309 CrossRef CAS
. - H. Liu, Z. H. Li, Y. R. Liang, R. W. Fu and D. C. Wu, Facile synthesis of MnO multi-core@ nitrogen-doped carbon shell nanoparticles for high performance lithium-ion battery anodes, Carbon, 2015, 84, 419–425 CrossRef CAS
. - J. Z. Zhao, Z. L. Tao, J. Liang and J. Chen, Facile synthesis of nanoporous γ-MnO2 structures and their application in rechargeable Li-ion batteries, Cryst. Growth Des., 2008, 8, 2799–2805 CrossRef CAS
. - C. Yan, G. Chen, X. Zhou, J. Sun and C. Lv, Template-based engineering of carbon-doped Co3O4 hollow nanofibers as anode materials for lithium-ion batteries, Adv. Funct. Mater., 2016, 26, 1428–1436 CrossRef CAS
. - S. Abouali, M. Akbari Garakani, B. Zhang, H. Luo, Z. L. Xu, J. Q. Huang, J. Huang and J. K. Kim, Co3O4/porous electrospun carbon nanofibers as anodes for high performance Li-ion batteries, J. Mater. Chem. A, 2014, 2, 16939–16944 RSC
. - X. Rui, H. Tan, D. Sim, W. Liu, C. Xu, H. H. Hng, R. Yazami, T. M. Lim and Q. Yan, Template-free synthesis of urchin-like Co3O4 hollow spheres with good lithium storage properties, J. Power Sources, 2013, 222, 97–102 CrossRef CAS
. - L. Li, A. R. O. Raji and J. M. Tour, Graphene-wrapped MnO2-graphene nanoribbons as anode materials for high-performance lithium ion batteries, Adv. Mater., 2013, 25, 6298–6302 CrossRef CAS PubMed
. - Y. Wu, X. Li, Q. Xiao, G. Lie, Z. Lie and J. Guan, The coaxial MnO2/CNTs nanocomposite freestanding membrane on SSM substrate as anode materials in high performance lithium ion batteries, J. Electroanal. Chem., 2019, 834, 161–166 CrossRef CAS
. - Z. Cao, X. Chen, L. Xing, Y. Liao, M. Xu, X. Li, X. Liu and W. Li, Nano-MnO2@TiO2 microspheres: a novel structure and excellent performance as anode of lithium-ion batteries, J. Power Sources, 2018, 379, 174–181 CrossRef CAS
. - R. Saravanan, S. Agarwal, V. K. M. M. Khan, F. Gracia, E. Mosquera, V. Narayanan and A. Stephen, Line defect Ce3+ induced Ag/CeO2/ZnO nanostructure for visible-light photocatalytic activity, J. Photochem. Photobiol., A, 2018, 353, 499–506 CrossRef CAS
. - D. Xiao, C. Lu, C. Chen and S. Yuan, CeO2-webbed carbon nanotubes as a highly efficient sulfur host for lithium-sulfur batteries, Energy Storage Mater., 2018, 10, 216–222 CrossRef
. - Q. Hao, G. Cui, Y. Tian, T. Tan and Y. Zhang, Three-dimensional S/CeO2/rGO composites as cathode materials for lithium–sulfur batteries, Materials, 2018, 11, 1720 CrossRef PubMed
. - M. Li, X. Fan, X. Xiao, X. Huang, Y. Jiang and L. Chen, Ternary perovskite nickel titanate/reduced graphene oxide nano-composite with improved lithium storage properties, RSC Adv., 2016, 6, 61312–61318 RSC
. - C. Yuan, H. Wang, J. Liu, Q. Wu, Q. Duan and Y. Li, Facile synthesis of Co3O4-CeO2 composite oxide nanotubes and their multifunctional applications for lithium ion batteries and CO oxidation, J. Colloid Interface Sci., 2017, 494, 274–281 CrossRef CAS PubMed
. - S. Phokha, S. Hunpratub, N. Chanlek, S. Sonsupap and S. Maensiri, Synthesis, characterization and electrochemical performance of carbo/Ni-doped CeO2 composites, J. Alloys Compd., 2018, 750, 788–797 CrossRef CAS
. - B. G. S. Raj, A. M. Asiri, J. J. Wu and S. Anandan, Synthesis of Mn3O4 nanoparticles via chemical precipitation approach for supercapacitor application, J. Alloys Compd., 2015, 636, 234–240 CrossRef
. - S. Zhang, Y. Zhao, M. D. Somoano, J. Yang and J. Zhang, Synergistic mercury removal over the CeMnO3 perovskite structure oxide as an SCR catalyst from coal combustion flue gas, Energy Fuels, 2018, 32(11), 11785–11795 CrossRef CAS
. - H. Yi, J. Xu, X. Tang, S. Zhao, Y. Zhang, Z. Yang, J. Wu, X. Meng, J. Meng, H. Yan and Q. Li, Novel synthesis of Pd-CeMnO3 perovskite based on unique ultrasonic intervention from combination of Sol–Gel and impregnation method for low temperature efficient oxidation of benzene vapour, Ultrason. Sonochem., 2018, 48, 418–423 CrossRef CAS PubMed
. - H. Li, G. Lu, Q. Dai, Y. Wang, Y. Guo and Y. Guo, Efficient low-temperature catalytic combustion of trichloroethylene over flower-like mesoporous Mn-doped CeO2 microspheres, Appl. Catal., B, 2011, 102, 475–483 CrossRef CAS
. - J. Bhagwan, A. Sahoo, K. L. Yadav and Y. Sharm, Nanofibers of spinel-CdMn2O4: a new and high performance material for supercapacitor and Li-ion batteries, J. Alloys Compd., 2017, 703, 86–95 CrossRef CAS
. - C. Li, R. Chen, X. Zhang, S. Shu, J. Xiong, Y. Zheng and W. Dong, Electrospinning of CeO2–ZnO composite nanofibers and their photocatalytic property, Mater. Lett., 2011, 65, 1327–1330 CrossRef CAS
. - Y. Zhang, J. Li, Q. Li, L. Zhu, X. Liu, X. Zhong, J. Meng and X. Cao, Preparation of CeO2–ZrO2 ceramic fibers by electrospinning, J. Colloid Interface Sci., 2007, 307, 567–571 CrossRef CAS PubMed
. - X. Hu, Y. Ji, M. Wang, F. Miao, H. Ma, H. Shen and N. Jia, Water-Soluble and Biocompatible MnO@PVP Nanoparticles for MR Imaging In Vitro and In Vivo, J. Biomed. Nanotechnol., 2013, 9, 976–984 CrossRef CAS
. - Q. Cui, X. Dong, J. Wang and M. Li, Direct fabrication of cerium oxide hollow nanofibers by electrospinning, J. Rare Earths, 2008, 26, 664–669 CrossRef
. - Z. A. Elsiddig, D. Wang, H. Xu, W. Zhang, T. Zhang, P. Zhang, W. Tian, Z. Sun and J. Chen, Three-dimensional nitrogen-doped graphene wrapped LaMnO3 nanocomposites as high-performance supercapacitor electrodes, J. Alloys Compd., 2018, 740, 148–155 CrossRef CAS
. - A. Younis, D. W. Chu and S. A. Li, Oxygen level: the dominant of resistive switching characteristics in cerium oxide thin films, J. Phys. D: Appl. Phys., 2012, 45, 355101 CrossRef
. - L. R. Shah, B. Ali, H. Zhu, W. G. Wang, Y. Q. Song, H. W. Zhang, S. I. Shah and J. Q. Xiao, Detailed study on the role of oxygen vacancies in structural, magnetic and transport behavior of magnetic insulator: Co–CeO2, J. Phys.: Condens. Matter, 2009, 21, 486004 CrossRef PubMed
. - M. Kumar, J. H. Yun, V. Bhatt, B. Singh, J. Kim, J. S. Kim, B. S. Kim and C. Y. Lee, Role of Ce3+ valence state and surface oxygen vacancies on enhanced electrochemical performance of single step solvothermally synthesized CeO2 nanoparticles, Electrochim. Acta, 2018, 284, 709–720 CrossRef CAS
. - D. R. Mullins, S. H. Overbury and D. R. Huntley, Electron spectroscopy of single crystal and polycrystalline cerium oxide surfaces, Surf. Sci., 1998, 409, 307–319 CrossRef CAS
. - A. Krittayavathananon, T. Pettong, P. Kidkhunthod and M. Sawangphruk, Insight into the charge storage mechanism and capacity retention fading of MnCo2O4 used as supercapacitor electrodes, Electrochim. Acta, 2017, 258, 1008–1015 CrossRef CAS
. - G. Praline, B. E. Koel, R. L Hance, H. Lee and J. M. White, X-ray photoelectron study of the reaction of oxygen with cerium, J. Electron Spectrosc. Relat. Phenom., 1980, 21, 17–30 CrossRef CAS
. - C. Wang, D. L. Wang, Q. M. Wang and H. J. Chen, Fabrication and lithium storage performance of three-dimensional porous NiO as anode for lithium-ion battery, J. Power Sources, 2010, 195, 7432–7437 CrossRef CAS
. - J. Wang, D. Jin, R. Zhou, X. Li, X. Liu, C. Shen, K. Xie, B. Li, F. Kang and B. Wei, Highly flexible graphene/Mn3O4 nanocomposite membrane as advanced anodes for Li-ion batteries, ACS Nano, 2016, 10, 6227–6234 CrossRef CAS PubMed
. - X. Gu, J. Yue, L. Li, H. Xue, J. Yang and X. Zhao, General synthesis of MnOx (MnO2, Mn2O3, Mn3O4, MnO) hierarchical microspheres as lithium-ion battery anodes, Electrochim. Acta, 2015, 184, 250–256 CrossRef CAS
. - X. Xu, R. Cao, S. Jeong and J. Cho, Spindle-like mesoporous α-Fe2O3 anode material prepared from MOF template for high-rate lithium batteries, Nano Lett., 2012, 12, 4988–4991 CrossRef CAS PubMed
. - C. Peng, B. Chen, Y. Qin, S. Yang, C. Li, Y. Zuo, S. Liu and Y. Yang, Facile ultrasonic synthesis of CoO quantum dot/graphene nanosheet composites with high lithium storage capacity, ACS Nano, 2012, 6, 1074–1081 CrossRef CAS PubMed
. - H. Liu and Q. Le, Synthesis and performance of cerium oxide as anode materials for lithium ion batteries by a chemical precipitation method, J. Alloys Compd., 2016, 669, 1–7 CrossRef CAS
. - Y. Cho, P. Oh and J. Cho, A new type of protective surface layer for high-capacity Ni-based cathode materials: nanoscaled surface pillaring layer, Nano Lett., 2013, 13, 1145–1152 CrossRef CAS
. - K. B. Hatzell, M. Beidaghi, J. W. Campos, C. R. Dennison, E. C. Kumbur and Y. Gogotsi, A high performance pseudocapacitive suspension electrode
for the electrochemical flow capacitor, Electrochim. Acta, 2013, 111, 888–897 CrossRef CAS
. - C. Hua, X. Fang, Z. Wang and L. Chen, Lithium storage in perovskite lithium lanthanum titanate, Electrochem. Commun., 2013, 32, 5–8 CrossRef CAS
. - Y. Wang, X. Wen, J. Chen and S. Wang, Foamed mesoporous carbon/silicon composite nanofiber anode for lithium ion batteries, J. Power Sources, 2015, 281, 285–292 CrossRef CAS
.
Footnote |
† These authors contributed equally to this work. |
|
This journal is © The Royal Society of Chemistry 2019 |