DOI:
10.1039/C9RA06113D
(Paper)
RSC Adv., 2019,
9, 32977-32983
Gram-scale synthesis of nitrogen doped graphene quantum dots for sensitive detection of mercury ions and L-cysteine†
Received
6th August 2019
, Accepted 26th September 2019
First published on 16th October 2019
Abstract
Sensitive and reliable detection of mercury ions (Hg2+) and L-cysteine (L-Cys) is of great significance for toxicology assessment, environmental protection, food analysis and human health. Herein, we present gram-scale synthesis of nitrogen doped graphene quantum dots (N-GQDs) for sensitive detection of Hg2+ and L-Cys. The N-GQDs are one-step synthesized using bottom-up molecular fusion in a hydrothermal process with gram-scale yield at a single run. N-GQDs exhibit good structural characteristics including uniform size (∼2.1 nm), high crystallinity, and single-layered graphene thickness. Successful doping of N atom enables bright blue fluorescence (absolute photoluminescence quantum yield of 24.8%) and provides unique selectivity towards Hg2+. Based on the fluorescence quenching by Hg2+ (turn-off mode), N-GQDs are able to serve as an effective fluorescent probe for sensitive detection of Hg2+ with low limit of detection (19 nM). As L-Cys could recover the fluorescence of N-GQDs quenched by Hg2+, fluorescent detection of L-Cys is also demonstrated using turn-off-on mode.
1. Introduction
Metal ions and small biomarkers play crucial roles in various physiological and pathological processes.1,2 For instance, mercury ions (Hg2+) are one of the most toxic pollutants and are a great threat to the environment and human health. Even a small intake of Hg2+ (2 mg kg−1 body weight per day) can damage the nervous, endocrine and other systems, which further leads to many fatal diseases (e.g. headaches, renal failure, loss of intelligence quotient).3–5 On the other hand, as one of the essential amino acids and small biomarkers in human body, L-cysteine (L-Cys) possesses vital physiological functions in neuronal tissues, metabolism and detoxification.6–10 Abnormal levels of L-Cys can cause growth retardation, neurotoxic effect, Alzheimer's disease and coronary heart disease.8–10 Therefore, simple and sensitive detection of Hg2+ and L-Cys is of great importance.
Up to now, many methods including atomic absorption spectrometry (AAS), atomic fluorescence spectrometry (AFS), inductively-coupled plasma mass spectrometry (ICP-MS) and surface-enhanced Raman scattering (SERS) have been developed for the detection of Hg2+.1,11,12 However, these methods often require highly extensive instruments and sophisticated operation techniques. Several strategies including high performance liquid chromatography (HPLC) and capillary electrophoresis (CE) have been designed to detect L-Cys.7,10 Tedious and time-consuming procedures are usually required. Among various detection techniques, fluorescence detections have attracted considerable interests owing to the ease of operation, rapid detection, high sensitivity, and possibility for real-time monitoring and local observation.8,9,13,14 Until now, considerable efforts have been devoted to the development of fluorescent probes for monitoring Hg2+ or L-Cys.
Graphene quantum dots (GQDs) or 0D graphene are the newest addition to the nanocarbon family.15–17 Owing to its atomic thickness, nanometer scale (<10 nm) and quantum confinement effect, GQDs exhibit tunable photoluminescence, high photostability, low cytotoxicity, and superior water dispersibility.18,19 These unique properties promise a wide range of applications in biological imaging,15,20 catalysis,21–24 drug delivery,19 energy conversion and storage22,25 and especially sensing.21,26–32 In recent years, it has been proved that heteroatom doping can effectively regulate the property and selectivity of GQDs. Challenge remains in facile and gram-scale synthesis of heteroatom-doped GQDs with specific selectivity.
In this work, we demonstrate gram-scale synthesis of nitrogen doped graphene quantum dots (N-GQDs) for sensitive detection of Hg2+ and L-Cys. As illustrated in Fig. 1, N-GQDs were easily synthesized through one-step bottom-up strategy under hydrothermal condition. The as-prepared N-GQDs show bright and emission-independent fluorescence, uniform size, and good crystalline. Owing to specific electron transfer and aggregation, N-GQDs exhibit selective and sensitive fluorescence quenching towards Hg2+. In presence of L-Cys, the quenched fluorescence of N-GQDs/Hg2+ system could be recovered. Based on these fluorescence quenching (turn-off) and recovery (turn-off-on) processes, sensitive and simultaneous detection of Hg2+ and L-Cys are demonstrated.
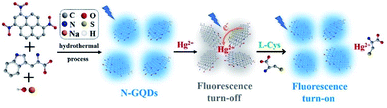 |
| Fig. 1 Schematic illustration for the preparation of N-GQDs and detection of Hg2+ and L-Cys. | |
2. Experimental
2.1 Materials and reagents
Pyrene, 4-(2-hydroxyethyl)-1-piperazineethanesulfonic acid (HEPES), NaOH, L-cysteine (L-Cys), L-histidine (His), D-methionine (Met), L-tryptophan, L-tyrosine (Tyr), L-leucine (Leu), L-threonine (Thr), L-alanine (Ala), L-glutamic acid (Glu), L-aspartic acid (Asp) and glycine (Gly) were obtained from Aladdin Chemistry Co. Ltd. (China). The standard solution of Hg2+ was purchased from National Standard Materials Center (China). Aqueous solutions of other cations were prepared from chloride salts (Ni2+, K+, Na+, Ca2+), nitrate salts (Zn2+, Cr3+, Ag+, Co2+, Cd2+, Pb2+, Fe3+), and sulfate salts (Mg2+, Cu2+, Al3+), respectively. All reagents are of analytical grade and used with further treatment. All aqueous solutions were prepared with ultrapure water (18.2 MΩ cm, Milli-Q, Millipore).
2.2 Characterizations
Transmission electron microscopic (TEM) images was taken on a JEM-2100 transmission electron microscope at operating voltage of 200 kV (JEOL Ltd., Japan). Atomic force microscopic (AFM) images were obtained using tapping mode by Bruker Multimode 8 (Bruker. Inc, USA). Elemental analysis of the N-GQDs was performed by X-ray photoelectron spectroscopy (XPS) with PHI5300 electron spectrometer (PE Ltd., USA) using Mg Kα radiation (250 W, 14 kV). Fluorescence spectra were recorded on RF-5301 PC spectrofluorometer (Shimadzu Corporation, Japan). Absolute photoluminescence quantum yield was measured using FL 3C-11 spectrofluorometer (Hariba Scientific, USA).
2.3 One-step preparation of N-GQDs
The precursor, 1,3,6-trinitropyrene, was synthesized according to the literature.17 N-GQDs were prepared using one-pot hydrothermal process. Briefly, 1,3,6-trinitropyrene (6 mM) and L-tryptophan (75 mM) were dissolved in NaOH (125 mM) with ultrasonic treatment for 0.5 h. The mixture was then transferred into 500 mL Teflon-lined autoclave and heated for 6 h at 200 °C. To optimize the preparation conditions, different concentrations of L-tryptophan (25–120 mM) and NaOH (5 mM to 0.5 M), reaction temperature (120–200 °C) and time (2–10 h) were used. After the reaction, the obtained solution was filtered through a 0.22 μm microporous membrane to remove insoluble carbon product. N-GQDs could be obtained by freeze-drying after dialysis (retained molecular weight of 1000 Da) for 24 h to remove unreacted small molecules. The synthesis yield for N-GQDs was calculated based on the precursor.
2.4 Fluorescent turn-off detection of Hg2+
The HEPES buffer solution (0.1 M, pH 6.0) containing tartaric acid (TA, 125 μM) was used for the detection of Hg2+. N-GQDs (0.07 mg mL−1) were mixed with Hg2+ at different concentrations (0.05–25 μM). After incubation at room temperature for 5 min, the fluorescence (FL) intensity in the absence (F0) and presence (F) of Hg2+ was measured with the excitation wavelength fixed at 370 nm. The relative fluorescence ratio (F/F0) and fluorescence quenching ratio (F0 − F)/F0 were used for calibration. Each detection was performed in triplicate.
For real sample analysis, the environmental sample was collected from Jinsha Lake (Hangzhou, China). Bacteria, algae and insoluble substances were removed by a 0.22 μm microporous membrane. The detection of Hg2+ was evaluated by standard addition method.
2.5 Fluorescence turn-off-on detection of L-Cys
As L-Cys can recover the fluorescence of N-GQDs quenched by Hg2+, fluorescence turn-off-on mode was applied to detect L-Cys. Briefly, N-GQDs (0.07 mg mL−1) was firstly mixed with Hg2+ (25 μM) and incubated for 5 min. Then, L-Cys was added at different concentrations (0.1–30.0 μM). After the mixed solution was incubated at room temperature for 5 min, the FL intensity was recorded (excited at 370 nm). The relative fluorescence recovery ratio (F1 − F)/F1 was used to evaluate the fluorescence recovery by L-Cys, where F and F1 represent the FL intensity in absence and presence of L-Cys, respectively.
3. Results and discussion
3.1 Gram-scale synthesis of N-GQDs
In present work, N-GQDs were synthesized using one-step hydrothermal fusion between trinitropyrene and tryptophan in NaOH medium (Fig. 1). Trinitropyrene was chosen as precursor because it has the same mother-nucleus structure as graphene and could be fused at alkaline medium. Tryptophan with N atoms and carboxyl groups was applied as dopant to introduce doped heteroatoms as well as functional groups on GQDs. As demonstrated in inset of Fig. 2A, GQDs with blue fluorescence are obtained. In order to obtain GQDs with excellent fluorescent properties, the conditions of hydrothermal reaction were optimized. The highest fluorescence (FL) intensity is obtained at a reaction of 6 h and 200 °C (Fig. S1A and S1B†). Obviously, high temperature can facilitate the efficiency of the bottom-up fusion. However, higher temperature was not investigated because it would have exceeded the tolerated range of the common Teflon-lined autoclave. Too short reaction time results in low concentration of GQDs due to incomplete molecule fusion, whereas too long time might result in GQDs stack. The FL intensity increases with the increase of tryptophan concentration and then reaches a plateau, presumably because the fusion between trinitropyrene and tryptophan is saturated (Fig. S1C†). The concentration of NaOH exhibits similar effects on the FL intensity of GQDs (Fig. S1D†). Thus, N-GQDs synthesized under the optimized conditions (75 mM L-tryptophan and 125 mM NaOH at 200 °C for 6 h) was applied for further investigation.
 |
| Fig. 2 (A) The FL excitation spectrum (EX, left) obtained at the emission wavelength of 445 nm and FL emission spectra (EM, right) of N-GQDs obtained with different excitation wavelength (340 to 410 nm, 10 nm increment). Insets are N-GQDs solution under daylight (left) and UV light (365 nm). (B) FL lifetime spectrum of N-GQDs. (C) TEM images of N-GQDs. Insets were the high-resolution TEM (HRTEM) image with indicated lattice parameter and size distribution of N-GQDs. (D) AFM image of the N-GQDs. Inset was the height profile along the red line. | |
The synthesis yield of obtained N-GQDs is as high as 91.2%, indicating high efficiency for the preparation of N-GQDs. It is worth noting that the volume of the autoclave in present work is 500 mL, which is significantly higher than that used in most GQDs synthesis (usually 25 mL or 50 mL volume). Even the reaction solution is only 40% of the volume of autoclave, more than 0.5 g of N-GQDs could be produced at a single run. Thus, gram-scale synthesis of N-GQDs could be realized using this green and one-step strategy.
3.2 Fluorescence property, morphology and composition of N-GQDs
Fig. 2A demonstrates the fluorescent excitation and emission spectra of N-GQDs. As shown, the maximum excitation at 370 nm gives the maximum emission at 445 nm. It is worth noting that the maximum FL emission of N-GQDs remains unchanged when the excitation wavelength changes. In addition, a narrow emission is revealed with the half-peak width of about 55 nm (excited at 370 nm). These emission-independent and narrow emission suggest that N-GQDs have uniform structure and luminescent states. The absolute quantum yield is 24.8% with a lifetime of 6.9 ns (Fig. 2B).
As revealed by transmission electron microscopy (TEM), the size of N-GQDs is uniform with average size of ∼2.1 nm. The crystallinity of N-GQDs was confirmed by high resolution TEM (HRTEM). The lattice spacing of 0.25 nm corresponds to the graphene (100) planes (Fig. 2C). Good crystallinity of N-GQDs might be attributed to the trinitropyrene precursor (Fig. 1), which has the mother-nucleus structure as graphene. The atomic force microscopic (AFM) image shows that the thickness of N-GQDs is about 1.0 nm. Considering the doped heteroatoms and surface groups, the as-prepared N-GQDs are mostly single-layered (Fig. 2D). The uniform size, good crystalline and homogeneous thickness suggest excellent structure characteristics of N-GQDs, which also support the narrow and emission-independent fluorescence.
The composition of N-GQDs was investigated by X-ray photoelectron spectroscopy (XPS). XPS survey spectrum reveals the presence of C, O, and N with atomic percentages of 78.7%, 17.3% and 4.0%, respectively (Fig. 3A), indicating successful introduction of N atoms on GQDs. The high resolution C 1s spectrum clearly evidences the presence of C–C
C (sp2 C, 284.6 eV), C–O/C–N (285.5 eV) and O–C
O (288.0 eV), respectively (Fig. 3B).17 According to the high resolution O 1s spectrum, N-GQDs contains carboxyl group (532.8 eV) and hydroxyl group (531.4 eV) (Fig. 3C).33 The pyrrole N (C–N–C, 398.7 eV) and graphite N (N–(C)3, 399.2 eV) in high resolution N 1s spectrum indicate that N atoms have successfully doped into the carbon skeleton of GQDs.34 In addition, N-GQDs also contain some primary amino groups (C–N–H, 400.1 eV) (Fig. 3D).
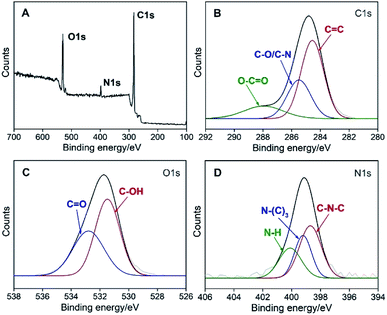 |
| Fig. 3 XPS survey spectrum (A) and high resolution C 1s (B), O 1s (C), and N 1s (D) spectra of N-GQDs. | |
3.3 Selective fluorescence quenching of N-GQDs towards Hg2+
The selectivity of N-GQDs towards different metal ions was investigated. As demonstrated in Fig. 4A, K+, Na+, Ca2+, Mg2+, Zn2+, Al3+, Ni2+, Co2+, Cr3+, Pb2+, Cd2+, and Ag+ hardly affect the fluorescence of N-GQDs. On the contrary, Hg2+, Fe3+ and Cu2+ could quench the fluorescence of N-GQDs. However, the quenching efficiency of Hg2+ is significantly higher than that of Fe3+ and Cu2+, indicating the potential for sensitive detection of Hg2+. In contrast to most reported GQDs-based fluorescent sensors, which usually applied to detect Fe3+ or Cu2+, GQDs with Hg2+ sensitivity is rarely reported. Tartaric acid (TA) is chosen as masking agent to eliminate the interference of Fe3+ and Cu2+. As shown in Fig. S2,† the addition of TA has no effect on the fluorescence of N-GQDs. In the presence of TA (125 μM), the fluorescence of N-GQDs quenched by Hg2+ is similar with that obtained in mixture of Hg2+ and all other ions in Fig. 4A (Fig. 4B). Hence, selective fluorescence response of N-GQDs towards Hg2+ could be achieved.
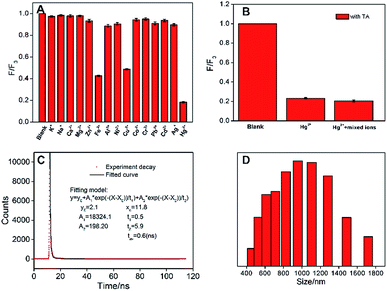 |
| Fig. 4 (A) The relative FL ratio of the N-GQDs in the presence of different metal ions (25 μM). (B) The relative FL ratio of the N-GQDs in the presence of Hg2+ or Hg2+ + mixed ions (all other ions in A) with tartaric acid (TA, 125 μM). (C) FL lifetime spectrum of N-GQDs in the presence of Hg2+. (D) The dynamic light scattering of N-GQDs in the presence of Hg2+. | |
The mechanism for the fluorescence quenching of N-GQDs by Hg2+ was investigated. Firstly, we measured the fluorescence lifetime of N-GQDs after interaction with Hg2+. The lifetime of N-GQDs in presence of Hg2+ is 0.6 ns (Fig. 4C), which is remarkably shorter than that of N-GQDs itself (6.9 ns). This indicates fluorescence quenching by electron transfer between N-GQDs and Hg2+, presumably because of the electron-donating moieties on GQDs formed by doped N atoms (Fig. 1). In addition, the remarkable change of particle size was also investigated by the dynamic light scattering (DLS). The average size of particles in N-GQDs-Hg2+ mixture is 1038.7 nm, indicating Hg2+-induced aggregation of N-GQDs. (Fig. 4D). Thus, aggregation-induced-quenching also occurs (Fig. 1).
3.4 Fluorescent turn-off detection of Hg2+ using N-GQDs as fluorescent probes
Owning to selective fluorescence quenching by Hg2+, fluorescent sensor (turn-off mode) was developed for the detection of Hg2+ using N-GQDs as fluorescent probe. To achieve the highest sensitivity, the detection conditions including pH and incubation time were optimized. As shown in Fig. 5A, the highest fluorescence quenching exists at pH 6. Too low a pH value (pH 3–5) might weaken the interaction between N-GQDs and metal ions through protonation of N atoms on GQDs whereas too high a pH value (pH 7) possibly converts metal ions into hydroxide. At the same time, fast kinetics is observed and a plateau of fluorescence intensity appeared within 3 min (Fig. 5B), indicating the strong interaction between N-GQDs and Hg2+. Under the optimized conditions, remarkable decrease in FL intensity towards different concentration of N-GQDs is observed (Fig. 5C). Good linearity existed between fluorescent quenching ratio (F0 − F)/F0 and Hg2+ concentration from 50 nM to 15 μM (Fig. 5D). The limit of detection (LOD) is 19 nM at a signal-to-noise ratio of 3. As shown in Table S1 (ESI†), the detection limit obtained with the present method was lower than those obtained by carbon dots (CDs),35 F doped CDs (F-CDs),36 N doped CDs (N-CDs),37 N and S co-doped CDs (N, S-CDs),38,39 N doped GQDs (N-GCDs),34 sulfur and nitrogen co-doped GQDs (S,N-GQDs),40 and N-GQDs,41 but higher than that obtained using Rhodamine B assisted GQDs (RhB-GQDs)42 and N and S co-doped GQDs (N, S-GQDs).43
 |
| Fig. 5 (A) The relative FL ratio of N-GQDs with Hg2+ at different pH. (B) The effect of incubation time on fluorescent quenching ratio of N-GQDs caused by Hg2+ (25 μM). (C) FL emission spectra of N-GQDs in the presence of different concentrations of Hg2+. (D) The linear dependence of (F0 − F)/F0 on concentration of Hg2+. | |
The application of the as-prepared fluorescent sensor for detection of Hg2+ in environmental samples (lake water) was investigated (Table 1). As the original Hg2+ is not detected, the standard addition method was applied to evaluate the detection reliability. Good recoveries ranged from 96.4% to 98.6% were obtained, indicating the potential application of N-GQDs in real analysis.
Table 1 Detection of Hg2+ in environmental water samples
Sample |
Concentration of (μM) |
RSD (n = 3, %) |
Recovery (%) |
Added Hg2+ |
Found by N-GQDs |
1 |
3.00 |
2.91, 2.83, 2.96 |
2.2 |
96.7 |
2 |
7.00 |
6.72, 6.65, 6.88 |
1.8 |
96.4 |
3 |
12.0 |
11.6, 11.9, 12.0 |
1.7 |
98.6 |
3.5 Detection of L-Cys based on fluorescent off-on mode
As illustrated in Fig. 6A, the fluorescence of N-GQDs quenched by Hg2+ could be recovered after addition of L-Cys to the N-GQDs-Hg2+ system. As shown in Fig. 6B, L-Cys itself has no effect on the fluorescence intensity of N-GQDs. The possible interference of other nine common amino acids on the detection of L-Cys was investigated. As shown in Fig. 6A, these amino acids could not significantly restore the fluorescence of N-GQDs quenched by Hg2+, indicating good selectivity of L-Cys. We speculate that the fluorescence recovery by L-Cys is due to the specific binding between –SH and Hg2+. In support of this notion, glutathione (GSH, tripeptide composed of Cys, glycine, and glutamic acid) and homocysteine (Hcy, only include an additional –CH2– before –SH in comparison with Cys) also recover the fluorescence to some extent because of the similar structure as Cys (Fig. S3†). Fluorescence turn-off-on sensing mode could be developed for individual detection of L-Cys as a proof-of-demonstration. As shown in Fig. 6C, the fluorescence of N-GQDs gradually restores with increasing the concentration of L-Cys. Good linear relationship is found between fluorescence recovery rate and the concentration of L-Cys from 100 nM to 50 μM (DL of 29 nM) (Fig. 6D). As shown in Table S2,† the detection limit obtained with the present method was lower than those obtained by N,S-CDs-Fe3+,44 N-CDs-Hg2+,47 Eu-GQDs-Cu2+,48 and N-GQDs-Hg2+,49 but higher than that obtained using CDs-Hg2+,45 N-CDs@V2O5,46 and N-GQDs-Hg2+.49 The application of the N-GQDs-Hg2+ system for detection of L-Cys in environmental samples (lake water) was investigated using standard addition method (Table S3†). Good recoveries ranged from 94.1% to 97.8% were obtained.
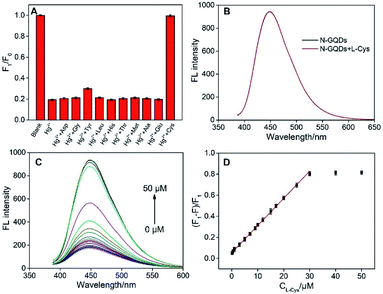 |
| Fig. 6 (A) The relative FL ratio of N-GQDs with Hg2+ in the presence of different amino acid. (B) FL emission spectra of N-GQDs in the absence and presence of L-Cys (50 μM). (C) FL emission spectra of N-GQDs with Hg2+ (25 μM) in the presence of different concentrations of L-Cys. (D) The linear dependence of (F1 – F)/F1 on concentration of L-Cys. | |
4. Conclusions
Gram-scale synthesis of nitrogen doped graphene quantum dots (N-GQDs) with Hg2+ sensitivity was readily achieved using one-step hydrothermal process. Such blue N-GQDs exhibit good characteristics including uniform size, good crystalline, bright and emission-independent fluorescence and single-layered graphene thickness. The ability to interact with Hg2+ results in selective and sensitive fluorescence quenching through electron transfer and aggregation. With N-GQDs being fluorescent probes, turn-off sensing of Hg2+ and turn-off-on detection of L-cysteine were demonstrated. The easy preparation and good characteristics of N-GQDs promise a large variety of applications, including optical sensing, photocatalysis and bioimaging.
Conflicts of interest
There are no conflicts to declare.
Acknowledgements
The authors gratefully acknowledge the financial support from the National Natural Science Foundation of China (No. 81860512), Guangxi Natural Science Foundation (2016GXNSFBA380194), and the Zhejiang Provincial Natural Science Foundation of China (LY19B050008).
References
- H. N. Kim, W. X. Ren, J. S. Kim and J. Yoon, Fluorescent and colorimetric sensors for detection of lead, cadmium, and mercury ions, Chem. Soc. Rev., 2012, 41, 3210–3244 RSC.
- M. Gao, X. Lu, S. Chen, D. Tian, Y. Zhu and C. Wang, Enhanced peroxidase-like activity of Mo6+-doped Co3O4 nanotubes for ultrasensitive and colorimetric L-cysteine detection, ACS Appl. Nano Mater., 2018, 1, 4703–4715 CrossRef CAS.
- Y. Liu, Y. Deng, T. Li, Z. Chen, H. Chen, S. Li and H. Liu, Aptamer-based electrochemical biosensor for mercury ions detection using AuNPs-modified glass carbon electrode, J. Biomed. Nanotechnol., 2018, 14, 2156–2161 CrossRef CAS.
- S. Y. Ding, M. Dong, Y. W. Wang, Y. T. Chen, H. Z. Wang, C. Y. Su and W. Wang, Thioether-based fluorescent covalent organic framework for selective detection and facile removal of mercury(II), J. Am. Chem. Soc., 2016, 138, 3031–3037 CrossRef CAS.
- G. H. Chen, W. Y. Chen, Y. C. Yen, C. W. Wang, H. T. Chang and C. F. Chen, Detection of mercury(II) ions using colorimetric gold nanoparticles on paper-based analytical devices, Anal. Chem., 2014, 86, 6843–6849 CrossRef CAS.
- M. Gao, X. Lu, M. Chi, S. Chen and C. Wang, Fabrication of oxidase-like hollow MnCo2O4 nanofibers and their sensitive colorimetric detection of sulfite and L-cysteine, Inorg. Chem. Front., 2017, 4, 1862–1869 RSC.
- H. Li, L. Ye, Y. Wang and C. Xie, A glassy carbon electrode modified with hollow cubic cuprous oxide for voltammetric sensing of L-cysteine, Microchim. Acta, 2018, 185, 5 CrossRef.
- X. Li, X. Y. Yang, J. Q. Sha, T. Han, C. J. Du, Y. J. Sun and Y. Q. Lan, POMOF/SWNT nanocomposites with prominent peroxidase-mimicking activity for L-cysteine “on-off switch” colorimetric biosensing, ACS Appl. Mater. Interfaces, 2019, 11, 16896–16904 CrossRef CAS.
- Y. C. Liang, Q. Zhao, X. Y. Wu, Z. Li, Y. J. Lu, Q. Liu, L. Dong and C. X. Shan, A ratiometric fluorescent nanoprobe based on quenched carbon dots-rhodamine B for selective detection of L-cysteine, J. Alloys Compd., 2019, 788, 615–622 CrossRef CAS.
- Y. Zhu, Z. Xu, K. Yan, H. Zhao and J. Zhang, One-Step Synthesis of CuO-Cu2O heterojunction by flame spray pyrolysis for cathodic photoelectrochemical sensing of L-cysteine, ACS Appl. Mater. Interfaces, 2017, 9, 40452–40460 CrossRef CAS.
- J. Wu, P. Ran, S. Zhu, F. Mo, C. Wang and Y. Fu, A highly sensitive electrochemiluminescence sensor for the detection of L-cysteine based on the rhombus-shaped rubrene microsheets and platinum nanoparticles, Sens. Actuators, B, 2019, 278, 97–102 CrossRef CAS.
- Y. Guo, Z. Wang, H. Shao and X. Jiang, Hydrothermal synthesis of highly fluorescent carbon nanoparticles from sodium citrate and their use for the detection of mercury ions, Carbon, 2013, 52, 583–589 CrossRef CAS.
- P. Makam, R. Shilpa, A. E. Kandjani, S. R. Periasamy, Y. M. Sabri, C. Madhu, S. K. Bhargava and T. Govindaraju, SERS and fluorescence-based ultrasensitive detection of mercury in water, Biosens. Bioelectron., 2018, 100, 556–564 CrossRef CAS.
- F. Yan, Y. Zou, M. Wang, X. Mu, N. Yang and L. Chen, Highly photoluminescent carbon dots-based fluorescent chemosensors for sensitive and selective detection of mercury ions and application of imaging in living cells, Sens. Actuators, B, 2014, 192, 488–495 CrossRef CAS.
- Y. Yan, J. Gong, J. Chen, Z. Zeng, W. Huang, K. Pu, J. Liu and P. Chen, Recent advances on graphene quantum dots: From chemistry and physics to applications, Adv. Mater., 2019, 31, e1808283 CrossRef.
- Y. Du and S. Guo, Chemically doped fluorescent carbon and graphene quantum dots for bioimaging, sensor, catalytic and photoelectronic applications, Nanoscale, 2016, 8, 2532–2543 RSC.
- L. Wang, Y. Wang, T. Xu, H. Liao, C. Yao, Y. Liu, Z. Li, Z. Chen, D. Pan, L. Sun and M. Wu, Gram-scale synthesis of single-crystalline graphene quantum dots with superior optical properties, Nat. Commun., 2014, 5, 5357 CrossRef CAS.
- S. Zhu, Y. Song, J. Wang, H. Wan, Y. Zhang, Y. Ning and B. Yang, Photoluminescence mechanism in graphene quantum dots: Quantum confinement effect and surface/edge state, Nano Today, 2017, 13, 10–14 CrossRef CAS.
- X. Yao, X. Niu, K. Ma, P. Huang, J. Grothe, S. Kaskel and Y. Zhu, Graphene quantum dots-capped magnetic mesoporous silica nanoparticles as a multifunctional platform for controlled drug delivery, magnetic hyperthermia, and photothermal therapy, Small, 2017, 13, 1602225 CrossRef.
- J. Dong, K. Wang, L. Sun, B. Sun, M. Yang, H. Chen, Y. Wang, J. Sun and L. Dong, Application of graphene quantum dots for simultaneous fluorescence imaging and tumor-targeted drug delivery, Sens. Actuators, B, 2018, 256, 616–623 CrossRef CAS.
- Y. Yan, J. Chen, N. Li, J. Tian, K. Li, J. Jiang, J. Liu, Q. Tian and P. Chen, Systematic bandgap engineering of graphene quantum dots and applications for photocatalytic water splitting and CO2 reduction, ACS Nano, 2018, 12, 3523–3532 CrossRef CAS.
- J. Tian, J. Chen, J. Liu, Q. Tian and P. Chen, Graphene quantum dot engineered nickel-cobalt phosphide as highly efficient bifunctional catalyst for overall water splitting, Nano Energy, 2018, 48, 284–291 CrossRef CAS.
- F. Xi, J. Zhao, C. Shen, J. He, J. Chen, Y. Yan, K. Li, J. Liu and P. Chen, Amphiphilic graphene quantum dots as a new class of surfactants, Carbon, 2019, 153, 127–135 CrossRef CAS.
- Z. Zeng, F. Xiao, H. Phan, S. Chen, Z. Yu, R. Wang, T. Nguyen and T. Tan, Unraveling the cooperative synergy of zero-dimensional graphene quantum dots and metal nanocrystals enabled by layer-by-layer assembly, J. Mater. Chem. A, 2018, 6, 1700–1713 RSC.
- Q. Wang, Z. Jin, D. Chen, D. Bai, H. Bian, J. Sun, G. Zhu, G. Wang and S. F. Liu, μ-Graphene crosslinked CsPbI3 quantum dots for high efficiency solar cells with much improved stability, Adv. Energy Mater., 2018, 8, 1800007 CrossRef.
- C. Shen, S. Ge, Y. Pang, F. Xi, J. Liu, X. Dong and P. Chen, Facile and scalable preparation of highly luminescent N,S co-doped graphene quantum dots and their application for parallel detection of multiple metal ions, J. Mater. Chem. B, 2017, 5, 6593–6600 RSC.
- S. Bian, C. Shen, Y. Qian, J. Liu, F. Xi and X. Dong, Facile synthesis of sulfur-doped graphene quantum dots as fluorescent sensing probes for Ag+ ions detection, Sens. Actuators, B, 2017, 242, 231–237 CrossRef CAS.
- D. Chen, X. Zhuang, J. Zhai, Y. Zheng, H. Lu and L. Chen, Preparation of highly sensitive Pt nanoparticles-carbon quantum dots/ionic liquid functionalized graphene oxide nanocomposites and application for H2O2 detection, Sens. Actuators, B, 2018, 255, 1500–1506 CrossRef CAS.
- B. Y. Fang, C. Li, Y. Y. Song, F. Tan, Y. C. Cao and Y. D. Zhao, Nitrogen-doped graphene quantum dot for direct fluorescence detection of Al3+ in aqueous media and living cells, Biosens. Bioelectron., 2018, 100, 41–48 CrossRef CAS.
- S. Bian, C. Shen, H. Hua, L. Zhou, H. Zhu, F. Xi, J. Liu and X. Dong, One-pot synthesis of sulfur-doped graphene quantum dots as a novel fluorescent probe for highly selective and sensitive detection of lead(II), RSC Adv., 2016, 6, 69977–69983 RSC.
- L. Lu, L. Zhou, J. Chen, F. Yan, J. Liu, X. Dong, F. Xi and P. Chen, Nanochannel confined graphene quantum dots for ultrasensitive electrochemical analysis of complex samples, ACS Nano, 2018, 12, 12673–12681 CrossRef CAS.
- J. Ju, R. Zhang and W. Chen, Photochemical deposition of surface-clean silver nanoparticles on nitrogen-doped graphene quantum dots for sensitive colorimetric detection of glutathione, Sens. Actuators, B, 2016, 228, 66–73 CrossRef CAS.
- Q. Xiao, S. Lu, C. Huang, W. Su and S. Huang, Novel N-doped carbon dots/beta-cyclodextrin nanocomposites for enantioselective recognition of tryptophan enantiomers, Sensors, 2016, 16, 1874 CrossRef.
- Z. Yan, X. Qu, Q. Niu, C. Tian, C. Fan and B. Ye, A green synthesis of highly fluorescent nitrogen-doped graphene quantum dots for the highly sensitive and selective detection of mercury(II) ions and biothiols, Anal. Methods, 2016, 8, 1565–1571 RSC.
- H. Goncalves, A. Duarte and J. Silva, Optical fiber sensor for Hg (II) based on carbon dots, Biosens. Bioelectron., 2010, 26, 1302–1306 CrossRef CAS.
- Z. Gao, Z. Lin, X. Chen, Z. Lai and Z. Huang, Carbon dots-based fluorescent probe for trace Hg2+ detection in water sample, Sens. Actuators, B, 2016, 222, 965–971 CrossRef CAS.
- R. Zhang and W. Chen, Nitrogen-doped carbon quantum dots: Facile synthesis and application as a “turn-off” fluorescent probe for detection of Hg2+ ions, Biosens. Bioelectron., 2014, 55, 83–90 CrossRef CAS PubMed.
- Y. Wang, S. Kim and L. Feng, Highly luminescent N, S-co-doped carbon dots and their direct use as mercury (II) sensor, Anal. Chim. Acta, 2015, 890, 134–142 CrossRef CAS.
- L. Li, B. Yu and T. You, Nitrogen and sulfur co-doped carbon dots for highly selective and sensitive detection of Hg (II) ions, Biosens. Bioelectron., 2015, 74, 263–269 CrossRef CAS.
- E. Sharma, D. Vashisht, A. Vashisht, V. Vats, S. Mehta and K. Singh, Facile synthesis of sulfur and nitrogen codoped graphene quantum dots for optical sensing of Hg and Ag ions, Chem. Phys. Lett., 2014, 55, 83–90 Search PubMed.
- Y. Liu, X. Tang, M. Deng, Y. Cao, Y. Li, H. Zhang, F. Li, F. Yan, T. Lan, L. Shi, L. Gao, L. Huang, T. Zhu, H. Lin, Y. Bai, D. Qu, X. Huang and F. Qiu, Nitrogen doped graphene quantum dots as a fluorescent probe for mercury(II) ions, Microchim. Acta, 2019, 186, 140–147 CrossRef.
- Y. Yang, X. Xiao, X. Xing, Z. Wang, T. Zou, Z. Wang, R. Zhao and Y. Wang, Rhodamine B assisted graphene quantum dots flourescent sensor system for sensitive recognition of mercury ions, J. Lumin., 2019, 207, 273–281 CrossRef CAS.
- N. Anh, A. Chowdhury and R. Doong, Highly sensitive and selective detection of mercury ions using N, S-codoped graphene quantum dots and its paper strip based sensing application in wastewater, Sens. Actuators, B, 2017, 252, 1169–1178 CrossRef.
- H. Wu, J. Jiang, X. Gu and C. Tong, Nitrogen and sulfur co-doped carbon quantum dots for highly selective and sensitive fluorescent detection of Fe (III) ions and L-cysteine, Microchim. Acta, 2017, 184(7), 2291–2298 CrossRef CAS.
- F. Yan, D. Shi, T. Zheng, K. Yun and X. Zhou, Carbon dots as nanosensor for sensitive and selective detection of Hg2+ and L-Cysteine by means of fluorescence “Off-On” switching, Sens. Actuators, B, 2016, 224, 926–935 CrossRef CAS.
- A. B. Ganganboina, A. C. Dutta and R. A. Doong, N-doped graphene quantum dots-decorated V2O5 nanosheet for fluorescence turn off-on detection of cysteine, ACS Appl. Mater. Interfaces, 2018, 10, 614–624 CrossRef CAS.
- H. Huang, Y. Weng, L. Zheng, B. Yao, W. Weng and X. Lin, Nitrogen-doped carbon quantum dots as fluorescent probe for “off-on” detection of mercury ions, L-cysteine and iodide ions, J. Colloid Interface Sci., 2017, 506, 373–378 CrossRef CAS.
- L. Lin, X. Song, Y. Chen, M. Rong, Y. Wang, L. Zhao, T. Zhao and X. Chen, Europium-decorated graphene quantum dots as a fluorescent probe for label-free, rapid and sensitive detection of Cu2+ and L-cysteine, Anal. Chim. Acta, 2015, 891, 261–268 CrossRef CAS.
- Z. Liu, Y. Gong and Z. Fan, Cysteine detection using a high-fluorescence sensor based on a nitrogen-doped graphene quantum dot-mercury(II) system, J. Lumin., 2016, 175, 129–134 CrossRef CAS.
Footnotes |
† Electronic supplementary information (ESI) available. See DOI: 10.1039/c9ra06113d |
‡ The two authors contributed equally. |
|
This journal is © The Royal Society of Chemistry 2019 |