DOI:
10.1039/C9RA03956B
(Paper)
RSC Adv., 2019,
9, 32232-32239
The effects of Bi2O3 on the selective catalytic reduction of NO by propylene over Co3O4 nanoplates
Received
25th May 2019
, Accepted 27th September 2019
First published on 10th October 2019
Abstract
Bi2O3/Co3O4 catalysts prepared by the impregnation method were investigated for the selective catalytic reduction of NO by C3H6 (C3H6-SCR) in the presence of O2. Their physicochemical properties were analyzed with SEM, XRD, H2-TPR, XPS, PL and IR measurements. It was found that the deposition of Bi2O3 on Co3O4 nanoplates enhanced the catalytic activity, especially at low reaction temperature. The SO2 tolerance of Co3O4 in C3H6-SCR activity was also improved with the addition of Bi2O3. Among all catalysts tested, 10.0 wt% Bi2O3/Co3O4 achieved a 90% NO conversion at 200 °C with the total flow rate of 200 mL min−1 (GHSV 30
000 h−1). No loss in its C3H6-SCR activity was observed at different temperatures after the addition of 100 ppm of SO2 to the reaction mixture. These enhanced catalytic behaviors may be associated with the improved oxidizing characteristics of 10.0 wt% Bi2O3/Co3O2. XRD results showed that Bi2O3 entered the lattice of Co3O4, resulting in the formation of lattice distortion and structural defects. H2-TPR results showed that the reduction of Co3O4 was promoted and the diffusion of oxygen was accelerated with the addition of Bi2O3. XPS measurements implied that more Co3+ formed on the 10.0% Bi2O3/Co3O2 catalysts. The improved oxidizing characteristics of the catalyst with the addition of Bi2O3 due to the synergistic effect of the nanostructure hybrid, thus enhanced the C3H6-SCR reaction and hindered the oxidization of SO2. Therefore, the 10.0% Bi2O3/Co3O4 catalyst exhibited the highest NO conversion and strongest SO2 tolerance ability.
1 Introduction
Lean burn engines, which are generally used in gasoline and diesel powered vehicles, are more fuel-efficient than the stoichiometric gasoline engines.1 They also effectively reduce unburned hydrocarbons, CO2 and CO in exhausts.2 However, lean burn engines operate with a large excess of air, leading to a significant concentration of oxygen in the exhausts, where the noble-metal three-way catalysts cannot work well to reduce nitrogen oxides (NOx).3 A large amount of NOx produced by lean burn engines leads to serious air pollution and public health problems.
In order to control NOx emission under the lean burn conditions, selective catalytic reduction of NO by hydrocarbons (e.g. propylene) has been undertaken and reported in the literatures as one potential application (HC-SCR). Many classes of catalysts, including supported noble metals (e.g. Pt,4,5 Au6,7), metal oxides (e.g. Ag2O,8,9 CuO,10,11 SnO2,12–14 CoOx (ref. 15–18)) and zeolite types (ZSM-5,19 MCM-41 (ref. 20)) have been investigated. In general, the noble metals are active and stable even at lower temperature, but the formation of N2O is undesirable by using such precious metals, particularly, platinum-based catalysts. The zeolite-based catalysts were low thermal stability. Among metal oxides catalysts, cobalt oxides (e.g. Co3O4) are considered as one promising catalyst for HC-SCR due to its high catalytic activity.21 When combined with other oxides, such as CeO2,16 ZrO2,15 Al2O3,18 sulphated ZrO2,22 the catalytic performances of the cobalt oxide catalyst could be improved as reported. These results implied that the chemical environment around cobalt oxide plays a crucial role in controlling the overall activity of cobalt containing catalysts in SCR reactions.
Bi2O3, a common oxide semiconductor, is widely used in the fields of chemical engineering and electronics such as NO detection23 and the oxidation or ammoxidation of propylene.24,25 In the oxidation/ammoxidation of propene over bismuth/molybdate catalyst, bismuth was thought to involve the rate-determining hydrogen abstraction from propylene,26 exhibiting its mild oxidizing characteristics. This property might be also beneficial for the partial oxidation of propylene in C3H6-SCR for NO reduction. Therefore, it is of considerable interest to explore the application of Bi2O3 in the reduction of NO with propylene.
In the present study, Co3O4 nanoplates and Bi2O3/Co3O4 were prepared with the solvothermal and impregnation method respectively. Their catalytic performances in the NO reduction by C3H6 in the presence of O2 were investigated. The catalysts were characterized with X-ray diffraction (XRD), temperature programmed reduction with hydrogen (H2-TPR), and X-ray photoelectron spectra (XPS). The effects of Bi2O3 on the selective catalytic reduction of NO by propylene over Co3O4 nanoplates were expected to be elucidated.
2 Experimental
2.1 Preparation of Co3O4 and Bi2O3/Co3O4
The Co3O4 support was synthesized via the solvent-thermal method, 50 mmol CoCl2 solution (200 mmol L−1, Sinopharm Chemical Reagent Co. China) and 25 mL of NaOH (2 mol L−1, Sinopharm Chemical Reagent Co. China) were added to a round-bottom flask, ultrasonicated for about 20 minutes to obtain a light brown uniform suspension. And then the suspension was transferred into a stainless steel autoclave with Teflon liner. The autoclave was sealed and maintained at 120 °C for 12 h. The obtained product was collected after washing with deionized water for several times, finally calcined at 500 °C for 5 h in air (S1).
The Bi2O3/Co3O4 catalysts with different Bi2O3 contents were synthesized by impregnation method as followed: 0.0155 g Bi(NO3)3·5H2O and 20 mL 3% NH3·H2O were added into a round-bottom flask, ultrasonicated for about 20 minutes to obtain a white uniform suspension. After that, the Co3O4 support (S1) were added in the suspension, ultrasonicated for about 15 minutes to make it homogeneously distributed in the suspension. The suspension was then dried at 80 °C with continuous stirring for 1 h, further heated at 120 °C for 12 h followed by calcination at 500 °C in air for 4 h, yielding the 5.0 wt% Bi2O3/Co3O4 catalyst (S2). 10.0 wt% Bi2O3/Co3O4 (S3) and 15.0 wt% Bi2O3/Co3O4 (S4) were prepared with 0.0310 g and 0.0465 g Bi(NO3)3·5H2O respectively. In addition, the physical mixture of 10% Bi2O3 nanoparticles and Co3O4 support was also prepared and labeled as S5. As references, classical catalysts 4% Ag/Al2O3 and 2% Pt/Al2O3 were prepared to compare the catalytic performance of the Bi2O3/Co3O4 catalysts.
2.2 Catalytic activity tests
C3H6-SCR over the catalysts was carried out at atmospheric pressure in a fixed-bed quartz reactor (diameter = 10 mm). 0.1 g catalyst was used in each run with a reaction mixture composed of 200 ppm NO, 200 ppm C3H6, 100 ppm SO2 (when needed) and 10 vol% O2 in balance gas N2. The total flow rate was 200 mL min−1, corresponding to a GHSV 30
000 h−1. Reaction temperature ranges from 100 to 500 °C. The concentration of NO was continuously measured by a NO analyzer (Thermo Environmental Instruments Inc., model 42c), which monitors NO, NO2, and NOx (NOx represents NO + NO2). The removal efficiency of NO was calculated as NO removal (%) = (1 − C/C0) × 100%, where C and C0 are concentrations of NO in the outlet and inlet, respectively.
2.3 Catalysts characterization
Scanning electron microscopy (SEM) images were taken on a Hitachi S4800 scanning electron microscope operating at 5.0 kV. X-ray powder diffraction (XRD) was carried out on Brukeraxs D8 Discover (Cu Kα = 1.5406 Å). The scanning rate is 1° min−1 in the 2θ range from 20 to 80 degree. The reducibility of Bi2O3/Co3O4 catalysts was estimated by temperature programmed reduction with hydrogen analysis (H2-TPR). The experiments were carried out with a Micromeritics 2910 apparatus using H2/Ar (3/97, v/v) gas with a total flow rate of 15 mL min−1. In each run, 0.030 g of the catalyst was previously activated at 500 °C for 30 min under air, and then cooled to RT. TPR started with the introduction of the mixture of H2 and Ar. The catalyst was heated from room temperature (RT) to 1000 °C (10 °C min−1). H2 consumption was continuously monitored with the thermal conductivity detector. X-ray photoelectron spectra (XPS) of the catalysts were measured in a VG Multilab 2000 spectrometer by using Al Kα (1486.6 eV) radiation as the X-ray source. Photoluminescence (PL) measurement was carried out on a Shimadzu RF-5301 PC fluorescence spectrophotometer. Raman spectra were recorded using a Horiba Jobin-Yvon Lab Ram HR800 Raman microspectrometer, with an excitation laser at 514 nm.
3 Results
3.1 Scanning electron microscope (SEM) observation and XRD analysis
Co3O4 nanoplates with different dimensions were observed on the support Co3O4 (S1), shown in Fig. 1(a). Fig. 1(b–d) presented Bi2O3/Co3O4 catalysts with different Bi2O3 loading amounts. It was shown that Bi2O3 nanoparticles with dimension ca. 20 nm were supported on Co3O4. And the crystal size of Bi2O3 slightly increased with increasing Bi2O3 loading amount from 5% to 15%.
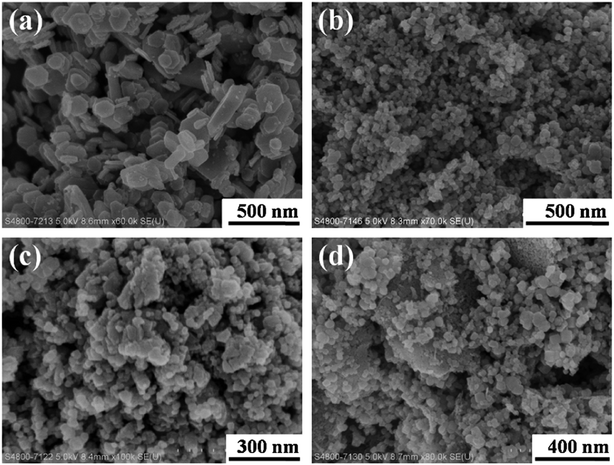 |
| Fig. 1 SEM images of Co3O4 (a), 5.0% Bi2O3/Co3O4 (b), 10.0% Bi2O3/Co3O4 (c) and 15.0% Bi2O3/Co3O4 (d). | |
Fig. 2(A) shows the XRD patterns of the synthesized Co3O4 and Bi2O3/Co3O4 catalysts. It can be seen that the Co3O4 support possessed the characteristic peaks of Co3O4 (JCPDS 73-1701, a = 5.45 Å). Besides the characteristic peaks of Co3O4, the diffraction peaks due to Bi2O3 (JCPDS 22-515, a = 10.94 Å and c = 11.28 Å) were also observed on Bi2O3/Co3O4 catalysts which became sharper with the increase of Bi2O3 loading amount. It was suggested that the crystal size of Bi2O3 was bigger on the catalyst with higher loading amount of Bi2O3, which was consistent with the SEM observations. Moreover, the diffraction peaks of Co3O4 over Bi2O3/Co3O4 catalysts behaved a slight shift towards lower degree in comparison with that of Co3O4, shown in Fig. 2(B). It indicated that the Bi3+ inserted the lattice of Co3O4 in the preparation process, and changed the lattice parameter of Co3O4 due to the different radius of Bi and Co atoms. At the same time, the characteristic peak of Co3O4 became weak obviously after the deposition of Bi2O3, which revealed the reduction of crystal size. It was implied that the insertion of Bi2O3 induced the structure defect of Co3O4 and suppressed the growth of crystal.
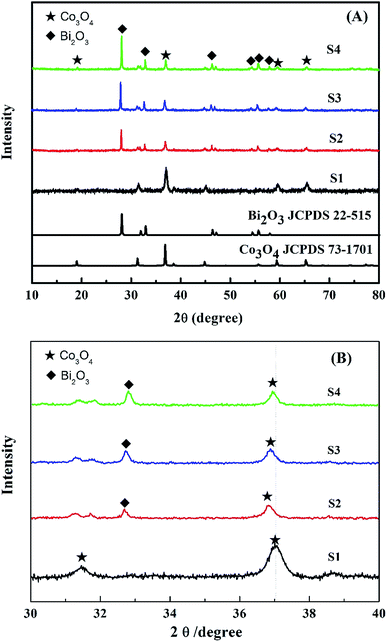 |
| Fig. 2 XRD patterns of Co3O4 (S1), 5.0% Bi2O3/Co3O4 (S2), 10.0% Bi2O3/Co3O4 (S3) and 15.0% Bi2O3/Co3O4 (S4) in the wide (A) and narrow (B) ranges. | |
3.2 Temperature-programmed reduction by hydrogen (H2-TPR)
The H2-TPR profiles of Co3O4, Bi2O3 and Bi2O3/Co3O4 catalysts were shown in Fig. 3. For the reduction of Bi2O3, a sharp peak was observed at ca. 490 °C, implying the reduction of Bi3+ in a narrow temperature range. A broad reduction peak from 330 to 460 °C with a large shoulder at the lower reduction temperature (ca. 380 °C) appeared on Co3O4 support (S1). Many researchers reported that the reduction of Co3O4 was a two-step reduction process involving the intermediate reduction of CoO.16,21,27 Two main clear reduction peaks respectively located around 186 °C and 310–480 °C were shown in the TPR spectra. The low temperature TPR peak was associated with the reduction of Co3+ to Co2+, and the peak at high temperature was the subsequent reduction of CoO to metallic cobalt. In the TPR spectrum of Co3O4 synthesized in the present work, there are no obvious two peaks probably due to an abroad particle size distribution as shown in SEM observation. The large shoulder at 376 °C (peak I) in Fig. 3 should be attributed to the reduction of Co3+ to Co2+, and main reduction peak (peak II) is ascribed to the reduction of CoO to metallic cobalt.
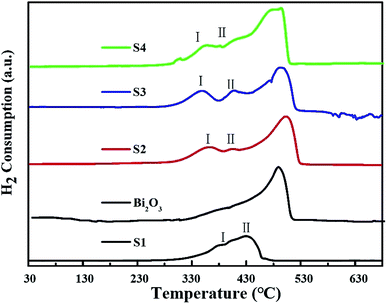 |
| Fig. 3 H2-TPR profiles of pure Co3O4 (S1), Bi2O3, 5.0% Bi2O3/Co3O4 (S2), 10.0% Bi2O3/Co3O4 (S3) and 15.0% Bi2O3/Co3O4 (S4). | |
The reduction process of Bi2O3/Co3O4 catalysts (S2–S4) became complicated with the introduction of Bi2O3. The reduction peak at 487 °C became wider and shifted towards to the lower temperature, especially on the Bi2O3/Co3O4 catalyst with the highest Bi loading amount (S4). In addition, two new peaks around 342 °C (peak I) and 402 °C (peak II) respectively ascribed to the reduction of Co3+ to Co2+, Co2+ to metallic Co appeared on Bi2O3/Co3O4 catalysts (S2–S4).28 Compared with the bulk Co3O4 (S1), both the reduction peak of Co3+ and that of Co2+ shifted to lower temperature after the deposition of Bi2O3, implying the promoted reduction of Co3O4. Moreover, the larger reduction peak I than peak II on S2–S4 samples indicated that the ratio of Co3+/Co2+ was higher on S2–S4 samples than that on the Co3O4 (S1). It revealed that the deposition of Bi2O3 on Co3O4 affected the oxidized state of cobalt in the synthesized Co3O4, more Co3+ were present on the supported samples (S2–S4) than the pure Co3O4.
3.3 X-ray photoelectron spectroscopy (XPS)
XPS measurements were carried out on Co3O4 and 10.0% Bi2O3/Co3O4 catalysts to examine the influence of Bi2O3 on the surface electronic state of Co3O4. The Co 2p and O 1s XPS profiles are shown in Fig. 4. In the Co 2p (Fig. 7(a)), the main peaks located at 779.6–781.3 eV and 794.8–796.5 eV are ascribed to Co 2p1/2 and Co 2p3/2 spin-orbital peaks, respectively.29,30 It was well-known that the spin–orbit splitting value for Co3+ compounds is 15.0 eV, 15.1–15.3 eV for the mixed-valence Co3O4. Here, the spin–orbit splitting values of Co 2p for CO3O4 and 10.0% Bi2O3/Co3O4 are the same, 15.2 eV, which is close to that of mixed-valence Co3O4. So the cobalt species on both S1 and S3 should be Co3O4, agreeable with the XRD and TPR results.
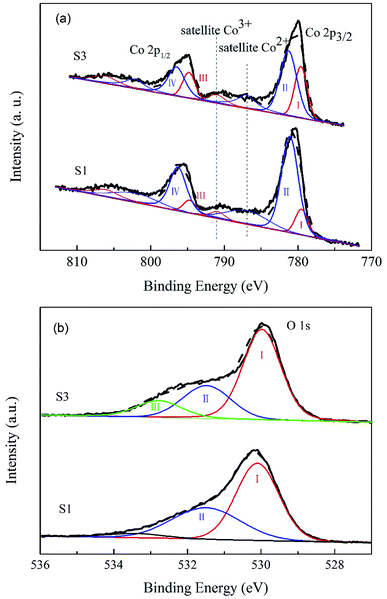 |
| Fig. 4 XPS study of Co3O4 (S1) and 10.0% Bi2O3/Co3O4 (S3): (a) Co 2p spectra, (b) O 1s spectra. | |
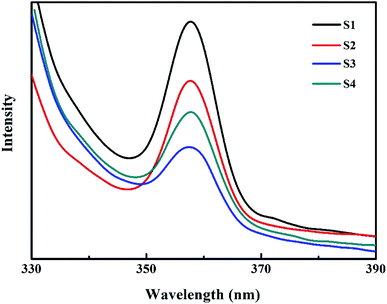 |
| Fig. 5 Room-temperature PL spectra of Co3O4 (S1), 5.0% Bi2O3/Co3O4 (S2), 10.0% Bi2O3/Co3O4 (S3) and 15.0% Bi2O3/Co3O4 (S4). | |
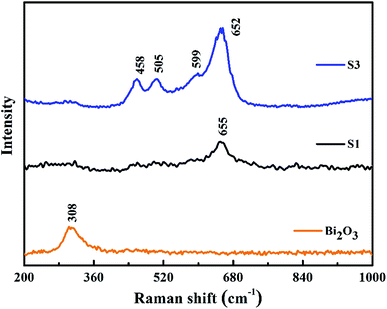 |
| Fig. 6 Raman spectra of Co3O4 (S1) and 10.0% Bi2O3/Co3O4 (S3). | |
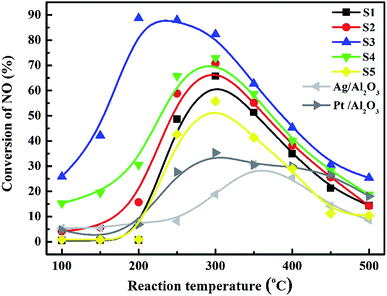 |
| Fig. 7 NO conversions over the catalysts: S1: Co3O4; S2: 5.0% Bi2O3/Co3O4; S3: 10.0% Bi2O3/Co3O4; S4: 15.0% Bi2O3/Co3O4; S5: the physical mixture of Bi2O3 and Co3O4. | |
Based on the restriction that Co 2p3/2 binding energies of Co2+ and Co3+ components are 781.3 eV and 779.6 eV, respectively, the spin–orbit doublet splitting is 15.2 eV with a fixed ratio of 2/1 for the 2p3/2-to-2p1/2 peak area,29 the Co 2p spectra of S1 and S3 can be fitted to the Co2+ (peak II and IV) and Co3+ (peak I and III) components.30,31 The satellite peak of Co2+ and that of Co3+ in Co3O4 were also respectively observed at 787.0 eV and 791.0 eV.32,33Fig. 4 shows that the peak areas of peak I and III increased with the addition of Bi2O3, implying that the surface Co3+ and the surface content ratio of Co3+/Co2+ increased with the addition of Bi2O3. More Co3+ was present on 10.0% Bi2O3/Co3O4 (S3) than the bulk Co3O4 support (S1), as consistent with the TPR results.
The O 1s XPS spectra of Co3O4 and Bi2O3/Co3O4 catalysts are shown in Fig. 4(b). For the Co3O4 sample, there are two peaks (I and II). The peak I located at ∼530.1 eV is attributed to the surface lattice of Co3O4, and the peak II at ∼531.5 eV is associated with OH− groups.34 In the case of 10.0% Bi2O3/Co3O4, besides the peak I and II, one new peak at ∼532.8 eV appeared, which should be related to the contribution of the oxygen from Bi2O3.
3.4 Photoluminescence (PL) and Raman spectra
PL emission spectra originating from the recombination of free charge carriers are useful to reveal the migration, transfer and separation of photogenerated charge carriers. Fig. 5 shows photoluminescence emission spectra of different catalysts at room temperature. All samples show one luminescence peak center at about 358 nm, which can be attributed to the radiative recombination of charge carriers. The pure Co3O4 has the strongest PL emission peak. This charge recombination process of Co3O4 can be greatly inhibited by the deposition of Bi2O3 on Co3O4. 10.0% Bi2O3/Co3O4 (S3) has the lowest PL emission peak, which is associated with its structural imperfection. The structural imperfection originating from the insertion of the Bi3+ into the lattice of Co3O4, as evidenced by XRD, increased the number of structural defects (e.g., oxygen vacancies), which could capture the electrons or holes, thus resulting in low radiative PL emission.
Fig. 6 shows the Raman spectra of 10.0% Bi2O3/Co3O4 (S3), pure Co3O4 (S1) and Bi2O3. For the pure Co3O4, the Raman peak at 655 cm−1 was corresponded to the symmetry of Co3O4.35 10.0% Bi2O3/Co3O4 gave the similar Raman spectra with Co3O4, while the characteristic peaks of Bi2O3 could not be detected. Compared with Co3O4, the Raman peaks on 10.0% Bi2O3/Co3O4 shifted to the lower frequencies with stronger intensities, which associated with the lattice distortion or residual stress of the spinel structure. The XRD results showed that part of Bi2O3 entered the lattice of Co3O4 over 10.0% Bi2O3/Co3O4, leading to the lattice distortion and lattice defect. The highly defective structure formed on 10.0% Bi2O3/Co3O4 could accelerate the adsorption and activation of O2, which was suggested to be related to the better catalytic performance.
3.5 Catalytic performance
Fig. 7 depicted the NO conversions over the Bi2O3/Co3O4 catalysts with different Bi2O3 contents (S1–S4), the physical mixture of 10% Bi2O3 nanoparticles and Co3O4 support (S5), 4% Ag/Al2O3 and 2% Pt/Al2O3 reference catalysts within the reaction temperature range of 100–500 °C. Co3O4 and Bi2O3/Co3O4 catalysts showed higher NO reduction activity than those of Ag/Al2O3 and Pt/Al2O3, especially Bi2O3/Co3O4 catalysts. The conversion of NO over Co3O4 support (S1) firstly increased with reaction temperature, reached the maximum conversion (ca. 60%) at ca. 300 °C and then decreased at higher temperature. The NO conversion was further increased with the addition of Bi2O3 into Co3O4 with the activity order: Co3O4 (S1) < 5.0% Bi2O3/Co3O4 (S2) < 15.0% Bi2O3/Co3O4 (S4) < 10.0% Bi2O3/Co3O4 (S3). Among all catalysts tested, 10.0% Bi2O3/Co3O4 (S3) possessed the highest activity for NO conversion in the reaction temperature window, reaching ca. 90% NO conversion at 200 °C. NO conversion under the lower reaction temperature (100–250 °C) over S3 also reached the highest among the catalysts tested. In contrast, the mixture of 10% Bi2O3 nanoparticles and Co3O4 support (S5) showed lower activity than S1 and S3. It was indicated that the interaction between Bi2O3 and Co3O4 in S3 is not the simply physical mixture like S5. The chemical interaction between them took place in S3 and should contribute the admirable catalytic performance of S3 in the C3H6-SCR reaction.
SO2 usually exists in the diesel engine exhaust. So it is necessary to investigate the SO2 tolerance of the catalyst in C3H6-SCR. Fig. 8 exhibited the effects of 100 ppm SO2 co-fed in the reaction gas on the NO conversions over the catalysts at the different reaction temperatures. NO conversion over the S3 (10% Bi2O3/Co3O4) catalyst clearly did not change in the wide reaction window. The steady-state NO conversion reached 90.3% on S3 at 250 °C in the presence and absence of SO2. In contrast, NO conversion decreased from 65.8% to 35.7% at 300 °C on the Co3O4 support (S1) when 100 ppm SO2 was contained in the feed gas. NO conversions at other reaction temperatures also reduced in the presence of SO2. These results obviously suggested that 10% Bi2O3/Co3O4 exhibited good resistibility against SO2 that coexists with NO and C3H6 in the reaction mixture.
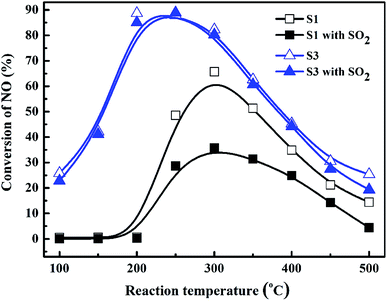 |
| Fig. 8 SO2 resistibilities of Co3O4 (S1) and 10.0% Bi2O3/Co3O4 (S3) with the reaction temperature. | |
Fig. 9 shows the SO2 durability of 10% Bi2O3/Co3O4 catalyst with the reaction time at the optimum reaction temperature 200 °C in the C3H6-SCR of NO. When NO conversion reached to the maximum (89.3%), 100 ppm SO2 was added in the reaction system, NO conversion immediately decreased. It was probably due to the competitive adsorption of NO and SO2 on the active site. 20 min later, NO conversion reduced to 63.6%. After that, NO conversion recovered to 85.7%, and maintained at ca. 88% through the whole reaction period of 90 min. This result further illustrates the outstanding SO2 resistibility of 10% Bi2O3/Co3O4 in the long time-reaction.
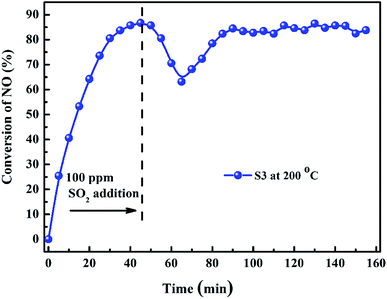 |
| Fig. 9 NO conversion over 10.0% Bi2O3/Co3O4 (S3) before and after the addition of SO2 (reaction temperature: 200 °C). | |
4 Discussions
In this study, the deposition of Bi2O3 with the proper loading amount on Co3O4 nanoplates enhanced NO conversion over Co3O4, especially at low reaction temperature (<200 °C). 10.0% Bi2O3/Co3O4 catalyst also showed the strong resistibility against SO2 in the feed gas. XRD results showed Bi2O3 could enter the lattice of Co3O4, and promote the formation of the lattice distortion and structural defect as demonstrated by PL spectra and IR spectra. The H2-TPR and XPS results showed that more Co3+ appeared with the deposition of Bi2O3. These changes were probably related to the promotive effects of Bi2O3 in the C3H6-SCR reaction.
Scheme 1 illustrated the mechanistic investigations for the HC-SCR reactions in the previous literatures.17,36,37 According to these findings, the reactants (C3H6, NO and NO2) are supposed to be first adsorbed on the active sites over the catalyst surface. Subsequently, the adsorbed nitrates formed via NO oxidation by O2. C3H6 was also activated to form CxHyOz species such as formate, acetate and so on. As these CxHyOz species become available, nitrates subsequently reacted with them to yield nitrogen-containing organic species, such as NCO species. The final step would be the interaction of NOC intermediates with NOx (NO, NOx), decomposing into N2, COx and H2O as final products. This proposed reaction process reveals the crucial role of O2 in the feed gas and the importance of oxidizing characteristics of the catalyst surface.
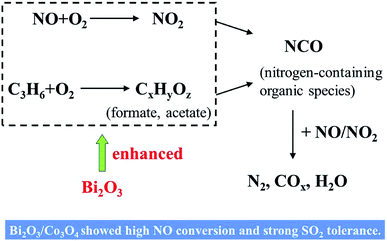 |
| Scheme 1 The promotive effects of Bi2O3 on NO reduction by propene over Co3O4 catalyst. | |
In our work, PL and IR results showed that more oxygen vacancies were produced on the Co3O4 after the doping of Bi2O3. The vacancy could accelerate the adsorption, activation and diffusion of oxygen, which was suggested to be available for the oxidation reactions involved in HC-SCR. The doped Bi2O3 also increased the Co3+ concentration on the surface. The richness of Co3+ could promote the adsorption and activation of NO and (or) C3H6. What is more is the mild oxidizing characteristics of bismuth oxide in the selective oxidation and ammoxidation of propene to acrolein and acrylonitrile. It will accelerate the formation of CxHyOz species. In short, the addition of Bi2O3 into the Co3O4 in the present study probably influenced the oxidation process in the C3H6-SCR reaction, favored the activation of C3H6 and NO, and then enhanced the following NCO intermediate formation and its decomposition with reaction with NOx to N2.
About poisoning HC-SCR catalyst with SO2, the previous studies reported that the suppression effect of SO2 on the SCR catalyst could attributed to the formation of sulphate on the catalyst.38 The presence of surface SO42− groups blocked the formation of nitrate and decreased the amount of adsorbed nitrates. Thus it hindered the transformation of NOC species and decreased the catalytic activity. In our work, the presence of 100 ppm SO2 in the feed gas decreased the NO conversion over Co3O4 catalyst, while the SO2 tolerance of Bi2O3/Co3O4 was strong. 10% Bi2O3/Co3O4 showed the good stability when SO2 was co-fed in the mixture gas during 90 min. This promotive role of Bi2O3 on the resistibility against SO2 also could be explained by the oxidizing properties of 10% Bi2O3/Co3O4. The addition of Bi2O3 into Co3O4 promoted the partial oxidation of propene activation of C3H6. The activation of C3H6 over 10% Bi2O3/Co3O4 seems more competitive in the compassion with the oxidization of SO2 to form surface sulfate. Subsequently, it is suggested that the addition of Bi2O3 into Co3O4 is one appropriate method for improving the SO2 resistance of Co3O4.
5 Conclusions
The optimum Bi2O3 loading on the Co3O4 nanoplates for the C3H6-SCR of NO was about 10%, giving the best catalytic activity, especially at low reaction temperature, as well as the strongest SO2 tolerance. The decoration of moderate Bi2O3 on Co3O4 influenced the oxidation state of Co3O4, facilitate the surface oxygen mobility and the partial oxidation of propene involved in the C3H6-SCR reaction. Therefore, the combination of Co3O4 with Bi2O3 is more active than Co3O4. The addition of 100 ppm SO2 to the feed hardly affected the catalytic performance of 10% Bi2O3/Co3O4.
Conflicts of interest
There are no conflict to declare.
Acknowledgements
This work was supported by the China National Natural Science Foundation (No. 51178360) and Hubei Superior and Distinctive Discipline Group of “Mechatronics and Automobiles” (XKQ2019058).
References
- C. R. Thomas, J. A. Pihl, M. J. Lance, T. J. Toops, J. E. Parks and J. Lauterbach, Effects of four-mode hydrothermal aging on three-way catalysts for passive selective catalytic reduction to control emissions from lean-burn gasoline engine, Appl. Catal., B, 2019, 244, 284–294 CrossRef CAS.
- Y. Rui, M. Ming, Z. Jing, L. Zheng, T. Hu and X. Li, A noble-metal-free SCR-LNT coupled catalytic system used for high-concentration NOx reduction under lean-burn condition, Catal. Today, 2018, 347–356 Search PubMed.
- J. A. Lupescu, J. W. Schwank, G. B. Fisher, J. Hangas, S. L. Peczonczyk and W. A. Paxton, Pd model catalysts: effect of air pulse length during redox aging on Pd redispersion, Appl. Catal., B, 2018, 223, 76–90 CrossRef CAS.
- Y. Hu and K. Griffiths, Selective Catalytic Reduction of NO by Hydrocarbons on a Stepped Pt Surface: Influence of SO2 and O2, J. Phys. Chem. C, 2015, 119, 19789–19801 CrossRef CAS.
- M. Khosravi, C. Sola, A. Abedi, R. E. Hayes, W. S. Epling and M. Votsmeier, Oxidation and selective catalytic reduction of NO by propene over Pt and Pt:Pd diesel oxidation catalysts, Appl. Catal., B, 2014, 147, 264–274 CrossRef CAS.
- P. Miquel, P. Granger, N. Jagtap, S. Umbarkar, M. Dongare and C. Dujardin, NO reduction under diesel exhaust conditions over Au/Al2O3 prepared by deposition-precipitation method, J. Mol. Catal. A: Chem., 2010, 322, 90–97 CrossRef CAS.
- X. Wang, N. Maeda and A. Baiker, Synergistic Effects of Au and FeOx Nanocomposites in Catalytic NO Reduction with CO, ACS Catal., 2016, 6, 7898–7906 CrossRef CAS.
- P. A. Kumar, M. P. Reddy, B. Hyun-Sook and H. H. Phil, Influence of Mg Addition on the Catalytic Activity of Alumina Supported Ag for C3H6-SCR of NO, Catal. Lett., 2009, 131, 85–97 CrossRef CAS.
- A. Musi, P. Massiani, D. Brouri, J.-M. Trichard and P. Da Costa, On the Characterisation of Silver Species for SCR of NOx with Ethanol, Catal. Lett., 2008, 128, 25–30 CrossRef.
- P. Namkhang and P. Kongkachuichay, Synthesis of Copper-Based Nanostructured Catalysts on SiO2–Al2O3, SiO2–TiO2, and SiO2–ZrO2 Supports for NO Reduction, J. Nanosci. Nanotechnol., 2015, 15, 5410–5417 CrossRef CAS.
- J. Liu, X. Li, Q. Zhao, D. Zhang and P. Ndokoye, The selective catalytic reduction of NO with propene over Cu-supported Ti–Ce mixed oxide catalysts: promotional effect of ceria, J. Mol. Catal. A: Chem., 2013, 378, 115–123 CrossRef CAS.
- Z. Liu, J. Li and J. Hao, Selective catalytic reduction of NOx with propene over SnO2/Al2O3 catalyst, Chem. Eng. J., 2010, 165, 420–425 CrossRef CAS.
- M. Chen, J. Yang, Y. Liu, W. Li, J. Fan, X. Ran, W. Teng, Y. Sun, W.-x. Zhang, G. Li, S. X. Dou and D. Zhao, TiO2 interpenetrating networks decorated with SnO2 nanocrystals: enhanced activity of selective catalytic reduction of NO with NH3, J. Mater. Chem. A, 2015, 3, 1405–1409 RSC.
- L. Zhang, L. Li, Y. Cao, Y. Xiong, S. Wu, J. Sun, C. Tang, F. Gao and L. Dong, Promotional effect of doping SnO2 into TiO2 over a CeO2/TiO2 catalyst for selective catalytic reduction of NO by NH3, Catal. Sci. Technol., 2015, 5, 2188–2196 RSC.
- D. Pietrogiacomi, M. C. Campa, L. R. Carbone, S. Tuti and M. Occhiuzzi, N2O decomposition on CoOx, CuOx, FeOx or MnOx supported on ZrO2: the effect of zirconia doping with sulfates or K+ on catalytic activity, Appl. Catal., B, 2016, 187, 218–227 CrossRef CAS.
- Y. Yu, Q. Zhong, W. Cai and J. Ding, Promotional effect of N-doped CeO2 supported CoOx catalysts with enhanced catalytic activity on NO oxidation, J. Mol. Catal. A: Chem., 2015, 398, 344–352 CrossRef CAS.
- F. Huang, W. Hu, J. Chen, Y. Wu, P. Qu, S. Yuan, L. Zhong and Y. Chen, Insight into Enhancement of NO Reduction with Methane by Multifunctional Catalysis over a Mixture of Ce/HZSM-5 and CoOx in Excess of Oxygen, Ind. Eng. Chem. Res., 2018, 57, 13312–13317 CrossRef CAS.
- L. Zhang, X. Yao, Y. Lu, C. Sun, C. Tang, F. Gao and L. Dong, Effect of precursors on the structure and activity of CuO-CoOx/gamma-Al2O3 catalysts for NO reduction by CO, J. Colloid Interface Sci., 2018, 509, 334–345 CrossRef CAS.
- B. Dou, G. Lv, C. Wang, Q. Hao and K. Hui, Cerium doped copper/ZSM-5 catalysts used for the selective catalytic reduction of nitrogen oxide with ammonia, Chem. Eng. J., 2015, 270, 549–556 CrossRef CAS.
- M. Sakmeche, A. Belhakem, R. Kessas and S. A. Ghomari, Effect of parameters on NO reduction by methane in presence of excess O2 and functionalized AlMCM-41 as catalysts, J. Taiwan Inst. Chem. Eng., 2017, 80, 333–341 CrossRef CAS.
- F. Bin, C. Song, G. Lv, J. Song, X. Cao, H. Pang and K. Wang, Structural Characterization and Selective Catalytic Reduction of Nitrogen Oxides with Ammonia: A Comparison between Co/ZSM-5 and Co/SBA-15, J. Phys. Chem. C, 2012, 116, 26262–26274 CrossRef CAS.
- D. Pietrogiacomi, A. Magliano, P. Ciambelli, D. Sannino, M. C. Campa and V. Indovina, The effect of sulphation on the catalytic activity of CoOx/ZrO2 for NO reduction with NH3 in the presence of O2, Appl. Catal., B, 2009, 89, 33–40 CrossRef CAS.
- A. Cabot, A. Marsal, J. Arbiol and J. R. Morante, Bi2O3 as a selective sensing material for NO detection, Sens. Actuators, B, 2004, 99, 74–89 CrossRef CAS.
- J. F. Brazdil, M. A. Toft, S. S. Y. Lin, S. T. McKenna, G. Zajac, J. A. Kaduk and J. T. Golab, Characterization of bismuth–cerium-molybdate selective propylene ammoxidation catalysts, Appl. Catal., A, 2015, 495, 115–123 CrossRef CAS.
- Y.-H. Lei and Z.-X. Chen, Theoretical study of propene oxidation on Bi2O3 surfaces, Sci. China: Chem., 2015, 58, 593–600 CrossRef CAS.
- R. B. Licht and A. T. Bell, A DFT Investigation of the Mechanism of Propene Ammoxidation over α-Bismuth Molybdate, ACS Catal., 2016, 7, 161–176 CrossRef.
- S. Kumar Megarajan, S. Rayalu, Y. Teraoka and N. Labhsetwar, High NO oxidation catalytic activity on non-noble metal based cobalt-ceria catalyst for diesel soot oxidation, J. Mol. Catal. A: Chem., 2014, 385, 112–118 CrossRef CAS.
- J.-W. Shi, G. Gao, Z. Fan, C. Gao, B. Wang, Y. Wang, Z. Li, C. He and C. Niu, NiyCo1−yMn2Ox microspheres for the selective catalytic reduction of NOx with NH3: the synergetic effects between Ni and Co for improving low-temperature catalytic performance, Appl. Catal., A, 2018, 560, 1–11 CrossRef CAS.
- D. Wang, Q. Wang and T. Wang, Morphology-controllable synthesis of cobalt oxalates and their conversion to mesoporous Co3O4 nanostructures for application in supercapacitors, Inorg. Chem., 2011, 50, 6482–6492 CrossRef CAS.
- J.-W. Shi, Z. Fan, C. Gao, G. Gao, B. Wang, Y. Wang, C. He and C. Niu, Mn–Co Mixed Oxide Nanosheets Vertically Anchored on H2Ti3O7 Nanowires: Full Exposure of Active Components Results in Significantly Enhanced Catalytic Performance, ChemCatChem, 2018, 10, 2833–2844 CrossRef CAS.
- Z. Zhu, G. Lu, Z. Zhang, Y. Guo, Y. Guo and Y. Wang, Highly Active and Stable Co3O4/ZSM-5 Catalyst for Propane Oxidation: Effect of the Preparation Method, ACS Catal., 2013, 3, 1154–1164 CrossRef CAS.
- H. Hu, S. Cai, H. Li, L. Huang, L. Shi and D. Zhang, In Situ DRIFTs Investigation of the Low-Temperature Reaction Mechanism over Mn-Doped Co3O4 for the Selective Catalytic Reduction of NOx with NH3, J. Phys. Chem. C, 2015, 119, 22924–22933 CrossRef CAS.
- G. Gao, J.-W. Shi, Z. Fan, C. Gao and C. Niu, MnM2O4 microspheres (M = Co, Cu, Ni) for selective catalytic reduction of NO with NH3: comparative study on catalytic activity and reaction mechanism via in situ diffuse reflectance infrared Fourier transform spectroscopy, Chem. Eng. J., 2017, 325, 91–100 CrossRef CAS.
- L. Wang, S. Zhang, Y. Zhu, A. Patlolla, J. Shan, H. Yoshida, S. Takeda, A. I. Frenkel and F. Tao, Catalysis and In Situ Studies of Rh1/Co3O4 Nanorods in Reduction of NO with H2, ACS Catal., 2013, 3, 1011–1019 CrossRef CAS.
- Y. Lou, L. Wang, Z. Zhao, Y. Zhang, Z. Zhang, G. Lu, Y. Guo and Y. Guo, Low-temperature CO oxidation over Co3O4-based catalysts: significant promoting effect of Bi2O3 on Co3O4 catalyst, Appl. Catal., B, 2014, 146, 43–49 CrossRef CAS.
- X. Chen, X. Yang, A. Zhu, C. T. Au and C. Shi, In situ DRIFTS study during C2H4-SCR of NO over Co-ZSM-5, J. Mol. Catal. A: Chem., 2009, 312, 31–39 CrossRef CAS.
- M. C. Campa, D. Pietrogiacomi, C. Scarfiello, L. R. Carbone and M. Occhiuzzi, CoOx and FeOx supported on ZrO2 for the simultaneous abatement of NOx and N2O with C3H6 in the presence of O2, Appl. Catal., B, 2019, 240, 367–372 CrossRef CAS.
- X. Xiao, S. Xiong, Y. Shi, W. Shan and S. Yang, Effect of H2O and SO2 on the Selective Catalytic Reduction of NO with NH3 Over Ce/TiO2 Catalyst: Mechanism and Kinetic Study, J. Phys. Chem. C, 2016, 120, 1066–1076 CrossRef CAS.
|
This journal is © The Royal Society of Chemistry 2019 |
Click here to see how this site uses Cookies. View our privacy policy here.