DOI:
10.1039/C9RA03276B
(Paper)
RSC Adv., 2019,
9, 23444-23449
Neodymium β-diketonate showing slow magnetic relaxation and acting as a ratiometric thermometer based on near-infrared emission†
Received
2nd May 2019
, Accepted 14th July 2019
First published on 29th July 2019
Abstract
Self-assembly of β-diketonate (Htta = thenoyl(trifluoro)acetone) and 4,4′-azopyridine (Azo-py) with neodymium(III) ions in the presence of methanol resulted in the formation of mononuclear complex [NdIII(TTA)3(MeOH)2]·0.5Azo-py (A) in which two asymmetric units are linked by Azo-py through hydrogen bonding via methanol. A reveals near-infrared emission (NIR) centred at about 895 and 1056 nm, in the 10–370 K temperature range, originating from the two emissive transitions on Nd(III) from 4F3/2 to 4I9/2 and 4I11/2 levels, respectively. Furthermore, the NIR luminescence intensity of A at room temperature augments two times upon thermal elimination of one coordinated methanol molecule. The thermally activated A exhibits single centre ratiometric thermometer behaviour in a wide temperature range from 10 to 300 K. Moreover, fluorescence properties of A were compared to another mononuclear complex [NdIII(TTA)3(4-OHpy)(H2O)] (B). Assembly A also exhibits field-induced slow magnetic relaxation properties with an energy barrier of ΔE/kB = 19.7(7) K and an attempt time of relaxation, τ0 = 3.7(8) × 10−7 s for fresh sample A, and ΔE/kB = 27.3 K and τ0 = 8.5(0) × 10−8 for assembly A after thermal treatment at 370 K.
Introduction
In recent years, much attention has been devoted to the synthesis and characterization of molecule-based materials with linkers (cyanide and thiocyanide) or chelating ligands (β-diketonates) showing various properties such as magnetism1 and luminescence.2 Especially, multifunctional materials with two or more physical properties have been broadly investigated which includes photo-magnetic,3 magneto-luminescent4 and humidity sensitive materials.5 The β-diketonate hybrid complexes are one of the longest explored class of chelating ligands due to their ability to stabilize the crystal framework which has found practical application in the design of technological devices.6 Meanwhile, trivalent lanthanides complexes have an intrinsic nature to exhibit excellent visible (VIS)7 and near-infrared (NIR)8 luminescence along with single molecule magnet (SMM) behavior due to sharp f–f electronic transitions and magnetic anisotropy. SMMs exhibiting magnetic hysteresis below a blocking temperature have potential in diverse research areas.9 Moreover, SMMs based on 4f-metal ions are promising candidates for luminescent magnets and have the flexibility to incorporate other functionalities. Thus, rare-Earth β-diketonates are suitable materials to be used in light-emitting diodes, spintronics and high density information storage.10 The potential hidden in molecule-based materials with lanthanide ions encourages us to look for molecular luminescent thermometers based on NIR emission. The working principle behind such thermometers is to utilize the temperature dependence of the luminescence, emission intensity, peak energy, integrated area of the peak, and lifetime or anisotropy. Recently, we have reported two molecular thermometers {[Tb1−xDyx(4-OHpy)2(H2O)3][Co(CN)6]}·0.5H2O (x = 0 and 0.5) based on cyanido-bridged metal assemblies showing colorometric as well as ratiometric thermometeric behaviour utilizing the intrinsic thermally reliant visible emission of TbIII and DyIII ions.11a In the pursuit to find multi-functional molecular thermometer, we synthesized neodymium β-diketonate complexes with molecular formula of [NdIII(TTA)3(MeOH)2]·0.5Azo-py (A), using organic ligand as a linkers, where TTA stands for trifluoro-1-(2-thienyl)-1,3-butanedione and Azo-py is 4,4′-azopyridine. Furthermore, we compared A with another fluorescent compound: [NdIII(TTA)3(4-OHpy)(H2O)] (B) to elucidate the mechanism of fluorescence effect in A.
Experimental
Materials
Neodymium(III) chloride hexahydrate (NdIIICl3·6H2O, CAS: 13477-89-9), 4,4,4-trifluoro-1-(2-thienyl)-1,3-butanedione (TTA, CAS: 326-91-0), 4-hydroxypyridine (4-OHpy, CAS: 626-64-2), 4,4′-azopyridine (Azo-py, CAS: 2632-99-7) and sodium hydroxide (NaOH, CAS: 1310-73-2) were purchased from commercial sources (Sigma-Aldrich, Tokyo Chemical Industry Co., Ltd., and Wako Pure Chemical Industries, Ltd).
Syntheses of [NdIII(TTA)3(MeOH)2]·0.5Azo-py (A) and [NdIII(TTA)3(4-OHpy)(H2O)] (B)
Assemblies A and B were obtained from 5 mL methanol solution of NdIIICl3·6H2O (0.6 mmol) and TTA (1.8 mmol) treated with NaOH (1.8 mmol) upon vigorous stirring. After 10 minutes of mixing, the purple filtrate X was collected using vacuum filtration. Successive addition of 5 mL methanol solution of Azo-py or 4-OHpy (0.6 mmol) to solution X and then slow evaporation for 4 days results in formation block shaped crystals of A and B, respectively. All products were collected by filtration, washed with small amount of methanol and used for further characterization. [NdIII(TTA)3(MeOH)2]·0.5Azo-py (A): yield: 414 mg (68%). Anal. calcd. for NdC31H24F9N2O8S3, (MW = 967 g mol−1): Nd, 14.9%; C, 38.5%; H, 2.8%; N, 2.9%; F, 17.7%; S, 9.95%. Found: Nd, 14.5%; C, 38.4%; H, 2.8%; N, 2.9%; F, 17.6%; S, 10.0%. [NdIII(TTA)3(4-OHpy)(H2O)] (B): yield: 380 mg (40%). Anal. calcd. for NdC30H23F9N1O9S3, (MW = 952.9 g mol−1): Nd, 15.3%; C, 37.8%; H, 2.4%; N, 1.5%; F, 17.9%; S, 10.1%. Found: Nd, 15.5%; C, 37.5%; H, 2.3%; N, 1.5%; F, 17.9%; S, 10.3%.
X-ray crystallography
Single-crystal diffraction measurements at 90 K for crystals of A and B, immersed in a paratone N-oil and mounted on Micro Mounts holder, were conducted using Rigaku R-axis RAPID diffractometer. Collected data were processed by using RAPID AUTO (Rigaku). Crystal structures were solved by a direct method with SIR2011 (ref. 12) for A and SHELXS97 (ref. 13) for B using CrystalStructure 4.2 crystallographic software package14 with the exception of refinement, which was performed using SHELXL-2018/3 (ref. 15) by a full-matrix least-squares technique. For disordered parts, isotropic refinements were performed. The disordered model was fixed with some restraints and constraints. Molecular graphics were prepared using Mercury 3.8 software.16
Physical techniques
Infrared absorption spectra were measured on a JASCO FTIR-4100 spectrometer for the pellet prepared by grinding together polycrystalline samples and KBr. UV-VIS-NIR diffuse reflectance spectra were performed by means of JASCO V-670 spectrophotometer for the powdered mixture of the crystalline sample and barium sulphate. The elemental analyses were performed by a standard microanalytical method. Thermogravimetric analysis of the polycrystalline samples were measured using Rigaku Thermo Plus TG8120 apparatus from 20 to 400 °C under an air atmosphere with a sweeping rate of 2 °C and with aluminium oxide as a reference. Emission and excitation spectra were measured on a Horiba Jobin-Yvon Fluorolog-3 (FL3-211) spectrofluorometer (model TKN-7) equipped with a Xe (450 W) lamp as an excitation source with the liquid nitrogen cooled InGaAs NIR linear array detectors working in a photon-counting mode. Temperature dependent NIR emission and excitation spectra were measured using the above spectrofluorometer and NIR detector where samples were mounted between two quartz glass inside the cryostat connected to a temperature controller. Temperature dependent UV-VIS-NIR absorption spectra were measured using Shimadzu UV-3600plus_MPC-603. Magnetic properties were measured using a Quantum Design MPMS XL magnetometer. The crystalline compounds were put with a paraffin oil to avoid the rotation of the sample under an applied magnetic field. The diamagnetic contributions from the sample, oil and the holder were estimated and corrected.
Computational details
DFT calculations were performed using the Gaussian 09 program package.17 The geometries of the models were not optimized and the coordinates from crystal structure are employed as the starting structure. Calculations were performed using the unrestricted Becke three-parameter hybrid functional with the Lee–Yang–Parr correlation functional (B3LYP)18 with the 6-31G(d,p) basis set. TD-DFT calculations were performed using UB3LYP to calculate the first 100 doublet transitions. Continuous Shape Measure Analysis for coordination spheres of [NdIII(TTA)3(MeOH)2] was performed using SHAPE software ver. 2.1.19
Results and discussion
Red crystals of A were synthesized following previously reported method20 under basic condition by mixing NdCl3·6H2O with HTTA and Azo-py in methanol (ESI†). Single-crystal X-ray diffraction analysis reveals that compounds A crystallize in the centrosymmetric monoclinic P21/c space group (Fig. 1, and S4–S6 and Tables S1–S3, ESI†). Asymmetric unit consists of eight coordinated NdIII ion connected by three TTA and two methanol molecules along with half equivalent of non-coordinated Azo-py. The crystalline material depicts eight-coordinated distorted square antiprism geometry around the Nd(III) ions as revealed by the continuous shape measure analysis (Table S3, ESI†). Similar geometry and ligands arrangement were observed for other LnIII-TTA systems.21 All 2-thiophene rings are oriented oppositely to the methanol connected to Azo-py in the pseudo “fac” geometry. Crystal packing of this complex is supported by hydrogen bonding between Azo-py and methanol (one methanol out of two in anisotropic unit), π⋯π interactions among 2-thiophene rings to another 2-thiophene and azo groups (Fig. S5, ESI†). The crystallinity and purity of bulk sample were confirmed by temperature-dependent powder X-ray diffraction measurements, infrared (IR) spectroscopy, thermogravimetric (TGA) and elemental analyses (Fig. S1–S3 and S7, ESI†). The optical properties of A including UV-VIS-NIR absorption, emission and excitation spectra measured at different temperatures are shown in Fig. 2 and S8–S14 (ESI†). The absorption spectra in the UV-VIS range have five characteristic peaks of Azo-py and TTA which are related to the combined contribution of singlet to singlet transitions (π → π*) from the organic ligands (Fig. S8–S10 and Table S4, ESI†). Additionally, sharp peaks in VIS-NIR region are assigned to the various f–f transitions coming from Nd3+ ion. NIR emission spectra of A reveal two sharp peaks centred around 895 and 1056 nm, originating from 4F3/2 to 4I9/2 and 4I11/2 transitions on neodymium(III), respectively (Fig. 2).22 The excitation spectra of A are centred around 360 nm with contribution exclusively from TTA and they also have sharp transitions of Nd3+ in the 450–650 nm range (Fig. 2b).
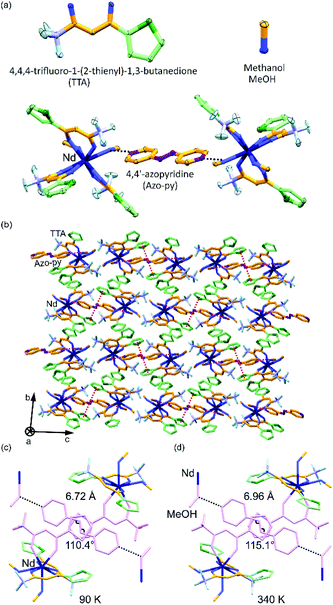 |
| Fig. 1 The structural unit of A with skeleton of β-diketonate ligand and methanol (a), the crystal packing along a crystallographic direction with partial overlap of Azo-py with 2-thiophene rings – red dotted lines and the hydrogen bonding between MeOH and Azo-py – orange dotted lines (b), and the view along b-axis with comparison of the deformed C–N–N bond angle and distance between two centroids of 2-thiophene ring at 90 K (c) and 340 K (d). Black dotted line indicates hydrogen bonding. | |
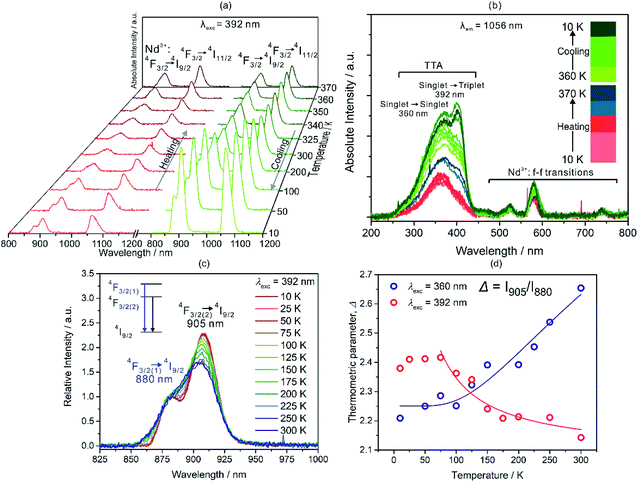 |
| Fig. 2 Solid state temperature dependent NIR emission spectra of A measured in 10 → 370 → 10 K temperature cycles for excitation wavelength, λexc, of 392 nm (a) and the corresponding excitation spectra of A collected at relevant emission wavelength, λem, of 1056 nm (b); the emission profile at 895 nm measured at various temperature for λexc = 392 nm (c). (d) Presents the temperature dependence of the thermometric parameter, Δ, for λexc = 392 and 360 nm together with the calibration curve (solid line) obtained from the best fit of the classical Mott–Seitz model involving two non-radiative recombination channels. | |
The very weak emission signal of A at room temperature might be caused by less efficient energy transfer from TTA to Nd(III). Consequently, we prepared reference compound [NdIII(TTA)3(4-OHpy)(H2O)](MeOH) (B) with coordinated 4-pyridinol (4-OHpy), (Fig. S1, S2, S6, S7 and S9 and Tables S1 and S2, ESI†), to confirm the previous observation. As indicated in Fig. S11 (ESI†) the emission and excitation spectra of B have relatively high intensity and the excitation spectra maxima compared to A are shifted to 392 nm which proves the role of coordinated 4-OHpy in transferring the energy efficiently to the NdIII. Moreover, to unravel the coordination effect on the luminescence properties, A was heated from 10 K to 370 K in the cryostat under 10−4 Pa pressure slowly by putting it between quartz plate with Nujol (crystal melted around 380 K under atmospheric pressure in single-crystal measurement, therefore T = 370 K was chosen for the experiment). The resulting emission spectra show gradual decrease of emission intensity from 10 K up to 300 K which can be attributed to the thermal activation of non-radiative relaxation pathways (Fig. 2a). Interestingly, emission intensity starts to increase after heating sample to 325 K which keep increasing until 370 K (Fig. 2a). On the other hand, the reverse cooling of the sample increases the emission intensity almost by five times at 10 K compared with pristine sample. Such behaviour can be explained by the release of the coordinated MeOH (Fig. S2 and S3, ESI†) and structural changes associated to this process which suppress non-radiative relaxation pathways and resulting in the more efficient energy transfer between TTA to Nd3+ ions.23c The reason can be supported by careful analysis of the excitation spectra (Fig. 2b). The peak intensity of the excitation spectra corresponding to transition from 0SX (singlet ground state) to 1SX (first singlet excited state) and to 3TX (triplet excited state) centred around 360 nm and 392 nm, respectively, changes with respect to temperature. For thermally stimulated sample triplet excited state gets populated more efficiently and transfers energy effectively to the neighbouring lanthanide ion due to better energy match. Moreover, we measured the single crystal structure of A at higher temperature of 340 K which indicated that C–N–N bond angles of Azo-py ring changes along with increase in the various bond distances (Fig. 1d and Table S1, ESI†). Upon looking at the temperature dependence of UV-VIS-NIR absorption and NIR emission spectra, we precisely studied the temperature dependent changes in the emission peak appearing at 895 nm in the range 10–300 K for 392 nm excitation light as well as 360 nm light (Fig. 2c, S10–S12 ESI†). The intensity ratio [(I(4F3/2(2) → 4I9/2)/I(4F3/2(1) → 4I9/2)] = Δ(T)) of these two peaks also known as thermometric parameter are plotted against temperature for 392 and 360 nm excitation light (Fig. 2d). The thermometric parameter strongly varies in 25–250 K for 392 nm and 10–300 K for 360 nm excitation wavelengths. For single centre ratiometric luminescent thermometers resulting data can be fitted by using Boltzmann population method (eqn (1), where Δ0 is the pre-exponential factor, ΔE0 is the energy difference between two emissive levels at reference temperature, C is offset factor, kB Boltzmann constant and T is absolute temperature; Δ0 (392) = 2.08(7), Δ0 (360) = 1.67(7), ΔE0/kB(392) = −11.70(6), ΔE0/kB(360) = 443.88(5), C(392) = 0, C(360) = 2.25(0), R2 = 0.91(5) (392) and 0.94(7) (360) for λexc = 392 and 360 nm respectively) which describes the thermal depopulation of two thermally coupled energy levels.11
|
Δ(T) = I905/I880 = Δ0 exp(−ΔE0/kBT) + C
| (1) |
The thermometric performance decreases with increasing temperature for λexc = 392 nm, however it increases for λexc = 360 nm which could be assigned to the difference in the population of thermally coupled emissive Stark levels 4F3/2(1) and 4F3/2(2) (Fig. 2d).23 The excitation by 392 nm populates triplet excited state of TTA more than the 360 nm light irradiation due to better energy matching therefore energy transfer in the former case is more efficient to the 4F3/2(1) Stark level which results into two opposing thermometric sensing ability (Fig. S12d and e, ESI†).
The sensitivity (Sr) and uncertainty (δT) for these two excitation wavelengths are computed using eqn (2) and (3) which indicated the best working temperature of 75–300 K (0.039 < Sr < 0.054 and 0.075 < δT < 1.993) and 10–300 K (0.02094 < Sr < 0.02098 and 0.017 < δT < 1.653) for 392 and 360 nm excitation wavelength, respectively (Fig. S12d, e, and S13, ESI†). In addition, we performed thermal heating–cooling cycle in the temperature range of 50–200 K which shows good repeatability (Fig. S15 and S16, ESI†).
|
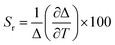 | (2) |
|
 | (3) |
The direct-current (dc) magnetic properties for A are indicated in Fig. S17, (ESI†). The χMT value for A at 300 K reaches 1.34 cm3 mol−1 K which is slightly lower than the estimated value of 1.64 cm3 mol−1 K for Nd3+ ions of the 4I9/2 ground multiplet. The decrease in the magnetization upon cooling can be explained by thermal depopulation of the mJ levels of ground electronic state. The saturation magnetization observed at 1.8 K in Hdc = 50 kOe equals 1.17 μB which is very close to the 1.27 μB of expected value for mJ = ±7/2 ground state with Ising anisotropy of gz = 5.1.22 The alternate-current (ac) magnetic studies (Fig. 3, S18 and S19, Table S5, ESI†) in the optimum dc field of 1000 Oe shows that A exhibits the single molecular magnet behaviour with energy barrier of ΔE/kB = 19.7(7) K, attempt time of relaxation, τ0 = 3.7(8) × 10−7 s and the τQTM−1 = 1300 s−1 after fitting with Arrhenius law indicated by eqn (4).
|
τ−1 = τQTM−1+ τ0−1 exp(−ΔE/kBT)
| (4) |
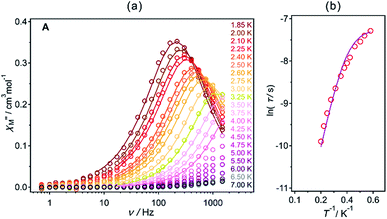 |
| Fig. 3 Ac magnetic properties of A (Hdc = 1000 Oe and Hac = 3 Oe): frequency (ν) dependences out-of-plane, (a) components of the magnetic susceptibility for the indicated temperatures in 1.85–7.0 K range and the temperature dependence of relaxation times (τ) presented as ln(τ) versus T−1 plots (b). Solid lines in (a) and (b) were fitted using the generalized Debye model (Table S8, ESI†) and eqn (4), respectively. | |
The magnetic-field induced single relaxation process can be assigned to the thermal relaxation behaviour with small contribution of quantum tunnelling of magnetization (QTM) at lower temperature. Thus, A acts as single molecule magnet generally rare among TTA based NdIII complex (Fig. 3 and Table S6, ESI†). We also measured the ac magnetic properties for the heated sample (in the similar condition like sample used for the luminescence measurement) under applied magnetic field of 1000 Oe. The heated sample of A shows slightly improved energy barrier of ΔE/kB = 27.3 K, attempt time of relaxation τ0 = 8.5(0) × 10−8 and the τQTM−1 = 1201 s−1 (Fig. S20, ESI†). The slight enhancement of energy barrier could be attributed to the fact that magnetic anisotropy of the Nd3+ ion improved due to release of two co-ordinating methanol (Fig. S2, ESI†).
Conclusions
In conclusion, we synthesized dinuclear coordination network [NdIII(TTA)3(MeOH)2]·0.5Azo-py (A) in which two asymmetric units are linked by an organic linker with hydrogen bonds. The crystalline compound reveals temperature dependent NIR emission as well as SMM properties. Upon heating the luminescence intensity increases by almost five times which is assigned to the thermally stimulated emission due to suppression of non-radiative relaxation pathways, hence, efficient energy transfers from TTA to Nd3+ ions. Additionally, NIR emission intensity can be modulated by temperature, which helped us to design a ratiometric thermometer in the temperature range 10–300 K. In the future, we will focus on the exploration of correlation between magnetic properties and ratiometric emission behaviour and the observation of the external stimuli effect on such phenomenon.
Conflicts of interest
There are no conflicts to declare.
Acknowledgements
The present research was partially supported by the Grant-in-Aid for Specially Promoted Research (Grant number 15H05697), Grants-in-Aid for Scientific Research on Innovative Areas Soft Crystals (area no. 2903, 17H06367) and APSA from MEXT. The Global Science course from MEXT, the Cryogenic Research Center in The University of Tokyo, and the Center for Nano Lithography & Analysis in The University of Tokyo supported by MEXT, and Quantum Leap Flagship Program (Q-LEAP) by MEXT are acknowledged.
Notes and references
-
(a) K. Kato, Y. Moritomo, M. Takata, M. Sakata, M. Umekawa, N. Hamada, S. Ohkoshi, H. Tokoro and K. Hashimoto, Phys. Rev. Lett., 2003, 91, 255502 CrossRef CAS PubMed
;
(b) T. Mahfoud, G. Molnar, S. Bonhommeau, S. Cobo, L. Salmon, P. Demont, H. Tokoro, S. Ohkoshi, K. Boukheddaden and A. Bousseksou, J. Am. Chem. Soc., 2009, 131, 15049 CrossRef CAS PubMed
;
(c) S. Ohkoshi, K. Imoto, Y. Tsunobuchi, S. Takano and H. Tokoro, Nat. Chem., 2011, 3, 564 CrossRef CAS PubMed
;
(d) S. Ohkoshi and H. Tokoro, Acc. Chem. Res., 2012, 45, 1749 CrossRef CAS PubMed
;
(e) D. M. Pajerowski, M. J. Andrus, J. E. Gardner, E. S. Knowles, M. W. Meisel and D. R. Talham, J. Am. Chem. Soc., 2010, 132, 4058 CrossRef CAS PubMed
;
(f) O. Sato, T. Iyoda, A. Fujishima and K. Hashimoto, Science, 1996, 272, 704 CrossRef CAS PubMed
;
(g) R. González, A. Acosta, R. Chiozzone, C. Kremer, D. Armentano, G. De Munno, M. Julve, F. Lloret and J. Faus, Inorg. Chem., 2012, 51, 5737 CrossRef PubMed
;
(h) J. Werner, Z. Tomkowicz, M. Rams, S. G. Ebbinghaus, T. Neumann and C. Näther, Dalton Trans., 2015, 44, 14149 RSC
;
(i) P. F. Yan, P. H. Lin, F. Habib, T. Aharen, M. Murugesu, Z. P. Deng, G. M. Li and W. B. Sun, Inorg. Chem., 2011, 50, 7059 CrossRef CAS PubMed
;
(j) M. Verdaguer, A. Bleuzen, V. Marvaud, J. Vaissermann, M. Seuleiman, C. Desplanches, A. Scuiller, C. Train, R. Garde, G. Gelly, C. Lomenech, I. Rosenman, P. Veillet, C. Cartier and F. Villain, Coord. Chem. Rev., 1999, 192, 1023 CrossRef
;
(k) J. S. Miller, Chem. Soc. Rev., 2011, 40, 3266 RSC
;
(l) K. R. Dunbar and R. A. Heintz, Prog. Inorg. Chem., 1996, 45, 283 Search PubMed
;
(m) B. Nowicka, O. Stefańczyk, D. Pinkowicz, S. Chorazy, R. Podgajny and B. Sieklucka, Coord. Chem. Rev., 2012, 256, 1946 CrossRef CAS
;
(n) F. Pointillart, O. Cador, B. Le Guennic and L. Ouahab, Coord. Chem. Rev., 2017, 346, 150 CrossRef CAS
. -
(a) J.-C. G. Bünzli and C. Piguet, Chem. Soc. Rev., 2005, 34, 1048 RSC
;
(b) K. Binnemans, Chem. Rev., 2009, 109, 4283 CrossRef CAS PubMed
;
(c) J. Rocha, L. D. Carlos, F. A. Almeida Paz and D. Ananias, Chem. Soc. Rev., 2011, 40, 926 RSC
;
(d) Y. Yamada, D. Koori, K. Mori and Y. Oshikawa, J. Coord. Chem., 2016, 69, 3735 CrossRef CAS
. -
(a) S. Ohkoshi, H. Tokoro, T. Hozumi, Y. Zhang, K. Hashimoto, C. Mathoniere, I. Bord, G. Rombaut, M. Verelst, C. C. D. Moulin and F. Villain, J. Am. Chem. Soc., 2006, 128, 270 CrossRef CAS PubMed
;
(b) S. Ohkoshi, Y. Yamada, T. Matsuda, Y. Tsunobuchi and H. Tokoro, Chem. Mater., 2008, 20, 3048 CrossRef CAS
;
(c) S. Ohkoshi, S. Takano, K. Imoto, M. Yoshikiyo, A. Namai and H. Tokoro, Nat. Photonics, 2014, 8, 65 CrossRef CAS
. -
(a) E. Chelebaeva, J. Larionova, Y. Guari, R. A. S. Ferreira, L. D. Carlos, F. A. Almeida Paz, A. Trifonov and C. Guerin, Inorg. Chem., 2009, 48, 5983 CrossRef CAS PubMed
;
(b) S. Chorazy, M. Arczynski, K. Nakabayashi, B. Sieklucka and S. Ohkoshi, Inorg. Chem., 2015, 54, 4724 CrossRef CAS PubMed
;
(c) J. Long, R. Vallat, R. A. S. Ferreira, L. D. Carlos, F. A. Almeida Paz, Y. Guari and J. Larionova, Chem. Commun., 2012, 48, 9974 RSC
;
(d) F. Pointillart, B. Le Guennic, S. Golhen, O. Cador and L. Ouahab, Chem. Commun., 2013, 49, 11632 RSC
. -
(a) S. Ohkoshi, K. Arai, Y. Sato and K. Hashimoto, Nat. Mater., 2004, 3, 857 CrossRef CAS PubMed
;
(b) K. Imoto, D. Takahashi, Y. Tsunobuchj, W. Kosaka, M. Arai, H. Tokoro and S. Ohkoshi, Eur. J. Inorg. Chem., 2010, 26, 4079 CrossRef
;
(c) N. Yanai, W. Kaneko, K. Yoneda, M. Ohba and S. Kitagawa, J. Am. Chem. Soc., 2007, 129, 3496 CrossRef CAS PubMed
. -
(a) N. B. D. Lima, A. I. S. Silva, P. C. Gerson, S. M. C. Gonçalves and A. M. Simas, PLoS One, 2015, 10, e0143998 CrossRef PubMed
;
(b) B. Rajamouli and V. Sivakumar, ChemistrySelect, 2017, 14, 4138 CrossRef
. -
(a) P. Chen, Q. Li, S. Grindy and N. Holten-Andersen, J. Am. Chem. Soc., 2015, 137, 11590 CrossRef CAS PubMed
;
(b) L. V. Meyer, F. Schönfeld and K. Müller-Buschbaum, Chem. Commun., 2014, 50, 8093 RSC
;
(c) S. Chorazy, K. Kumar, K. Nakabayashi, B. Sieklucka and S. Ohkoshi, Inorg. Chem., 2017, 56, 5239 CrossRef CAS PubMed
;
(d) K. S. Kisel, G. Linti, G. L. Starova, V. V. Sizov, A. S. Melnikov, A. P. Pushkarev, M. N. Bochkarev, E. V. Grachova and S. P. Tunik, Eur. J. Inorg. Chem., 2015, 2015, 1734 CrossRef CAS
;
(e) V. V. Khistiaeva, A. S. Melnikov, S. O. Slavova, V. V. Sizov, G. L. Starova, I. O. Koshevoy and E. V. Grachova, Inorg. Chem. Front., 2018, 5, 3015 RSC
. -
(a) N. M. Shavaleev, L. P. Moorcraft, S. J. A. Pope, Z. R. Bell, S. Faulkner and M. D. Ward, Chem. Commun., 2003, 10, 1134 RSC
;
(b) G. M. Davies, S. J. A. Pope, H. Adams, S. Faulkner and M. D. Ward, Inorg. Chem., 2005, 44, 4656 CrossRef CAS PubMed
;
(c) K. A. White, D. A. Chengelis, K. A. Gogick, J. Stehman, N. L. Rosi and S. Petoud, J. Am. Chem. Soc., 2009, 131, 18069 CrossRef CAS PubMed
;
(d) S. Chorazy, M. Rams, J. Wang, B. Sieklucka and S. Ohkoshi, Dalton Trans., 2017, 46, 13668 RSC
. -
(a) J. Kido and Y. Okamoto, Chem. Rev., 2002, 102, 2357 CrossRef CAS
;
(b) J. L. Sessler and R. A. Miller, Biochem. Pharmacol., 2000, 59, 733 CrossRef CAS PubMed
;
(c) J. D. Rinehart and J. R. Long, Dalton Trans., 2012, 41, 13572 RSC
. -
(a) I. V. Taydakov, A. A. Akkuzina, R. I. Avetisov, A. V. Khomyakov, R. R. Saifutyarov and I. Ch. Avetissov, J. Lumin., 2016, 177, 31 CrossRef CAS
;
(b) I. Savchenko, A. Berezhnytska, E. Trunova, N. Rusakova and G. Grozduyk, Mol. Cryst. Liq. Cryst., 2016, 640, 134 CrossRef CAS
. -
(a) K. Kumar, S. Chorazy, K. Nakabayashi, H. Sato, B. Siecklucka and S. Ohkoshi, J. Mater. Chem. C, 2018, 6, 8372 RSC
;
(b) H. Kusama, O. J. Sovers and T. Yoshioka, Jpn. J. Appl. Phys., 1976, 15, 2349 CrossRef CAS
;
(c) J.-C. G. Bünzli and V. K. Pecharsky, Handbook on the Physics and Chemistry of Rare Earths, Elsevier, 2016, ch. 281, vol. 49 Search PubMed
. - M. C. Burla, R. Caliandro, M. Camalli, B. Carrozzini, G. L. Cascarano, C. Giacovazzo, M. Mallamo, A. Mazzone, G. Polidori and R. Spagna, J. Appl. Crystallogr., 2012, 45, 357 CrossRef CAS
. - G. M. Sheldrick, Acta Crystallogr., Sect. A: Found. Crystallogr., 2008, 64, 112 CrossRef CAS PubMed
. - CrystalStructure Version 4.2, Rigaku Corporation, Tokyo, Japan, 2017 Search PubMed
. - G. M. Sheldrick, SHELXL 2018, University of Göttingen, Germany, 2018 Search PubMed
. - C. F. Macrae, I. J. Bruno, J. A. Chisholm, P. R. Edgington, P. McCabe, E. Pidcock, L. Rodriguez-Monge, R. Taylor, J. van der Streek and P. A. Wood, J. Appl. Crystallogr., 2008, 41, 466 CrossRef CAS
. - M. J. Frisch, G. W. Trucks, H. B. Schlegel, G. E. Scuseria, M. A. Robb, J. R. Cheeseman, G. Scalmani, V. Barone, B. Mennucci, G. A. Petersson, H. Nakatsuji, M. Caricato, X. Li, H. P. Hratchian, A. F. Izmaylov, J. Bloino, G. Zheng, J. L. Sonnenberg, M. Hada, M. Ehara, K. Toyota, R. Fukuda, J. Hasegawa, M. Ishida, T. Nakajima, Y. Honda, O. Kitao, H. Nakai, T. Vreven, J. A. Montgomery Jr, J. E. Peralta, F. Ogliaro, M. Bearpark, J. J. Heyd, E. Brothers, K. N. Kudin, V. N. Staroverov, T. Keith, R. Kobayashi, J. Normand, K. Raghavachari, A. Rendell, J. C. Burant, S. S. Iyengar, J. Tomasi, M. Cossi, N. Rega, J. M. Millam, M. Klene, J. E. Knox, J. B. Cross, V. Bakken, C. Adamo, J. Jaramillo, R. Gomperts, R. E. Stratmann, O. Yazyev, A. J. Austin, R. Cammi, C. Pomelli, J. W. Ochterski, R. L. Martin, K. Morokuma, V. G. Zakrzewski, G. A. Voth, P. Salvador, J. J. Dannenberg, S. Dapprich, A. D. Daniels, Ö. Farkas, J. B. Foresman, J. V. Ortiz, J. Cioslowski and D. J. Fox, Gaussian 09, Revision C.01, Gaussian, Inc., Wallingford CT, 2010 Search PubMed
. - A. D. Becke, J. Chem. Phys., 1993, 98, 5648 CrossRef CAS
. - M. Llunell, D. Casanova, J. Cirera, J. Bofill, P. Alemany, S. Alvarez, M. Pinsky and D. Avnir, SHAPE v. 2.1. Program for the Calculation of Continuous Shape Measures of Polygonal and Polyhedral Molecular Fragments, University of Barcelona, Barcelona, Spain, 2013 Search PubMed
. - C. Seward and S. Wang, Can. J. Chem., 2001, 79, 1187 CrossRef CAS
. -
(a) J. G. White, Inorg. Chim. Acta, 1976, 16, 159 CrossRef CAS
;
(b) V. Vallet, A. Fischer, Z. Szabo and I. Grenthe, Dalton Trans., 2010, 39, 7666 RSC
;
(c) P. S. Barber and A. de Bettencourt-Dias, Acta Crystallogr., Sect. E: Struct. Rep. Online, 2011, 67, m1188 CrossRef CAS PubMed
;
(d) G. Zucchi, P. Thuery, and M. Ephritikhine, CCDC 909451: Experimental Crystal Structure Determination, 2013, DOI:10.5517/cczjc4z
. - S. Chorazy, T. Charytanowicz, J. Wang, S. Ohkoshi and B. Sieklucka, Dalton Trans., 2018, 47, 7870 RSC
. -
(a) O. A. Savchuk, J. J. Carvajal, L. G. De la Cruz, P. Haro-Gonzalez, M. Aguilo and F. Diaz, J. Mater. Chem. C, 2016, 4, 7397 RSC
;
(b) U. Rocha, C. Jacinto, W. F. Silva, I. Guedes, A. Benayas, L. M. Maestro, M. A. Elias, E. Bovero, F. C. J. M. van Veggel, J. G. Solé and D. Jaque, ACS Nano, 2013, 7, 1188 CrossRef CAS PubMed
;
(c) A. M. Kaczmarek, D. Esquivel, J. Ouwehand, P. V. D. Voort, F. J. Romero-Salguero and R. V. Deun, Dalton Trans., 2017, 46, 7878 RSC
.
Footnote |
† Electronic supplementary information (ESI) available: Experimental details, IR and UV-Vis-NIR absorption spectra, TGA data, structural figures, structural parameters, PXRD patterns, additional emission and excitation spectra, ac magnetic plots, and parameters used for the fitting of Argand plots. CCDC 1900260–1900262. For ESI and crystallographic data in CIF or other electronic format see DOI: 10.1039/c9ra03276b |
|
This journal is © The Royal Society of Chemistry 2019 |