DOI:
10.1039/C9RA04005F
(Paper)
RSC Adv., 2019,
9, 26773-26779
Highly sensitive polyaniline-coated fiber gas sensors for real-time monitoring of ammonia gas†
Received
27th May 2019
, Accepted 18th August 2019
First published on 27th August 2019
Abstract
A single-yarn-based gas sensor has been made from conductive polyaniline coated on commercial yarns. This can detect ammonia gas concentration in an environment or a working area. Cotton, rayon and polyester are utilized as substrates using a dip-coating process. The conductive yarns show ohmic behavior with an electrical resistance of 15–31 kΩ cm−1. The conductive polyester yarn exhibits higher mechanical strength even after intensive chemical treatment. It also has the highest gas response of 57% of 50 ppm ammonia gas, the concentration at which health problems will occur. A linear gas response of the yarn sensor appears in a range of 5–25 ppm ammonia concentration. The polyester yarn sensor can be reused without any change in its sensing response. It can monitor gas levels continuously giving real-time results. By using a microcontroller as part of the circuitry, the gas detection results are transferred and updated wirelessly to a computer or to a smartphone. The textile-based gas sensor can be sewn directly onto the fabrics since it is made with the same fabric. This single-yarn-based gas sensor is suitable for mass production and is appropriate for sophisticated applications.
Introduction
There has been an increasing demand for smart electronic devices in recent years. Stretchability and flexibility are two essential needs in the next-generation of wearable electronic devices.1–3 Electronic textiles, a remarkable improvement on lightweight and low cost manufacturing of electronic devices will enable the development of smart wearable devices which can be used for energy harvesting,2 energy storage devices3–5 and biomedical sensors.6 A textile-based gas sensor integrated into with electronic circuits will have a high potential for use in smart clothing which can monitor the environment and can improve personal safety protection. The concentrations of toxic and corrosive gases are the things to monitor in industrial and working spaces. Therefore, the integration of gas monitoring sensors into clothing and other wearable fabrics are necessary. This would be useful to the first responders and to workers who deal with toxic gas. It would also make them convenient to use onsite.
There have been some investigations on high performance textile-based gas sensors, for example, nanoscale carbon materials7–9 and conducting polymers.10–14 The conductive polymer is interesting due to its being a highly stretchable conductive material, so it has been used in various applications.10,15–18 However, making a polymer-based sensor is complicated because of stringent electrical and mechanical constraints as well as the need to simultaneously detect different oxidizing and reducing gases. Some examples of conductive polymer are polypyrrole,14 polythiophene,19 poly(3,4-ethylenedioxythiophene) polystyrene sulfonate,20,21 poly(3-hexylthiophene)22 and polyaniline (PANi).10–12 PANi is one of most interesting sensing materials due to its ease of synthesis. It is also stable and has tunable electrical property obtained by using different redox process.11,13,23 The gas sensing mechanism relies on the presence of π–π conjugated electrons in conducting polymer chains.14 The interaction of gas molecules with PANi will change carrier densities in the polymer.23 Its electrical conductivity will change depending on the type of gas molecules being monitored.24 There are many studies on the conductivity of PANi on substrates obtained by various techniques such as the polymerization process,11 electrospun method,12 dip coating24 or printing techniques.25 PANi is one of the polymer which can detect ammonia (NH3) gas, one of a toxic gas. The detectable concentration level of NH3 is another aspect that needs to be consider. NH3 is a colorless and pungent odor that humans can detect unaided at concentration greater than 5 ppm. Concentrations of NH3 above 50 ppm can cause health problems.26 The recommended exposure limit (REL) for NH3 set by the National Institute for Occupational Safety and Health Administration (NIOSH) is 25 ppm for an 8 hour time-weighted average (TWA), while the short-term exposure limit (STEL) of 35 ppm during any 15 minute period in a work day.27–30 There are some studies that reported the used of PANi as sensing material for textile-based NH3 gas sensor.31,32 The polymerization method was often used for PANi fabrication on textile31,32 or flexible substrate.33 The process was done with many parameters controlling. However, to avoid complicated conditions, coating process is a considerable method since it is easy, convenient and has high possibility to be commercialized.
In this research, we constructed a new type of single-yarn-based gas sensor that can be sewable and be reused as a low concentration NH3 detection. The material PANi was used as the conductive and sensitive layers incorporated into a single yarn substrates by a dip coating process. The sensing properties of the sewable yarn sensors were investigated for room temperature operation. We demonstrated that the single yarn gas sensor can act as a variable resistor for adjusting the current through the light emitting diode (LED). This sophisticated applications were accomplished by integrating the gas sensor yarn into a microcontroller system which can communicates what the environment surrounding the clothes. The gas sensor only requires one single yarn in the weave. This makes it convenient for mass production.
Results and discussion
PANi coating process
To fabricate the yarn-based NH3 gas sensor, the coating process for PANi as seen in Fig. 1a was first optimized. The concentration of PANi was varied from 1.0, 1.5, 2.0 and 2.5 wt% as show in Fig. 1b. After doping with hydrochloric acid (HCl), the electrical resistance of the conductive cotton and rayon yarns decreased significantly with increasing concentrations of PANi solution. At concentrations of 1.0 and 1.5 wt%, the average electrical resistances of cotton and rayon were high with large variation over different parts of the yarn. This indicates that the PANi molecules were not well distributed over the yarns' surface, mainly due to the low concentration of PANi in the coating solution. By increasing the PANi concentration to 2.0 wt%, the average resistance as well as the variation was considerably reduced. Particularly, the PANi-coated cotton and rayon exhibited average resistance values of 19.4 ± 8.9 kΩ cm−1 and 45.2 ± 21.6 kΩ cm−1, respectively (Fig. S1†). This indicates that PANi molecules in this case are well distributed on the yarn surface. Increasing the concentration to 2.5 wt% only had slight influence on the resistance and variation. Therefore, further experiments were carried out for the yarn with 2.0 wt% PANi concentration. In the case of polyester yarn, the resistance could not be measured even after the PANi was coated. This was attributed to the surface nature of polyester yarn which had no hydroxyl (–OH) groups. The cotton and rayon yarns, on the other hand, have a considerable number of –OH groups on their surfaces, which allow the adsorption of PANi molecules on the surface.
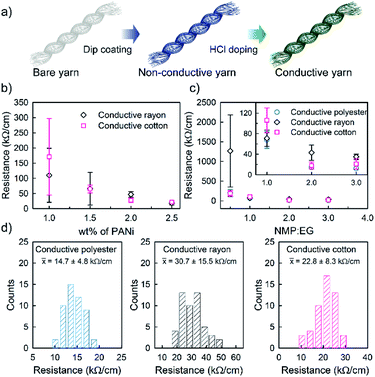 |
| Fig. 1 (a) Fabrication process of the conductive yarn. (b) Electrical resistance investigation by increasing weight percent of PANi. (c) Electrical resistance investigation by increasing ratio of NMP : EG on polyester, cotton and rayon yarns. (d) Histograms of electrical resistance of conductive polyester, cotton and rayon yarns with adding EG. | |
To promote the adhesion of PANi molecules on the polyester yarn surface, we added ethylene glycol (EG) to the coating solution as a adhesion promoter. Since the EG molecule contains the –OH groups, it can induce the bonding between PANi molecule and the polyester yarn.34 To optimize the EG concentration, the ratio of 1-methyl-2-pyrrolidinone to EG (NMP
:
EG) was varied as 1
:
2, 1
:
1, 2
:
1 and 3
:
1 by volume. Fig. 1c shows the electrical resistance of PANi-coated polyester yarns fabricated with different NMP
:
EG ratios. At first, it can be seen that by adding EG, the conductive polyester yarns could be obtained. This indicated that PANi was successfully coated onto the polyester yarn. Next, as the volume ratio of EG was decreased from 1
:
2 to 1
:
1 and 2
:
1, the electrical resistance decreased from 197.9 ± 90.7 kΩ cm−1 to 67.8 ± 16.4 kΩ cm−1 and 14.7 ± 4.8 kΩ cm−1, respectively. Changing the NMP
:
EG ratio to 3
:
1 had a negligible effect on the resistance. We therefore set the optimum ratio of NMP
:
EG to be 2
:
1, in regards to the conductivity. Fig. 1d, left panel shows the electrical resistance distribution of the fabricated conductive polyester yarns (NMP
:
EG ratio = 2
:
1) measured at 50 points. This confirms that the yarns were of reasonable uniformity. In a similar manner, the conductive cotton and rayon yarns were fabricated, by using coating solutions with NMP
:
EG ratio of 2
:
1. Fig. 1d, middle and right panels show the electrical resistance distributions of conductive cotton and rayon yarns, respectively. Compared to those fabricated without the EG, the resistance values are only slightly different.
The surface morphologies of PANi-coated conducive yarns are analyzed. Fig. 2 shows the scanning electron microscope (SEM) images of polyester, rayon and cotton yarns with different coating conditions. In the case of rayon and cotton yarns, the bare yarns show smooth surface while the PANi-coated yarns, either with or without using EG, exhibit rough and irregular morphologies. These are indicative of the PANi molecule adsorption on the surface. In the case of polyester yarn, however, no significant change in the surface was observed when the PANi was coated without using EG. By adding EG, on the other hand, uniform and dense layer of PANi was coated on the surface. Additional SEM images can be seen in Fig. S2.†
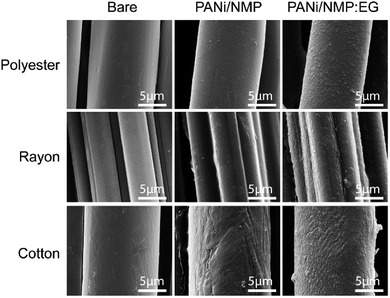 |
| Fig. 2 Comparison of SEM images between bare yarns and coating PANi with/without EG on polyester, rayon and cotton yarns. | |
To further identify the existence of PANi layer on yarn surfaces, Fourier-transform infrared spectroscopy (FTIR) with an attenuated total reflection (ATR) was done as shown in Fig. 3. The cotton and rayon show almost the same peaks on both the bare yarns and coated yarns. While the polyester shows different peaks since it has a different structure. The carbonyl (C
O) stretching band at 1714 cm−1 in the FTIR spectra identify it as polyester.35 For PANi molecules, the peaks at 1593 cm−1 and 1498 cm−1 indicate the presence of quinoid and benzenoid ring-stretching, respectively.36,37 In ESI Table S1† also shows the functional group relating to its wavenumber (cm−1). This clearly reveals and supports the absorption of the PANi molecules on the yarns' surface.
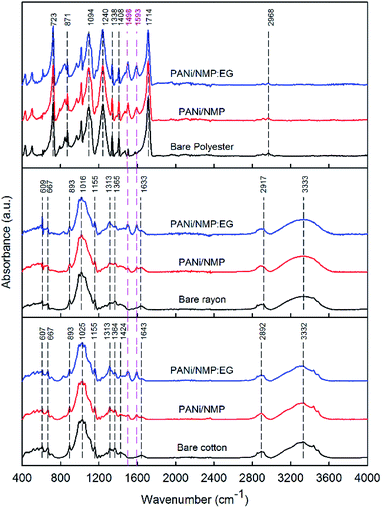 |
| Fig. 3 Comparison FTIR spectra of bare yarns and PANi coating with/without EG on polyester, rayon and cotton yarns. | |
Mechanical properties of PANi-coated conductive yarns
The influence of the PANi coating on the mechanical properties of conductive yarns are shown in Fig. S3.† The maximum strain (εmax) of the bare cotton yarn was 15.5% and is reduced to 13.1% after coating the PANi layer (before HCl doping). After HCl doping, the εmax is further reduced to 8.3%. Similar behavior was observed for the rayon yarn. In this case, the bare yarn shows an εmax of 15.2% which is reduced to 14.9% and 8.4% after PANi coating and HCl doping, respectively. The significant reduction of mechanical strength and of the breaking load observed in cotton and rayon yarns can be attributed to the HCl, which can break the structure of cellulose.38 In the case of polyester yarn, however, opposite behavior is observed. Here, the εmax was increased from 15.3% to 19.2% after the PANi coating. Even after the HCl doping, the εmax is still higher than that of the bare yarn.
Gas sensing properties of PANi-coated conductive yarns
Using the fabricated conductive yarns, textile-based gas sensors were fabricated as shown in Fig. 4a. The PANi-coated conductive yarn was used as a resistance load connection between the buttons on cloth, one side of the button is attached to a LED and the other side attached to a battery. Linear and almost symmetrical current–voltage (I–V) characteristics of the gas sensor units indicate that ohmic contacts are formed between the conductive yarn and the metal electrodes (Fig. 4b). The sensing response (S) was calculated from the gas response curve by using S = (Rg − R0)/R0, where, R0 and Rg are sensing resistance before and after exposure to a target gas. Since the conductive polyester yarn exhibited better mechanical properties than the other conductive yarns, it was first tested to examine the sensitivity to different toxic gases such as ammonia (NH3), nitrogen dioxide (NO2), carbon monoxide (CO), hydrogen disulfide (H2S) and sulfur dioxide (SO2). Fig. 4c and S4a† show the dynamic sensing response on each toxic gas at concentrations of 50 ppm. It appears that the sensor exhibited the highest sensitivity to NH3 gas among the toxic gases tested. Its sensitivity was comparable to the volatile organic compounds such as ethanol, methanol, acetone and toluene (at concentrations of 200 ppm), Fig. S4b.† The sensitivity to NH3 gas was comparably higher (Fig. 4d). These results show that the PANi-coated polyester yarn has a selectivity to NH3 gas and it would be a good candidate for a sensing element for NH3.
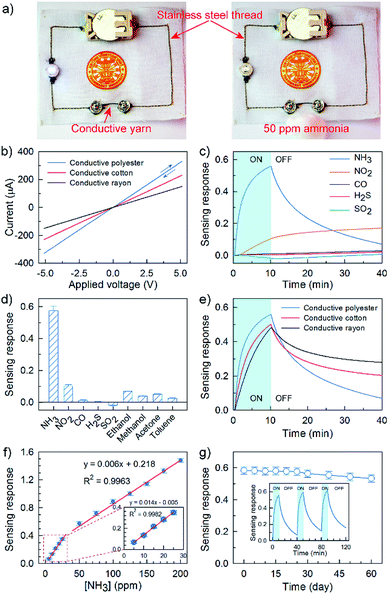 |
| Fig. 4 (a) Testing circuit images of gas sensor yarns. (b) Current–voltage (I–V) characteristic of textile sensors on different yarn substrate. (c) Sensing response of conductive polyester yarn sensor on different toxic gases exposure at 50 ppm. (d) Selectivity to different toxic gases exposure at 50 ppm and different volatile organic compounds exposure at 200 ppm. (e) Sensing response comparison of different yarn substrates on 50 ppm NH3 gas. (f) Gas sensing response from 5 ppm to 200 ppm of ammonia gas concentrations on conductive polyester yarn sensor (inset: the linear sensing responses were shown at 5 ppm to 25 ppm). (g) Stability of sensing response (blue circle) measuring within 60 days of conductive polyester yarn sensor (inset: dynamic response under repeated 50 ppm NH3 gas exposure). | |
In Fig. 4e, the gas response characteristics of three different conductive yarns, polyester, cotton and rayon, are compared, when exposed to 50 ppm of NH3 gas. The conductive polyester yarn exhibited the highest response up to 57%, while the conductive cotton and rayon yarns showed a bit lower response of 50% and 48%, respectively. The low responses for cotton and rayon can be attributed to the low stability of conductive cotton and rayon yarns under dark and dry air (30% RH, 25 °C, Fig. S5†), leading to a high base-resistance and a low sensing response under gas exposure. In addition, compared to cotton and rayon yarns, the polyester yarn showed much faster recovery. It is expected that the cellulose structure in cotton and rayon yarns can adsorb the NH3 molecules more closely,39 thereby slightly delaying the recovery process after the NH3 gas exposure. The effect of humidity to NH3 gas sensing response was also tested and shown in Fig. S6.† The results indicate that the humidity has a negligible effect on the gas sensing properties.
To investigate the concentration-dependent sensing properties of polyester yarn sensors, the sensing response at different NH3 gas concentrations was measured in the range of 5–200 ppm (Fig. 4f). In the range of 5–25 ppm, the polyester yarns sensor exhibited a good linearity against the NH3 concentration (R2 = 0.9982) and gas sensitivity of 0.014 ppm−1. From the extrapolation of the line, the minimum detection limit was 0.4 ppm. Above 50 ppm, the sensitivity was slightly reduced (0.006 ppm−1) which can be attributed to a high surface coverage of NH3 molecules attached on the PANi. Considering the human's detection level for NH3 gas is above 5 ppm and the recommended exposure limit (25 ppm),26,28 the polyester yarn sensor would be a good candidate for real-time monitoring of NH3 gas.
For the practical application of polyester yarn-based gas sensors, the long-term operational stability was also examined over a period of 60 days. As shown in Fig. 4g, the sensor responses were measured for every 5 days. The sensing response showed relatively stable characteristics and only a slight decrease in the sensing response was observed (8.6% after 60 days). In addition, a repeated dynamic response of polyester yarn sensor was also investigated by exposing the NH3 gas (50 ppm) at an interval of 30 min. The NH3 gas was tuned on for 10 min. As shown in the inset of Fig. 4g, the sensor showed reasonably good and reproducible response behavior to the repeated NH3 gas exposure.
Concerning the NH3 gas sensing mechanism using PANi, a schematic illustration is shown in Fig. S7.† The sensing mechanism can describe based on a change of polaron density inside the band gap of polymer.13 In particular, polyaniline emeraldine base (PANi-EB) contains nitrogen (N) atoms attach to quinoid rings, and these N atoms can interact with HCl which is an oxidizing agent. Once the nitrogen atoms get protonated from HCl, as show in eqn (1), yielding conducive form known as the polyaniline emeraldine salt (PANi-ES). The free positive charge was created and can move along the polymer backbone. The coupling between mobile carriers and polarons on the PANi backbone are responsible for conductivity in PANi-ES.13 PANi-ES is a p-type semiconductor and under the conduction state, after the appearance of PANi-H+ in the backbone, it can detect NH3 gas which is well-known as a reducing gas. Upon expose to NH3 gas, electrons are transferred from NH3 to PANi-H+, which then reduce hole-carrier concentration in the PANi-ES resulting in higher in resistance of the backbone. In addition, this creates lower amount of polarons and thus increase the electrical resistance.25 Also, after the NH3 molecule losses the electron, it behaves as NH4+ ion attach to the backbone. The electron donation and reversible process of PANi-ES and NH3 gas can occur due to the redox reaction23 as seen in eqn (2). When the air flows to the system, the amount of the NH3 is gradually diluted yielding a lower amount of donated electrons to PANi-H+. This makes the hole carrier concentration also gradually increased back to what it was before and resulting in the original value of PANi-ES conductivity. This corresponds to results shown in Fig. 4e and the inset of Fig. 4g.
|
PANi + HCl → PANi-H+ + Cl−
| (1) |
|
PANi-H+ + NH3 ⇌ PANi + NH+4
| (2) |
We also investigated the effect of bending angle on the gas sensing properties. Here, the bending angle was changed from 0° to 45°, 90° and 135°, and the sensing responses were observed. As shown in Fig. S8,† no significant variation was observed even at an angle of 135°, suggesting that the fabricated gas sensors are mechanically flexible and stable.
Real-time monitoring of NH3 gas using polyester yarn sensors
In order to demonstrate a possible application for textile-based gas sensor, an NH3 gas sensing unit was fabricated on a fabric using the PANi-coated polyester yarn. As shown in Fig. 5a, the polyester yarn sensor was sewn on the fabric in a triangular pattern (a total length of 1.5 cm). Stainless steel threads were used as electrical contacts on both ends of the yarn. For the gas sensing unit, three-trace level of NH3 gas was designed using a simple comparator circuit, which indicates the exposure level by LEDs. As shown in Fig. 5b, under clean atmosphere, no LEDs were turned on. However, when the NH3 gas concentration becomes higher than 5 ppm, one LED is turned on (Fig. 5c). When the NH3 gas concentration is higher than 25 ppm and 50 ppm, two and three LEDs turn on as seen in Fig. 5d and e, respectively. These results clearly show that the fabricated PANi-coated polyester yarn can be utilized in real-time monitoring of NH3 gas, indicating the exposure levels to the users. By integrating the polyester yarn sensor with microcontroller systems, an active gas sensor for wireless applications can be realized. In Fig. S9a,† we showed a wireless wearable NH3 gas monitoring system, in which the polyester yarn sensor was sewn on the upper arm of a shirt. The sensor was connected to a microcontroller system integrated with a wireless transmitter and receiver circuit. As illustrated in Fig. S9b,† when the NH3 gas in the environment reaches a limit, the system sends an alarm signal to the user. The wireless gas sensing system can also be used to monitor the gas level continuously over a given period of time. Here, when the sensor is exposed to a certain level of NH3 gas, the resistance of the sensor changes which results in a voltage drop (Fig. S9c†). As the exposed gas level is increased, the voltage drop will become greater.
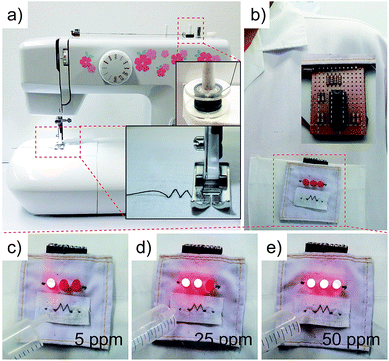 |
| Fig. 5 Photograph images of conductive polyester yarn sensor. (a) Sewing conductive polyester yarn on cloth by using a sewing machine. (b) Polyester yarn sensor attached on a pocket of laboratory coat. (c–e) Gas sensor demonstration with different concentration of ammonia gas at 5 ppm, 25 ppm and 50 ppm by turning on LEDs. | |
The performance of our yarn sensor is compared with other works in Table 1. It can be noted that the resistance value of a yarn sensor is an important factor to determine the cost effectiveness of the sensor circuit. Due to low based resistance is necessary for a simple amplifier circuit and low level input impedance of measurement devices. Our polyester sensor had a resistance value of 0.015 MΩ cm−1 which is the lowest compare to other PANi-based yarn sensors and it is only 2 times larger than that of SWCNT-on-cotton which had a value of 0.008 MΩ cm−1. There are also a few reports show stability testing on yarn sensors because it is another importance factor to identify durability in real use. Our yarn sensor showed a stability up to 60 days which is relatively good compared to other PANi-based NH3 sensors. Our intension to fabricate low cost sewable gas sensor for commercialize make us conduct sensor response after pulling our yarn sensor with a commercial sewing machine. The pulling force from the sewing machine has no effect to sensing response since the results of our yarn sensor confirm its higher value of strain/force after treatment with chemicals. The other importance parameter is area detection, this is essential for making array gas detector. Our sensor can perform under small area compare to the other yarn sensors.
Table 1 Comparison of the performance of the yarn sensorsa
Materials |
Substrates |
Gas |
R0 (MΩ) |
Strain (%)/force (N) |
Stability (days) |
Detection area (mm2) |
Ref. |
MWCNT is multiwall carbon nanotube; SWCNT is single wall carbon nanotube; PPy is polypyrrole; RGO is reduced graphene oxide; PAN is polyacrylonitrile; NW-PP is non-woven polypropylene. |
PANi |
Yarn/PS |
NH3 |
0.015/cm |
17/10 |
60 |
9.42 |
This work |
PANi |
Yarn/PAN |
NH3 |
0.2/cm |
5/0.51 |
30 |
5.65 |
31 |
PANi |
Fabric/NW-PP |
NH3 |
5.55/cm2 |
— |
— |
600 |
40 |
PANi/MWCNT |
Fabric/— |
NH3 |
2.28/cm2 |
— |
30 |
100 |
32 |
SWCNT |
Yarn/CT |
NH3 |
0.008/cm |
14/— |
— |
— |
9 |
PPy |
Fabric/CT |
NH3 |
— |
— |
— |
— |
41 |
RGO |
Yarn/CT |
NO2 |
— |
— |
7 |
28.27–47.12 |
8 |
MoS2/RGO |
Yarn/CT |
NO2 |
— |
— |
— |
21.99–43.98 |
7 |
Experimental section
Materials
Polyaniline emeraldine base (PANi-EB, Mw ∼ 20
000), 1-methyl-2-pyrrolidinone 99% (NMP) and ethylene glycol (EG) 99.8% were purchased from Sigma Aldrich. Hydrochloric acid 37% (HCl), acetone and all solvents were purchased from RCI Labscan. All toxic gases, inert gases and synthetic air were purchased from Linde. All chemicals were used as received.
Fabrication of conductive yarns
The conductive yarns were fabricated on single yarn substrates in ambient environment by dip coating process. In brief, the precursor solution of conductive and sensitive layers was prepared by dissolving PANi-EB (32.3 mg, ∼1.6 μmol) in 1-methyl-2-pyrrolidinone (1 mL, 10 mmol) yielding a concentration of 2 wt%. The PANi-EB/NMP solution was stirred for 72 hours at room temperature. To enhance the adhesion of PANi on the yarn surfaces, ethylene glycol (0.5 mL, 8.97 mmol) was slowly dropped into the PANi-EB/NMP solution using a mixing ratio of 2
:
1 (NMP
:
EG). A single polyester yarn substrate was sonicated using acetone and dried in oven at 60 °C for 30 minutes to remove any surface residues. After that, the cleaned yarn was immersed into the PANi-EB/NMP
:
EG solution for 10 minutes and dried at 60 °C for 2 hours. Finally, the PANi-coated yarn was doped with 5 M HCl for 30 minutes to induce a conductive form of polyaniline emeraldine salt (PANi-ES). Identical process was also carried out for rayon and cotton yarns (Fig. 1a).
Fabrication of textile-based gas sensors and circuits
For application of all textile-based gas sensor, the PANi-coated yarn was sewn onto the fabric by using a sewing machine. Conducting stainless-steel threads were used as the electrode connector for measurement purposes. For fabrication of real-time gas monitoring devices, the sensing signal was wired to an input signal of three-level comparator circuits (LM324 operation amplifier) which was connected to a small rechargeable battery 3.7 V. LED was used as the alarm devices. For wireless gas monitoring, a microcontroller (NodeMCU Devkit V1.0) was used instead.
Characterization of gas sensors
The morphologies of yarn-based gas sensors were observed by using a scanning electron microscope (SEM, FEI model Quanta 450). The deposition of PANi on yarn surfaces was verified by Fourier-transform infrared spectroscopy (FTIR, Bruker model Vertex 70) with an attenuated total reflection (ATR) setup. The electrical characteristics of the sensors were measured by Keithley 6517B electrometer and Keithley 2400 source meter. The gas sensing characteristics were analyzed using a homebuilt gas measurement system. Briefly, the conductive yarn sensor was placed in a closed chamber and a constant voltage of 5 V was supplied to the sensor. Desired concentration of NH3 gas was obtained by diluting 500 ppm standard gas with synthetic air before feeding into the measurement chamber. The total gas flow rate was set at 150 sccm by programmable mass flow controller. In addition, the operating temperature and humidity inside the chamber were measured using a commercial sensor (SHT15, Sensirion AG). The tensile strength of the yarns was investigated using an automatic tensile tester. Here, the load cell was 50 N and the sample length was 10 cm with a pulling speed of 10 mm min−1.
Conclusions
In summary, a single-yarn-based gas sensor with a highly mechanical and sewable textile sensor as well as clothes-based sensing system has been shown for the first time. A chemiresistive type of textile-based gas sensor was fabricated by coating PANi on the surface of common yarns such as cotton, rayon and polyester. EG was used as a mediator to help PANi attached to the yarns surface. This is possible due to the existence of –OH group in the EG. By doping PANi with HCl, the conductive yarns were achieved. The results show that the conductive polyester yarn exhibits a higher sensing response with a good mechanical strength when compared to those of conductive cotton and conductive rayon yarns. The conductive polyester yarn also exhibits the highest response to NH3 gas compared the response to other toxic gases. NH3 gas can be measured to as low as 5 ppm and up to more than 200 ppm under using the same conductive yarn. This means that the gas can be sensed repeatedly and this textile-based gas sensor is reusable. More advantage of this sensor, its light weight and its sewable into a fabric using a sewing machine. The results show that the conductive polyester yarn-based gas sensor has the advantage of being able to be incorporate into smart gas monitoring shirts use.
Conflicts of interest
There are no conflicts to declare.
Acknowledgements
This work was partially supported by Thammasat University through the National Research Center of Thailand (Contract No. 17/2559 and 16/2560), Thammasat University innovation scholar (Contract No. 2/2559) and Faculty of Science and Technology (Contract No. 11/2559). N. P. thanks Kasetsart University Research and Development Institute (KURDI) and the Faculty of Science, Kasetsart University. We thank the Scientific Equipment Center and Department of Materials Science at Faculty of Science, Kasetsart University for using their SEM and FTIR equipment.
Notes and references
- D. J. Lipomi, B. C. K. Tee, M. Vosgueritchian and Z. Bao, Adv. Mater., 2011, 23, 1771 CrossRef CAS PubMed.
- Y. M. Choi, M. G. Lee and Y. Jeon, Energies, 2017, 10, 1 Search PubMed.
- C. Li, M. Islam, J. Moore, J. Sleppy, C. Morrison, K. Konstantinov, S. X. Dou, C. Renduchintala and J. Thomas, Nat. Commun., 2016, 7, 1 Search PubMed.
- Z. Chai, N. Zhang, P. Sun, Y. Huang, C. Zhao, H. J. Fan, X. Fan and W. Mai, ACS Nano, 2016, 10, 9201 CrossRef CAS PubMed.
- A. E. Ostfeld, A. M. Gaikwad, Y. Khan and A. C. Arias, Sci. Rep., 2016, 6, 1 CrossRef PubMed.
- J. C. Y. Kenry and C. T. Lim, Microsyst. Nanoeng., 2016, 2, 1 Search PubMed.
- Y. J. Yun, W. G. Hong, D. Y. Kim, H. J. Kim, Y. Jun and H. K. Lee, Sens. Actuators, B, 2017, 248, 829 CrossRef CAS.
- Y. J. Yun, W. G. Hong, N. J. Choi, B. H. Kim, Y. Jun and H. K. Lee, Sci. Rep., 2015, 5, 1 CrossRef.
- J. W. Han, B. Kim, J. Li and M. Meyyappan, Appl. Phys. Lett., 2013, 102, 1 Search PubMed.
- Z. Ma, P. Chen, W. Cheng, K. Yan, L. Pan, Y. Shi and G. Yu, Nano Lett., 2018, 18, 4570 CrossRef CAS PubMed.
- S. Chen and G. Sun, ACS Appl. Mater. Interfaces, 2013, 5, 6473 CrossRef CAS PubMed.
- Y. Zhang, J. J. Kim, D. Chen, H. L. Tuller and G. C. Rutledge, Adv. Funct. Mater., 2014, 24, 4005 CrossRef CAS.
- S. Virji, J. Huang, R. B. Kaner and B. H. Weiller, Nano Lett., 2004, 4, 491 CrossRef CAS.
- H. Bai and G. Shi, Sensors, 2007, 7, 267 CrossRef CAS.
- J. Janata and M. Josowicz, Nat. Mater., 2003, 2, 19 CrossRef CAS PubMed.
- M. E. Abdelhamid, A. P. O'Mullane and G. A. Snook, RSC Adv., 2015, 5, 11611 RSC.
- J. G. Ibanez, M. E. Rincón, S. Gutierrez-Granados, M. Chahma, O. A. Jaramillo-Quintero and B. A. Frontana-Uribe, Chem. Rev., 2018, 118, 4731 CrossRef CAS PubMed.
- M. Naseri, L. Fotouhi and A. Ehsani, Chem. Rec., 2018, 18, 599 CrossRef CAS PubMed.
- J. B. Chang, V. Liu, V. Subramanian, K. Sivula, C. Luscombe, A. Murphy, J. Liu and J. M. J. Fréchet, J. Appl. Phys., 2006, 100, 1 CrossRef PubMed.
- M. Hakimi, A. Salehi and F. A. Boroumand, IEEE Sens. J., 2016, 16, 6149 CAS.
- J. Eom, R. Jaisutti, H. Lee, W. Lee, J. Heo, J. Lee, S. K. Park and Y. Kim, ACS Appl. Mater. Interfaces, 2017, 9, 10190 CrossRef CAS PubMed.
- M. R. Cavallari, J. E. E. Izquierdo, G. S. Braga, E. A. T. Dirani, M. A. Pereira-da-Silva, E. F. G. Rodríguez and F. J. Fonseca, Sensors, 2015, 15, 9592 CrossRef CAS PubMed.
- H. Ullah, A. A. Shah, S. Bilal and K. Ayub, J. Phys. Chem. C, 2013, 117, 23701 CrossRef CAS.
- D. Nicolas-debarnot and F. Poncin-epaillard, Anal. Chim. Acta, 2003, 475, 1 CrossRef CAS.
- K. Crowley, A. Morrin, A. Hernandez, E. O. Malley, P. G. Whitten, G. G. Wallace, M. R. Smyth and A. J. Killard, Talanta, 2008, 77, 710 CrossRef CAS.
- NIOSH (National Institute for Occupational Safety and Health), Occupational safety and health guidelines for chemical hazards. Supplement III-OHG Revised Guideline for Ammonia, 1992 Search PubMed.
- M. J. Fedoruk and B. D. Kerger, J. Exposure Anal. Environ. Epidemiol., 2005, 15, 534 CrossRef CAS PubMed.
- New Jersey Department of Health, Hazardous substance fact sheet: Ammonia (RTK substance No. 0084) Revision, Right to Know, 2016 Search PubMed.
- U.S. Department of Health and Human Services, Public Health Registry and Agency for Toxic Substances and Disease Registry, Toxicological Profile for Ammonia, 2004 Search PubMed.
- Integrated Risk Information System, National Center for Environmental Assessment, Office of Research and Development and U.S. Environmental Protection Agency, Toxicological Review of Ammonia Noncancer. Inhalation: Executive Summary [CASRN 7664-41-7], Washington, 2016 Search PubMed.
- S. Wu, P. Liu, Y. Zhang, H. Zhang and X. Qin, Sens. Actuators, B, 2017, 252, 697 CrossRef CAS.
- D. Maity and R. T. R. Kumar, ACS Sens., 2018, 3, 1822 CrossRef CAS PubMed.
- J. Cai, C. Zhang, A. Khan, C. Liang and W. Li, RSC Adv., 2018, 8, 5312 RSC.
- W. Wardencki and A. H. H. Tameesh, J. Chem. Technol. Biotechnol., 1981, 31, 86 CrossRef CAS.
- P. Peets, I. Leito, J. Pelt and S. Vahur, Spectrochim. Acta, Part A, 2017, 173, 175 CrossRef CAS PubMed.
- E. N. Konyushenko, S. Reynaud, V. Pellerin, M. Trchová, J. Stejskal and I. Sapurina, Polymer, 2011, 52, 1900 CrossRef CAS.
- M. Trchová, I. Šeděnková, E. Tobolková and J. Stejskal, Polym. Degrad. Stab., 2004, 86, 179 CrossRef.
- J. H. Lin, Y. H. Chang and Y. H. Hsu, Food Hydrocolloids, 2009, 23, 1548 CrossRef CAS.
- M. Wada, Y. Nishiyama and P. Langan, Macromolecules, 2006, 39, 2947 CrossRef CAS.
- J. Qi, X. Xinxin, X. Liu and K. T. Lau, Sens. Actuators, B, 2014, 202, 732 CrossRef CAS.
- S. C. Deogaonkar and N. V. Bhat, Fibers Polym., 2015, 16, 1803 CrossRef CAS.
Footnote |
† Electronic supplementary information (ESI) available. See DOI: 10.1039/c9ra04005f |
|
This journal is © The Royal Society of Chemistry 2019 |
Click here to see how this site uses Cookies. View our privacy policy here.