DOI:
10.1039/C9RA03268A
(Paper)
RSC Adv., 2019,
9, 23096-23108
Protective effects of macamides from Lepidium meyenii Walp. against corticosterone-induced neurotoxicity in PC12 cells†
Received
1st May 2019
, Accepted 9th July 2019
First published on 26th July 2019
Abstract
Maca has attracted considerable attention owing to its neuroprotective effects in vitro and vivo. Macamides, a series of nonpolar and long-chain fatty acid N-benzylamides, are considered unique constituents in maca. This study investigated the protective effects of ethanol extracts of maca (EEM) and macamides on corticosterone-induced (CORT) neurotoxicity in rat pheochromocytoma (PC12) cells. CORT reduced cell viability and increased LDH release, intracellular ROS levels, and MMP decline rate, and induced mitochondrial apoptosis. However, pretreatment with EEM and macamides ameliorated CORT-induced neurotoxicity. EEM increased the cell viability and reduced the LDH release. M 18:1, M 18:2, and M 18:3 increased cell viability and reduced LDH release and intracellular ROS generation. M 18:2 and M 18:3 inhibited MMP reduction and reduced the Bax/Bcl-2 ratios. M 18:1 reduced the intracellular ROS without affecting other factors. Moreover, M 18:3 prevented CORT-induced mitochondrial apoptosis, restrained the expression levels of pro-apoptotic proteins, namely, Bax, cytochrome C, cleaved-caspase-3, and cleaved-PARP, and increased the expression levels of Bcl-2. In addition, M 18:3 increased Akt phosphorylation and the ability of M 18:3 to protect against CORT-induced cytotoxicity was remarkably reduced by LY294002, a PI3K phosphorylation inhibitor. M 18:3 also elevated the phosphorylation of CREB and activated the BDNF protein levels in CORT-induced PC12 cells. In conclusion, macamides, especially M 18:3, exert protective effects on CORT-induced PC12 cells. The cellular mechanism of M 18:3 against CORT-induced cytotoxicity may involve inhibition of mitochondrial apoptosis, and activation of Akt and CREB phosphorylation. Overall, macamides may potentially treat neuronal damage induced by CORT.
1. Introduction
Chronic stress damages central neurons and causes nervous system disorders, such as depression, anxiety disorder, Alzheimer's disease, and Parkinson's disease.1 Under normal circumstances, the hypothalamic–pituitary–adrenal (HPA) axis controls the production of CORT, which exhibits a negative feedback regulation effect on the HPA axis. However, when the negative feedback is inhibited by chronic stress associated with multiple social factors, the HPA axis is dysregulated and over-activated. Thus, CORT continues to increase.2,3 Increased CORT can damage the nervous system, induce neurobiochemical changes in the brain, and cause chronic neurodegenerative diseases.1 High levels of CORT can cause pathological damage to hippocampal neurons in vitro and vivo,3–5 activate differentiated proteins, and induce apoptosis.4,6,7 Neuronal apoptosis is induced by CORT by several mechanisms, including the mitochondrial apoptosis pathway,8 endoplasmic reticulum stress,9 extracellular signal-regulated kinase 1 or 2 pathway,6 CREB protein pathway,10 and phosphoinositide 3-kinase/protein kinase B (PI3K/Akt) pathway.11 The PC12 cell line is derived from a transplantable rat pheochromocytoma, and large amounts of catecholamine, dopamine, norepinephrine, and CORT receptors are expressed in PC12 cells. Thus, PC12 cells exposed to CORT are widely used to study the neuronal damage associated with chronic stress.1,12
Maca (Lepidium meyenii Walp.) is a cruciferous plant that has been used as a functional food for centuries because of its high nutritional value and health benefits.13–15 Maca and its extracts exert various physiological activities such as anti-oxidative,16 anti-fatigue,17 and anti-osteoporotic effects,18 promote spermatogenesis,19 treat menopausal symptoms,20 and especially improve depression symptoms,21–23 improve cognitive function,24 and prevent memory impairment.25–27 Maca lipophilic extract containing macamides has shown neuroprotective effects against middle cerebral artery occlusion in vivo and H2O2 injury in vitro.28,29 Macamides, a series of nonpolar and long-chain fatty acid N-benzylamides, are considered as unique constituents in maca,30,31 exhibiting various biological activities, including anti-oxidative, anti-fatigue, and anti-osteoporotic activities,32–34 and activities related to improving the nervous system. Macamides are acetylcholinesterase (AChE) inhibitors.35 AChE can hydrolyze acetylcholine (ACh), and ACh deficiency was observed in patients with Alzheimer's disease. Inhibition of AChE is still a strategy for treating Alzheimer's disease. The AChE inhibitors play key roles in anti-inflammatory, anti-oxidant, and anti-apoptotic effects.36 Moreover, macamides are fatty acid amide hydrolase (FAAH) inhibitors,37–39 which can potentially treat inflammation and neurodegenerative diseases.40,41 FAAH inhibitors play a neuroprotective role by increasing endogenous anandamide to activate CB1, and prevent neuronal death in vitro and vivo.42–44 Recently, two papers have reported the neuroprotective effects of macamides. Zhou et al. reported that macamides protected against the dopaminergic neuron loss treated with 1-methyl-4-phenyl-1,2,3,6-tetrahydropyridine (MPTP) in zebrafish through the inhibition of AChE.35 Gugnani et al. reported that macamides exerted neuroprotective effects by binding to cannabinoid receptors 1 (CB1) in manganese (Mn)-induced U-87 MG glioblastoma cells.32 However, neuroprotective and anti-apoptotic effects of macamides against CORT-induced injury in PC12 cells remain unclear. Moreover, the liposolubility could make it easier for macamides to cross the blood–brain barrier and act within the central nervous system.28 Furthermore, chemical drugs such as fluoxetine and clozapine can protect against CORT-induced cytotoxicity in PC12 cells,45,46 but they exhibit hepatotoxicity (high doses of fluoxetine),47 myotoxicity,48 and hematopoietic toxicity.49 Therefore, researchers have focused on developing low-toxic and effective drugs from abundant medical herb resources. In addition, although many monomers exert neuroprotective effects, they need to be chemically synthesized or extracted and purified by complex techniques, which is inconvenient. Ethanol extracts are relatively easy to obtain. Our results showed that EEM also exerted neuroprotective effects in CORT-induced PC12 cells.
M 18:1, M 18:2, and M 18:3 (Fig. 1A) are the most representative and typical macamides. Thus, in this study, we hypothesize that the three macamides could counteract CORT-induced cytotoxicity. The effects of three macamides on CORT-induced cytotoxicity were compared. ROS levels, mitochondrial membrane potential (MMP) decline, mRNA and expression levels of Bax and Bcl-2 were determined. It was found that M 18:2 and M 18:3 possessed significant protective effects. However, based on the expression levels of Bax and Bcl-2, M 18:3 was chosen to investigate the effects on pro-apoptotic proteins and pro-survival proteins in order to further reveal macamides' neuroprotective mechanisms.
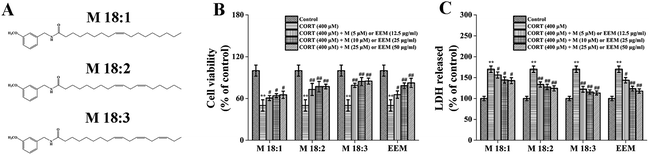 |
| Fig. 1 Neuroprotective effects of macamides (M 18:1, M 18:2, and M 18:3) and ethanol extract of maca (EEM) against CORT-induced injury in PC12 cells. (B) Effects of macamides (5–25 μM) and EEM (12.5–50 μg ml−1) on CORT-induced (400 μM) cell death in PC12 cells. (C) Effects of macamides (5–25 μM) and EEM (12.5–50 μg ml−1) on LDH leakage in CORT-treated (400 μM) PC12 cells. Results are expressed as mean ± SEM of six independent experiments. **P < 0.01 vs. control group; #P < 0.05 and ##P < 0.01 vs. CORT group. CORT: corticosterone. (A) Chemical structures of N-(3-methoxybenzyl)-9Z-octadecenamide (M 18:1), N-(3-methozybenzyl)-(9Z,12Z)-octadecadienamide (M 18:2), and N-(3-methozybenzyl)-(9Z,12Z,15Z)-octadecatrienamide (M 18:3). | |
2. Materials and methods
2.1. Materials
Macamides (batch number 20171212; ≥98% purity as determined by HPLC) were purchased from Huashite (Wuhan, China). CORT (batch number 103A031, purity ≥ 98%) was obtained from Solarbio (Beijing, China). FBS, HS, penicillin, and streptomycin were acquired from Gibco (Grand Island, USA). DMEM was provided by HyClone (GE, USA). The primary antibodies were obtained from Cell Signaling (CST, USA), Affinity Biosciences (Cincinnati, OH, USA), Abcam (Cambridge, UK), and Servicebio (Wuhan, China). All secondary antibodies were supplied by Servicebio (Wuhan, China).
2.2. Preparation of ethanol extract of maca (EEM)
Dried hypocotyls of maca were obtained from Lijiang BaiSuiFang Biotechnology Development Co. Ltd. (Yunnan, China). They were ground into a powder and passed through an 80-mesh sieve to obtain maca powder. Dried maca powder (10 g) was soaked in 100 ml of 95% ethanol (1/10, w/v) and reflux-extracted at 70 °C for 2 h twice. The extract was collected, filtered, and evaporated with a rotary evaporator at 50 °C to yield 1.97 g of EEM. To identify the active ingredients in EEM, we used a chromatographic technique. HPLC experiments comprised an UltiMate3000 system equipped with a UV-vis detector. Separation was performed using a C18 column (250 mm × 4.6 mm × 5 μm, Waters Sunfire) at 30 °C. The mobile phase was 90% acetonitrile with a flow rate of 0.6 mL min−1. EEM was dissolved using an appropriate volume of methanol and was filtered through a 0.45 μm syringe filter. The extract with an injection volume of 20 μL was added to HPLC vials and injected into the automatic sampler, and was detected at 210 nm. Macamides were qualitatively identified by comparing the retention times with the standards, and their contents were calculated based on the peak area. By HPLC analysis, the extract contained 750.23 mg/100 g total macamides.
2.3. Cell culture and treatment
PC12 cells were cultured using the method described by Ruile Pan.50 PC12 cells obtained from the Cell Bank of the Chinese Academy of Sciences (Shanghai, China) were routinely incubated in DMEM (high glucose) supplemented with 10% FBS, 5% HS, penicillin (100 U ml−1), and streptomycin (100 μg ml−1) at 37 °C in a humidified atmosphere of 95% air and 5% CO2. Cells in the exponential growth phase were used for every experiment. EEM, macamides, and CORT were dissolved in dimethylsulfoxide (DMSO) and then diluted with medium to various concentrations. The final concentration of DMSO was less than 0.1% (v/v). In order to determine the working concentrations of CORT in PC12 cells, the survival rate of PC12 cells was measured with MTT after incubation with different concentrations of CORT (50, 100, 200, 400, and 800 μM) for 24 h (Fig. S1†). To determine the protective effects of EEM and macamides on CORT-induced cytotoxicity, PC12 cells were divided into the following groups: untreated control, 400 μM CORT, 400 μM CORT plus EEM (12.5, 25, and 50 μg ml−1), and 400 μM CORT plus macamide (5, 10, and 25 μM). The treatments were conducted 24 h after the cells were seeded. EEM and macamides were applied 1 h prior to the treatment with 400 μM CORT. The cells were then co-incubated with macamides and CORT for another 24 h.
2.4. Cell viability
The cell viability was determined by MTT assay. PC12 cells (2 × 104 cells per well) were seeded into 96-well plates. After treatment of cells with 400 μM CORT at 37 °C for 24 h in the presence or absence of various concentrations of macamides or EEM, the solution of MTT was added, incubated for 4 h at 37 °C, and treated with DMSO for 15 min at 37 °C to dissolve the formazan crystals. Absorbance was determined at 570 nm using a microplate reader (BioTek, USA).
2.5. LDH release
The LDH release was assessed by a commercial assay kit. PC12 cells (1 × 105 cells per well) were seeded in 24-well plates. After treatment of cells with 400 μM CORT at 37 °C for 24 h in the presence or absence of various concentrations of macamides or EEM, the supernatant was incubated with NADH and 2,4-dinitrophenylhydrazine at 37 °C for 15 min, respectively. Then, NaOH was added to stop the reaction. The harvested cells were homogenized to determine the intracellular LDH activity. The amount of LDH released was measured by a microplate reader (440 nm).
2.6. Intracellular ROS level
Intracellular accumulation of ROS was measured after staining cells with DCFH-DA. PC12 cells (5 × 105 cells per well) were seeded in 6-well plates. After incubation with 25 μM macamides for 1 h, the cells were then co-incubated with 25 μM macamides plus 400 μM CORT for 24 h. The cells were harvested, washed with PBS, and then incubated with DCFH-DA (Beyotime, China, 10 mM for DCFH-DA) at 37 °C for 30 min in the dark. After incubation, the cells were washed with PBS and then analyzed with a FACS Calibur flow cytometer (Ex = 488 nm; Em = 530 nm) (FCMFC500MPL, Beckman Coulter, Fullerton, CA, USA). PC12 cells (5 × 105 cells per well) were seeded in 6-well plates. After the treatments, the cells were stained with DCFH-DA and observed by inverted fluorescence microscopy (IX71, Olympus, Japan).
2.7. Mitochondrial membrane potential
MMP was detected after staining cells with 5,5′,6,6′-tetrachloro-1,1′,3,3′-tetraethylbenzimidazolyl-carbo-cyanine iodide (JC-1). PC12 cells (5 × 105 cells per well) were seeded in 6-well plates. The cells were incubated with 25 μM macamides for 1 h, then followed by 25 μM macamides plus 400 μM CORT treatment. After drug treatment, the cells were harvested, washed with PBS, and then incubated with JC-1 (Beyotime, China, 2 mM for JC-1) at 37 °C for 30 min in the dark. After incubation, the cells were washed with PBS and then analyzed with a FACS Calibur flow cytometer (Ex = 488 nm; Em = 530 nm).
2.8. Quantitative real-time PCR
PC12 cells (5 × 105 cells per well) were seeded in 6-well plates. The cells were treated with various concentrations of macamides with CORT. After drug treatment, total RNA was extracted from PC12 cells using an RNA extraction kit (Servicebio, China). Total RNA was then reverse transcribed into cDNA using RevertAid First Strand cDNA Synthesis kit (Thermo, USA). Briefly, total RNA was incubated with oligo(dT)18 for 5 min at 65 °C, and then mixed with a 5× reaction buffer, dNTP Mix, RiboLock RNAse inhibitor, and RevertAid M-MuLV reverse transcriptase for 60 min at 42 °C. The reaction was maintained for 10 min at 70 °C to inactivate the reverse transcriptase. Quantitative real-time PCR was performed using the StepOne Plus Real-Time PCR System (ABI, USA) and FastStart Universal SYBR Green Master (Rox) (Roche, Switzerland). The forward and reverse primers were as follows: 5′-TGAACTGGACAACAACATGGAG-3′ and 5′-AGCAAAGTAGAAAAGGGCAACC-3′ for Bax, 5′-TTGTGGCCTTCTTTGAGTTCG-3′ and 5′-GCATCCCAGCCTCCGTTAT-3′ for Bcl-2, and 5′-CTGGAGAAACCTGCCAAGTATG-3′ and 5′-GGTGGAAGAATGGGAGTTGCT-3′ for GAPDH. Gel electrophoresis and melting curves were used to analyze the PCR products and the specificity of amplification. Transcript levels were quantified by the ΔΔCt value method using GAPDH for normalization.
2.9. Annexin V and PI double staining
The apoptosis rate was determined using a flow cytometer. PC12 cells (5 × 105 cells per well) were seeded in 6-well plates. The cells were incubated with various concentrations of M 18:3 for 1 h, then followed by various concentrations of M 18:3 plus 400 μM CORT treatment. After drug treatment, the cells were harvested, washed with PBS, and then incubated with the binding buffer containing 5 μl annexin V-FITC and 10 μl PI for 15 min at room temperature in the dark. The suspended cells were analyzed with a FACS Calibur flow cytometer (Ex = 488 nm; Em = 530 nm).
2.10. Western blot analysis
PC12 cells (5 × 105 cells per well) were seeded in 6-well plates. The cells were treated with various concentrations of macamides with CORT. After drug treatment, the PC12 cells were washed thrice with PBS, and homogenized with lysis buffer (Servicebio, China) containing protease and phosphatase inhibitors. The cells were scraped into 1.5 ml centrifuge tubes and incubated on ice for 30 min to ensure complete cell lysis. After the lysates were centrifuged at 12
000g for 10 min at 4 °C, the supernatant was collected for protein and western blot analyses. BCA kit (Servicebio, China) was used to determine the protein concentration. A Nuclear-Cytosol Extraction Kit (Servicebio, Wuhan, China) was used to extract the nuclear and cytoplasmic proteins. Equal amounts of proteins were separated by 10% SDS–PAGE, transferred onto nitrocellulose membrane (Millipore, USA), and incubated overnight at 4 °C with primary antibodies against cleaved-caspase-3 (1:500, Rabbit, Cell Signaling), Bax (1:2000, Rabbit, Abcam), cytochrome C (Cyt-C) (1:1000, Rabbit, Servicebio), cleaved-poly (ADP-ribose) polymerase (PARP) (1:500, Rabbit, Cell Signaling), Bcl-2 (1:1000, Rabbit, Abcam), cleaved-caspase-9 (1:1000, Rabbit, Servicebio), p-Akt (1:500, Rabbit, Affinity), Akt (1:500, Rabbit, Affinity), p-CREB (1:1000, Rabbit, Cell Signaling), CREB (1:1000, Rabbit, Cell Signaling), BDNF (1:1000, Rabbit, Servicebio), β-actin (1:3000, Mouse, Servicebio), and GAPDH (1:25
000, Mouse, Servicebio). After washing four times for 10 min with TBST, the membranes were incubated with the corresponding secondary antibodies conjugated with HRP (goat-anti-rabbit and goat-anti-mouse) at 37 °C for 1 h. Protein bands were detected using an enhanced chemiluminescence detection kit (Servicebio, China). The blots were quantified using an analytical system (Alpha Innotech, USA), with β-actin and GAPDH serving as the internal controls for the whole cell lysate and cytosolic fraction proteins.
2.11. Statistical analysis
The cell viability, the LDH release rate, and the levels of ROS, mRNA, and protein expression were presented as a percentage of the control. Cell apoptosis rates and MMP levels were expressed as a percentage of the total cells. Data were presented as mean ± SEM, and all experiments were performed for a minimum of four times. Statistical analysis was performed with SPSS (Version 19.0), and the differences between groups were determined using one-way analysis of variance followed by the least significance difference (LSD) test as appropriate. P-Values < 0.01 and <0.05 are indicated in the figures.
3. Results
3.1. Macamides and EEM ameliorated CORT-induced neurotoxicity in PC12 cells
CORT at 400 μM considerably decreased cell viability, which was approximately 50.5% ± 6.2% and showed concentration dependence (Fig. S1†). However, EEM at 12.5, 25, and 50 μg ml−1 remarkably increased their viability compared with that in the CORT group with the highest being 82.5% ± 6.5% at 50 μg ml−1. Compared with the CORT group, M 18:1, M 18:2, and M 18:3 at 5, 10, 25 μM remarkably increased the viability of PC12 cells and the highest was 79.1% ± 2.9%, 84.4% ± 5.7%, and 85.1% ± 4.3% for M 18:3, respectively. These results suggest that CORT causes damage to PC12 cells, whereas macamides and maca extract improve cell viability in CORT-induced PC12 cells (Fig. 1B).
LDH release is another important indicator of cell injury. CORT at 400 μM considerably increased the LDH release, which was about 170.2% ± 7.6% compared to the control group. However, EEM at 12.5, 25, and 50 μg ml−1 remarkably decreased the LDH release and the lowest was 117.8% ± 5.3% at 50 μg ml−1. Compared with the CORT group, M 18:1, M 18:2, and M 18:3 at 5, 10, 25 μM remarkably decreased the LDH release assay, the lowest was 122.1% ± 7.0%, 115.5% ± 5.3%, and 113.3% ± 4.8% for M 18:3, respectively. These results suggest that CORT increases LDH release whereas macamides and maca extract decrease LDH release in CORT-induced PC12 cells (Fig. 1C).
3.2. Effects of macamides on intracellular ROS generation in CORT-induced PC12 cells
Treatment with CORT at 400 μM considerably increased the ROS level in PC12 cells to 156.0% ± 8.7% compared to the control group. Compared with the CORT group, pretreatments with M 18:1, M 18:2, and M 18:3 at 25 μM remarkably reduced the ROS levels and the lowest was 98.6% ± 4.4% for M 18:3 (Fig. 2A). Exposure to CORT exhibited a stronger fluorescence intensity than the control group. Pretreatments with M 18:1, M 18:2, and M 18:3 at 25 μM reduced the fluorescence intensity, indicating reduced ROS generation (Fig. 2B). Thus, macamides reduced the CORT-induced ROS in PC12 cells.
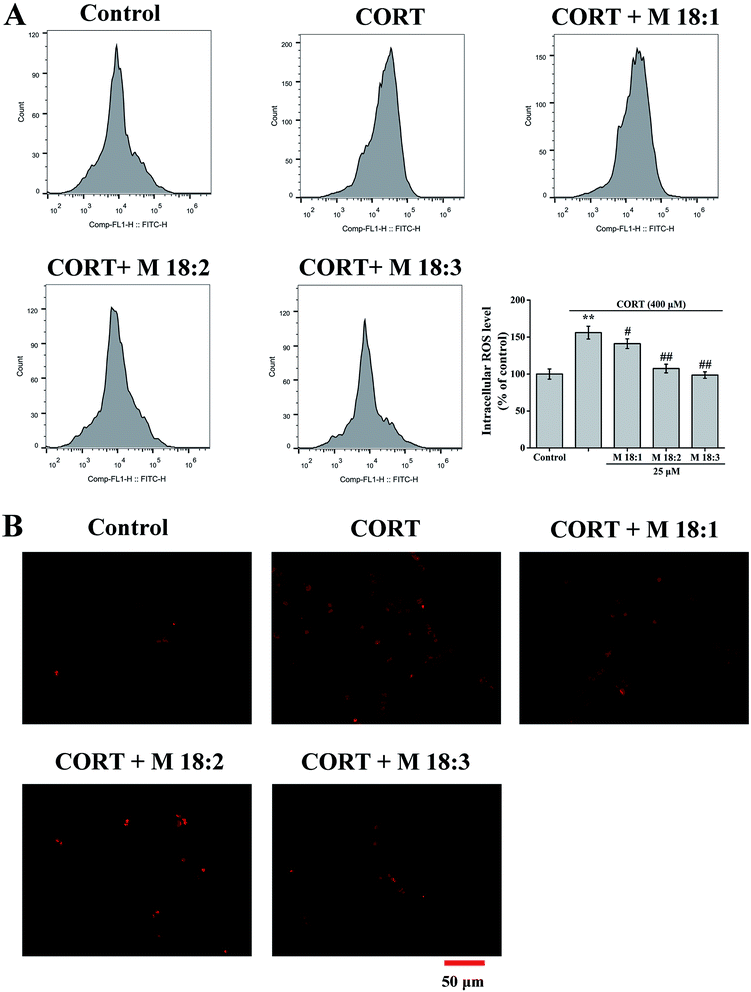 |
| Fig. 2 Effects of macamides (M 18:1, M 18:2, and M 18:3) (25 μM) on intracellular ROS levels in CORT-induced PC12 cells. (A) The bar chart describes ROS production. (B) DCFH-DA staining for intracellular ROS. Results are represented as mean ± SEM of four independent experiments. **P < 0.01 vs. control group; #P < 0.05 and ##P < 0.01 vs. CORT group. CORT: corticosterone. | |
3.3. Effects of macamides on MMP and Bax/Bcl-2 ratios in CORT-induced PC12 cells
CORT at 400 μM considerably increased the MMP decline rate in PC12 cells from 15.2% ± 1.4% to 84.9% ± 6.3%. Compared with the CORT group, pretreatment with 25 μM macamides remarkably reduced the MMP decline rates from 84.9% ± 6.3% to 36.5% ± 2.7% and 23.4% ± 1.9% with M 18:2 and M 18:3, respectively. 25 μM 18:1 did not remarkably decrease the MMP decline (Fig. 3A). Thus, M 18:2 and M 18:3 attenuated the reduction of MMP in CORT-treated PC12 cells.
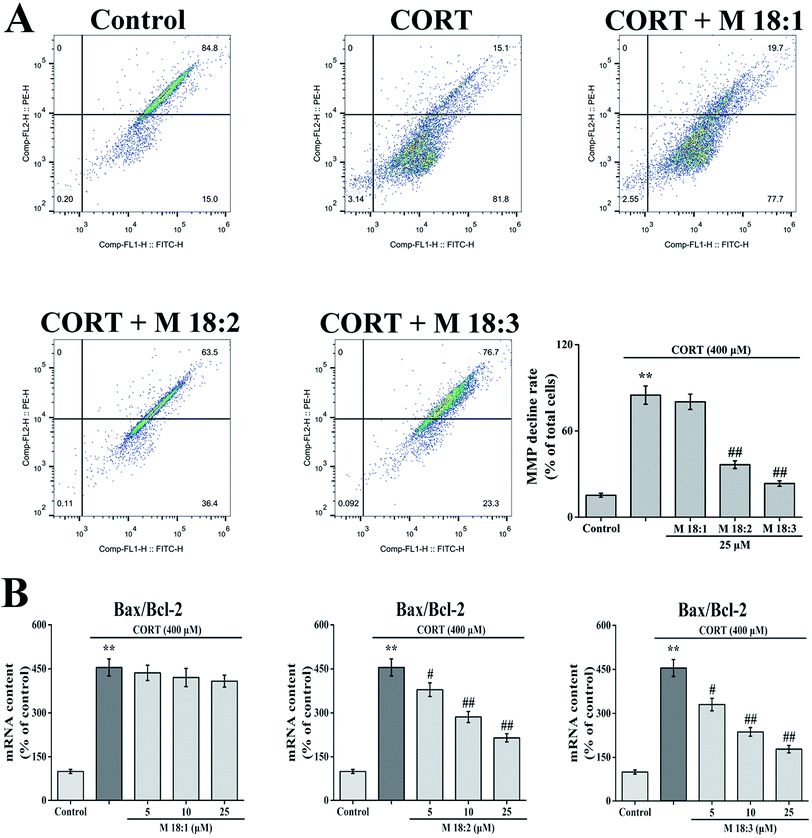 |
| Fig. 3 Effects of macamides (M 18:1, M 18:2, and M 18:3) on MMP depolarization and mRNA levels of Bax/Bcl-2 in CORT-induced PC12 cells. The bar chart of the deterioration of MMP (A) and the mRNA levels of Bax/Bcl-2 (B). Results are represented as mean ± SEM of four independent experiments. **P < 0.01 vs. control group; #P < 0.05 and ##P < 0.01 vs. CORT group. CORT: corticosterone. | |
After the cells were treated with 400 μM CORT, the Bax/Bcl-2 ratio was increased to 454.9% ± 28.9%. Compared with the CORT group, pretreatments with M 18:2 and M 18:3 at 5, 10, and 25 μM remarkably decreased the Bax/Bcl-2 ratios, the lowest were 330.1% ± 21.4%, 236.8% ± 14.5%, and 178.1% ± 12.7% for M 18:3, respectively. In addition, we found that M 18:1 at 5, 10, and 25 μM showed no evident effect on the mRNA levels of Bax and Bcl-2 (Fig. 3B). Thus, M 18:2 and M 18:3 could reduce the Bax/Bcl-2 ratios in CORT-induced PC12 cells.
Treatment with CORT at 400 μM considerably increased the expression level of Bax in PC12 cells compared to the control group. Compared with the CORT group, pretreatments with M 18:2 and M 18:3 at 5, 10, and 25 μM remarkably reduced the expression levels of Bax. 400 μM CORT considerably reduced the expression level of Bcl-2. However, pretreatments of PC12 cells with M 18:2 and M 18:3 at 5, 10, and 25 μM remarkably increased the expression levels of Bcl-2. M 18:1 at 5, 10, and 25 μM showed no evident effect on the expression levels of Bax and Bcl-2 (Fig. 4). Thus, M 18:2 and M 18:3 could reduce the Bax expressions and increase the Bcl-2 expressions in CORT-induced PC12 cells.
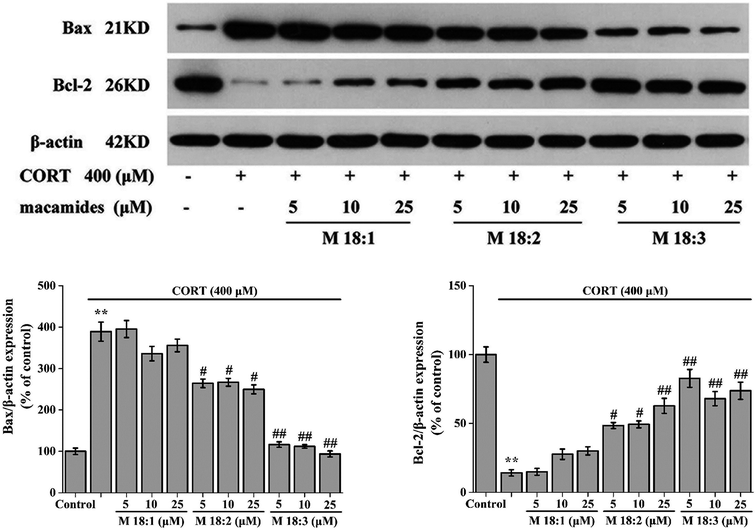 |
| Fig. 4 Effects of macamides (M 18:1, M 18:2, and M 18:3) on the expression levels of Bax and Bcl-2 in CORT-induced PC12 cells. Western blot analysis and quantification of immunoblot for the ratio of Bax and Bcl-2 to β-actin, respectively. Results are represented as mean ± SEM of four independent experiments. **P < 0.01 vs. control group; #P < 0.05 and ##P < 0.01 vs. CORT group. CORT: corticosterone. | |
3.4. Effects of macamide (M 18:3) on apoptosis and the expressions of pro-apoptotic proteins in CORT-induced PC12 cells
Treatment with 400 μM CORT increased the percentages of early apoptotic (EA) cells, EA cells + late apoptotic (LA) cells, and LA cells + necrotic cells to 14.6% ± 0.6%, 31.3% ± 1.3%, and 19.9% ± 0.8%, respectively. Pretreatment with M 18:3 at 5, 10, and 25 μM remarkably reduced the percentages of these cells and the lowest were 10.1% ± 0.4%, 15.0% ± 0.7%, and 7.8% ± 0.3% at 25 μM, respectively (Fig. 5). Thus, macamides inhibited CORT-induced apoptosis in PC12 cells. CORT treatment considerably increased the expression levels of cleaved-caspase-3, Bax, cytochrome C, and cleaved-PARP and remarkably decreased the expression level of Bcl-2. However, pretreatment with M 18:3 at 5, 10, and 25 μM reversed these trends in PC12 cells (Fig. 6). Thus, M 18:3 could inhibit apoptosis, reduce the expression of pro-apoptotic proteins and increase Bcl-2 expression in CORT-induced PC12 cells.
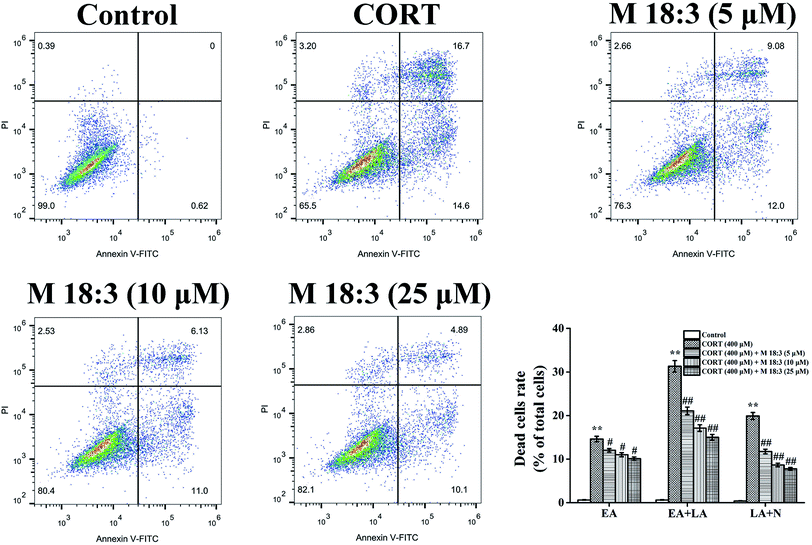 |
| Fig. 5 Protective effect of macamide (M 18:3) (5 μM, 10 μM, 25 μM) against apoptosis in CORT-treated PC12 cells. The bar chart describes the perceptual distribution of apoptotic (early apoptosis, EA; late apoptosis, LA; and necrosis, N) cells. Results are represented as mean ± SEM of four independent experiments. **P < 0.01 vs. control group; #P < 0.05 and ##P < 0.01 vs. CORT group. CORT: corticosterone. | |
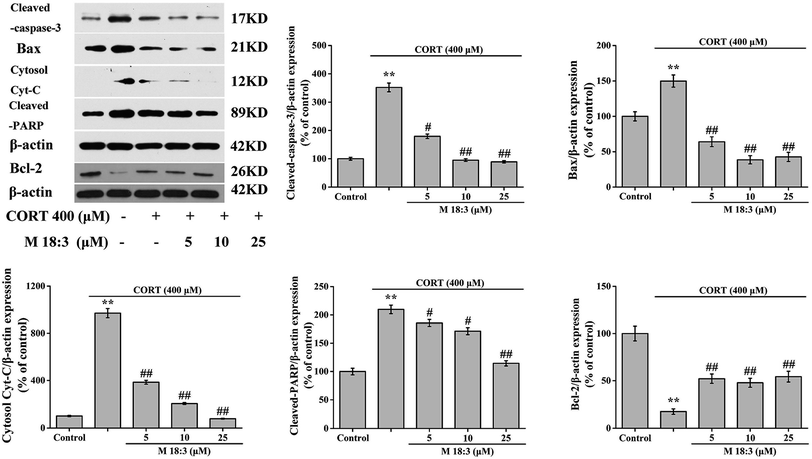 |
| Fig. 6 Protective effect of macamide (M 18:3) (5 μM, 10 μM, 25 μM) against apoptosis in CORT-treated PC12 cells. Western blot analysis and quantification of immunoblot for the ratio of cleaved-caspase-3, Bax, cytochrome C, cleaved-PPAR and Bcl-2 to β-actin, respectively. Results are represented as mean ± SEM of four independent experiments. **P < 0.01 vs. control group; #P < 0.05 and ##P < 0.01 vs. CORT group. CORT: corticosterone. | |
3.5. Effects of macamide (M 18:3) on the expression levels of pro-survival proteins (Akt, CREB and BDNF) in CORT-induced PC12 cells
Treatment of PC12 cells with 10 μM LY294002 for 24 h had no effect on the viability of normal cells. When PC12 cells were treated with 400 μM CORT for 24 h, cell viability was decreased significantly. However, after PC12 cells were pretreated with M 18:3 for 1 h and then incubated with CORT for 24 h, cell viability was significantly increased. Interestingly, when PC12 cells were pretreated with 10 μM LY294002 for 1 h followed by incubation with M 18:3 for 1 h, and were finally incubated with M 18:3 and CORT for 24 h, cell viability was significantly reduced (Fig. 7).
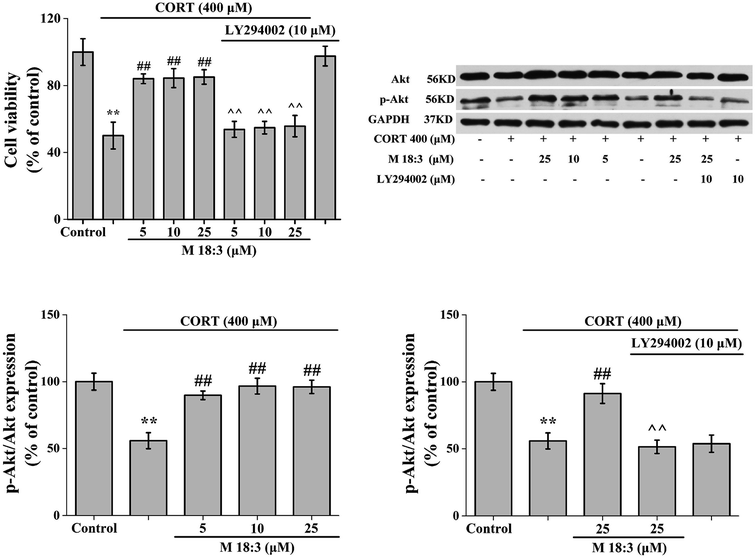 |
| Fig. 7 Macamide (M 18:3) (5 μM, 10 μM, 25 μM) increased Akt phosphorylation in CORT-induced PC12 cells. M 18:3 significantly increased CORT-induced decrease in cell viability, which was blocked by the PI3K/Akt-inhibitor LY294002. Western blot analysis showed that M 18:3 significantly increased the ratio of p-Akt/Akt, which was blocked by LY294002. Results are represented as mean ± SEM of four independent experiments. **P < 0.01 vs. control group; #P < 0.05 and ##P < 0.01 vs. CORT group; ^^P < 0.01 vs. CORT + M 18:3 group. CORT: corticosterone. | |
The expression level of Akt was slightly increased by 25 μM M 18:3, but not remarkably, whereas 400 μM CORT considerably reduced the expression level of p-Akt. However, pretreatment of PC12 cells with M 18:3 remarkably increased the expression level of p-Akt. Interestingly, when PC12 cells were pretreated with LY294002, the above trend was reversed. At the same time, there was no significant change in p-Akt levels in the inhibitor LY294002 plus CORT group compared with that in the CORT group, indicating that the inhibitor LY294002 could not cause interference with the system (Fig. 7).
CORT at 400 μM considerably decreased the expression levels of p-CREB/CREB and BDNF. However, pretreatment with M 18:3 at 5, 10, and 25 μM remarkably increased the expression levels of p-CREB/CREB and BDNF (Fig. 8). In summary, the above results showed that treatment with CORT reduced the expression levels of p-Akt, p-CREB, and BDNF whereas pretreatment with M 18:3 increased these.
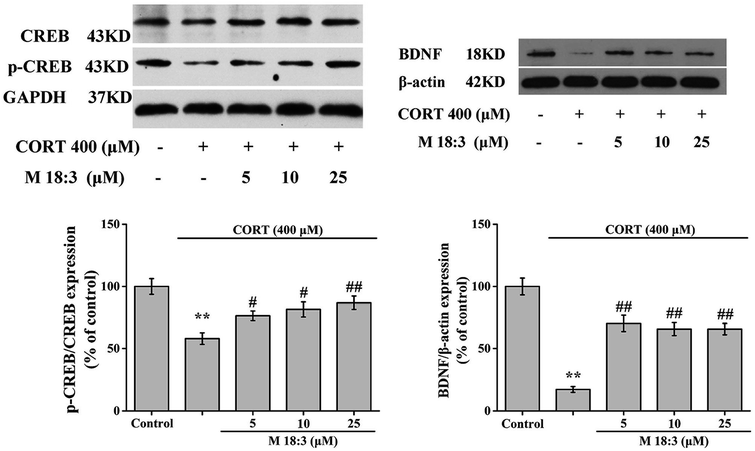 |
| Fig. 8 Macamide (M 18:3) (5 μM, 10 μM, 25 μM) increased the expression levels of p-CREB/CREB and BDNF in CORT-induced PC12 cells. Results are represented as mean ± SEM of four independent experiments. **P < 0.01 vs. control group; #P < 0.05 and ##P < 0.01 vs. CORT group. CORT: corticosterone. | |
4. Discussion and conclusions
This study demonstrated that CORT treatment decreased cell viability and increased LDH release. However, EEM and macamides increased the cell viability and decreased LDH release, among which M 18:3 was superior to EEM. These results showed that EEM and macamides exert protective effects against CORT-induced cytotoxicity, and that macamides may be potential neuroprotectants in EEM. Gugnani et al. investigated the neurotoxicity of Mn and found that macamides can counter this toxicity by binding to the CB1 receptor.32 Mn is an environmental toxin that could cause Parkinson's disease. However, we used CORT, a hormone secreted by mammals, to damage PC12 cells as excessive CORT can cause nervous system disorders related to chronic stress.1
Long-term exposure to high levels of glucocorticoids increases intracellular ROS.51,52 Excessive ROS levels in cells can disrupt redox balance, damage biomacromolecules, and activate Bax to cause apoptosis.53 M 18:2 and M 18:3 reduced the intracellular ROS generation, inhibited MMP decline rates, and reduced the Bax/Bcl-2 ratios. These results suggest that M 18:2 and M 18:3 might protect PC12 cells from CORT-induced mitochondrial injury by reducing oxidative stress at least in part. M 18:1 increased the cell viability and reduced intracellular ROS without producing other beneficial effects. These results showed that M 18:1 possessed weaker anti-apoptosis activity compared to M 18:2 and M 18:3 against CORT-induced cytotoxicity in PC12 cells. In this study, all three macamides exhibited protective effects against CORT-induced cell damage. However, M 18:2 and M 18:3 exhibited better protective effects. It is speculated that with an increase in the number of double bonds, the ability of macamide to scavenge ROS was enhanced and the ability to inhibit the damage produced by CORT was also enhanced. Similar experimental results were obtained with oleic acid, which with one double bond is structurally similar to M 18:1, and with alpha-linolenic acid (ALA), which with three double bonds is structurally similar to M 18:3. These fatty acids protect neurons by lowering ROS levels, which may be result from the scavenge of free radicals through their double bonds, rather than by increasing the activity of antioxidant enzymes.54,55
The Bcl-2 family proteins exists on the membranes of cells as well as the nuclear, mitochondrial, and endoplasmic reticulum membranes. There are several subtypes including the pro-apoptotic protein Bax, pro-apoptotic protein Bid, and anti-apoptotic protein Bcl-2.56 Bax is involved in the formation of mitochondrial permeability transporter pores (MPTPs). When MPTPs are opened, mitochondrial depolarization and MMP decrease are observed, and various cytokines in mitochondria such as Cyt-C, Smac, AIF, and EndoG, are released to the cytoplasm to induce caspase and mitochondrial dependent apoptosis.57,58 Bcl-2 can competitively bind to Bax on the mitochondrial membrane to form a Bcl-2/Bax heterodimer resulting in closing of the MPTPs and preventing the release of pro-apoptotic factors from mitochondria, thus achieving an anti-apoptotic effect.53 High levels of CORT cause nerve cell apoptosis in vitro and vivo.6,7,12,59 Zhou et al. suggested a potential mechanism of toxicity by CORT: CORT causes a large increase in the levels of intracellular ROS, decreases MMP, releases cytochrome C, activates caspase-3, and all of this inducing apoptosis.53 In our experiment, CORT remarkably increased the number of early and late apoptotic cells, whereas the number of apoptotic cells was considerably reduced after M 18:3 treatment. Moreover, CORT increased the expression levels of cleaved-caspase-3, Bax, cytochrome C, and cleaved-PARP, and decreased the expression level of Bcl-2. However, M 18:3 reduced the Bax/Bcl-2 ratio and the expression levels of Bax, cytochrome C, cleaved-caspase-3, and cleaved-PARP. These results suggested that M 18:3 could inhibit mitochondrial apoptosis and regulate the pro-apoptotic proteins.
The PI3K/Akt signaling pathway is one of the most important pathways involved in regulating cell proliferation.60 Activation of the PI3K/Akt pathway prevents apoptosis. PI3K enhances neuroprotective effects through phosphorylation of Akt, which is a pro-survival kinase. Through the PI3K pathway, Akt is phosphorylated at the Ser473 site, playing a key role in the survival of neurons. Phosphorylated Akt affects the downstream apoptosis-related proteins such as Bcl-2 family proteins, GSK-3β, and caspase-3, and plays a protective role against apoptosis.61,62 In this study, CORT considerably reduced the expression level of p-Akt, whereas M 18:3 at 5, 10, and 25 μM increased the expression levels of p-Akt. When the PC12 cells were pretreated with the PI3K/Akt inhibitor LY294002, the ability of M 18:3 to induce phosphorylation of Akt was inhibited. Moreover, when LY294002 was added, the ability of M 18:3 to protect against CORT-induced cytotoxicity was remarkably reduced. These results suggest that M 18:3 could promote the phosphorylation of Akt in CORT-induced PC12 cells. Similar experimental results are also reported previously. ALA, which is structurally similar to M 18:3, promotes Akt phosphorylation in high glucose-induced cultured HUVECs.63 Long-term dietary intake of ALA activates Akt signaling in the hippocampus of natural aging rats.64
CREB is a transcription factor involved in critical functions such as neuronal plasticity and survival. CREB is phosphorylated at Ser133, and p-CREB is its active form. Activated CREB can improve cell survival by regulating BDNF expression,65,66 which is considered an important factor for neuronal survival.35 In this study, M 18:3 increased the expression levels of p-CREB and BDNF. This result suggested that M 18:3 might improve the phosphorylation of CREB, and then activate the BDNF protein synthesis thereby attenuating CORT-induced neurotoxicity.
In conclusion, macamides, especially M 18:3, could inhibit CORT-induced neurotoxicity in PC12 cells. The cellular mechanism of M 18:3 against CORT-induced cytotoxicity may involve decrease in intracellular ROS, inhibition of mitochondrial apoptosis, and activation of Akt and CREB phosphorylation. Overall, macamides may potentially treat neuronal damage induced by CORT.
Conflicts of interest
No conflicts of interest are declared for any of the authors.
Abbreviations
LDH | Lactate dehydrogenase |
ROS | Reactive oxygen species |
MMP | Mitochondrial membrane potential |
FBS | Fetal bovine serum |
HS | Horse serum |
DMEM | Dulbecco's modified Eagle medium |
PI3K/Akt | Phosphoinositide 3-kinase/protein kinase B |
CREB | cAMP-response element binding protein |
BDNF | Brain-derived neurotrophic factor |
HUVECs | Human umbilical vein endothelial cells |
Acknowledgements
This research work was supported by National Natural Science Foundation of China (Grant No. J1103514), Nature Science Foundation of Hubei Province (No. 2012FFB02607). The authors thank the Analytical and Testing Center of Huazhong University of Science and Technology for the LC-MS analysis of macamides.
References
- Y. Noh, S. Cheon, I. H. Kim, I. Kim, S. A. Lee, D. H. Kim and Y. Jeong, The protective effects of ethanolic extract of Clematis terniflora against corticosterone-induced neuronal damage via the AKT and ERK1/2 pathway, BMB Rep., 2018, 51, 400–405 CrossRef CAS PubMed.
- M. Aihara, I. Ida, N. Yuuki, A. Oshima, H. Kumano, K. Takahashi, M. Fukuda, N. Oriuchi, K. Endo, H. Matsuda and M. Mikuni, HPA axis dysfunction in unmedicated major depressive disorder and its normalization by pharmacotherapy correlates with alteration of neural activity in prefrontal cortex and limbic/paralimbic regions, Psychiatry Res., Neuroimaging, 2007, 155, 245–256 CrossRef CAS PubMed.
- F. Murray, D. W. Smith and P. H. Hutson, Chronic low dose corticosterone exposure decreased hippocampal cell proliferation, volume and induced anxiety and depression like behaviours in mice, Eur. J. Pharmacol., 2008, 583, 115–127 CrossRef CAS PubMed.
- M. Y. Zhu, W. P. Wang and G. Bissette, Neuroprotective effects of agmatine against cell damage caused by glucocorticoids in cultured rat hippocampal neurons, Neuroscience, 2006, 141, 2019–2027 CrossRef CAS PubMed.
- H. Chen, G. N. Pandey and Y. Dwivedi, Hippocampal cell proliferation regulation by repeated stress and antidepressants, NeuroReport, 2006, 17, 863–867 CrossRef PubMed.
- M. Gao, H. Zhou and X. Li, Curcumin protects PC12 cells from corticosterone-induced cytotoxicity: possible involvement of the ERK1/2 pathway, Basic Clin. Pharmacol. Toxicol., 2009, 104, 236–240 CrossRef CAS.
- M. Zheng, C. Liu, F. Pan, D. Shi, F. Ma, Y. Zhang and Y. Zhang, Protective effects of flavonoid extract from Apocynum venetum leaves against corticosterone-induced neurotoxicity in PC12 cells, Cell. Mol. Neurobiol., 2011, 31, 421–428 CrossRef CAS PubMed.
- B. P. Jiang, Y. M. Liu, L. Le, Z. Y. Li, J. Y. Si, X. M. Liu, Q. Chang and R. L. Pan, Cajaninstilbene acid prevents corticosterone-induced apoptosis in PC12 cells by inhibiting the mitochondrial apoptotic pathway, Cell. Physiol. Biochem., 2014, 34, 1015–1026 CrossRef CAS PubMed.
- Y. Liu, S. Shen, Z. Li, Y. Jiang, J. Si, Q. Chang, X. Liu and R. Pan, Cajaninstilbene acid protects corticosterone-induced injury in PC12 cells by inhibiting oxidative and endoplasmic reticulum stress-mediated apoptosis, Neurochem. Int., 2014, 78, 43–52 CrossRef CAS PubMed.
- F. Wu, H. Li, L. Zhao, X. Li, J. You, Q. Jiang, S. Li, L. Jin and Y. Xu, Protective effects of aqueous extract from Acanthopanax senticosus against corticosterone-induced neurotoxicity in PC12 cells, J. Ethnopharmacol., 2013, 148, 861–868 CrossRef PubMed.
- A. Nitta, W. H. Zheng and R. Quirion, Insulin-like growth factor 1 prevents neuronal cell death induced by corticosterone through activation of the PI3k/Akt pathway, J. Neurosci. Res., 2004, 76, 98–103 CrossRef CAS PubMed.
- Z. Y. Li, Z. Guo, Y. M. Liu, X. M. Liu, Q. Chang, Y. H. Liao and R. L. Pan, Neuroprotective effects of Total Saikosaponins of Bupleurum yinchowense on corticosterone-induced apoptosis in PC12 cells, J. Ethnopharmacol., 2013, 148, 794–803 CrossRef CAS PubMed.
- H. E. Flores, T. S. Walker, R. L. Guimarães, H. P. Bais and J. M. Vivanco, Andean root and tuber crops: Underground rainbows, HortScience, 2003, 38, 161–167 Search PubMed.
- Y. Wang, Y. Wang, B. McNeil and L. M. Harvey, Maca: An Andean crop with multi-pharmacological functions, Food Res. Int., 2007, 40, 783–792 CrossRef.
- G. F. Gonzales, Ethnobiology and Ethnopharmacology of Lepidium meyenii (Maca), a Plant from the Peruvian Highlands, J. Evidence-Based Complementary Altern. Med., 2012, 2012, 193496 Search PubMed.
- S. Zha, Q. Zhao, J. Chen, L. Wang, G. Zhang, H. Zhang and B. Zhao, Extraction, purification and antioxidant activities of the polysaccharides from maca (Lepidium meyenii), Carbohydr. Polym., 2014, 111, 584–587 CrossRef CAS PubMed.
- E. H. Choi, J. I. Kang, J. Y. Cho, S. H. Lee, T. S. Kim, I. H. Yeo and H. S. Chun, Supplementation of standardized lipid-soluble extract from maca (Lepidium meyenii) increases swimming endurance capacity in rats, J. Funct. Foods, 2012, 4, 568–573 CrossRef CAS.
- Y. Zhang, L. Yu, M. Ao and W. Jin, Effect of ethanol extract of Lepidium meyenii Walp. on osteoporosis in ovariectomized rat, J. Ethnopharmacol., 2006, 105, 274–279 CrossRef PubMed.
- C. Gonzales, J. Rubio, M. Gasco, J. Nieto, S. Yucra and G. F. Gonzales, Effect of short-term and long-term treatments with three ecotypes of Lepidium meyenii (MACA) on spermatogenesis in rats, J. Ethnopharmacol., 2006, 103, 448–454 CrossRef PubMed.
- M. S. Lee, B. C. Shin, E. J. Yang, H. J. Lim and E. Ernst, Maca (Lepidium meyenii) for treatment of menopausal symptoms: a systematic review, Maturitas, 2011, 70, 227–233 CrossRef PubMed.
- Z. Ai, A. F. Cheng, Y. T. Yu, L. J. Yu and W. Jin, Antidepressant-like behavioral, anatomical, and biochemical effects of petroleum ether extract from maca (Lepidium meyenii) in mice exposed to chronic unpredictable mild stress, J. Med. Food, 2014, 17, 535–542 CrossRef CAS PubMed.
- J. Rubio, M. Caldas, S. Dávila, M. Gasco and G. F. Gonzales, Effect of three different cultivars of Lepidium meyenii (Maca) on learning and depression in ovariectomized mice, BMC Complementary Altern. Med., 2006, 6, 23 CrossRef PubMed.
- L. Stojanovska, C. Law, B. Lai, T. Chung, K. Nelson, S. Day, V. Apostolopoulos and C. Haines, Maca reduces blood pressure and depression, in a pilot study in postmenopausal women, Climacteric, 2015, 18, 69–78 CrossRef CAS PubMed.
- S. S. Guo, X. F. Gao, Y. R. Gu, Z. X. Wan, A. M. Lu, Z. H. Qin and L. Luo, Preservation of Cognitive Function by Lepidium meyenii (Maca) Is Associated with Improvement of Mitochondrial Activity and Upregulation of Autophagy-Related Proteins in Middle-Aged Mouse Cortex, J. Evidence-Based Complementary Altern. Med., 2016, 2016, 4394261 Search PubMed.
- J. Rubio, H. Dang, M. Gong, X. Liu, S. L. Chen and G. F. Gonzales, Aqueous and hydroalcoholic extracts of Black Maca (Lepidium meyenii) improve scopolamine-induced memory impairment in mice, Food Chem. Toxicol., 2007, 45, 1882–1890 CrossRef CAS PubMed.
- J. Rubio, S. Yucra, M. Gasco and G. F. Gonzales, Dose–response effect of black maca (Lepidium meyenii) in mice with memory impairment induced by ethanol, Toxicol. Mech. Methods, 2011, 21, 628–634 CrossRef CAS PubMed.
- J. Rubio, W. Qiong, X. Liu, Z. Jiang, H. Dang, S. L. Chen and G. F. Gonzales, Aqueous extract of black maca (Lepidium meyenii) on memory impairment induced by ovariectomy in mice, J. Evidence-Based Complementary Altern. Med., 2008, 2011, 253958 Search PubMed.
- A. Pino-Figueroa, D. Nguyen and T. J. Maher, Neuroprotective effects of Lepidium meyenii (Maca), Ann. N. Y. Acad. Sci., 2010, 1199, 77–85 CrossRef PubMed.
- A. Pino-Figueroa, H. Vu, C. J. Kelley and T. J. Maher, Mechanism of Action of Lepidium meyenii (Maca): An Explanation for Its Neuroprotective Activity, Am. J. Neuroprot. Neuroregener., 2011, 3, 87–92 CrossRef.
- M. M. Mccollom, J. R. Villinski, K. L. Mcphail, L. E. Craker and S. Gafner, Analysis of macamides in samples of Maca (Lepidium meyenii) by HPLC-UV-MS/MS, Phytochem. Anal., 2005, 16, 463–469 CrossRef CAS PubMed.
- J. Zhao, I. Muhammad, D. C. Dunbar, J. Mustafa and I. A. Khan, New alkamides from maca (Lepidium meyenii), J. Agric. Food Chem., 2005, 53, 690–693 CrossRef CAS PubMed.
- K. S. Gugnani, N. Vu, A. N. Rondón-Ortiz, M. Böhlke, T. J. Maher and A. J. Pino-Figueroa, Neuroprotective activity of macamides on manganese-induced mitochondrial disruption in U-87 MG glioblastoma cells, Toxicol. Appl. Pharmacol., 2018, 340, 67–76 CrossRef CAS PubMed.
- Q. Yang, W. Jin, X. Lv, P. Dai, Y. Ao, M. Wu, W. Deng and L. Yu, Effects of macamides on endurance capacity and anti-fatigue property in prolonged swimming mice, Pharm. Biol., 2016, 54, 827–834 CrossRef CAS PubMed.
- H. Liu, W. Jin, C. Fu, P. Dai, Y. Yu, Q. Huo and L. Yu, Discovering anti-osteoporosis constituents of maca (Lepidium meyenii) by combined virtual screening and activity verification, Food Res. Int., 2015, 77, 215–220 CrossRef CAS.
- Y. Zhou, P. Li, A. Brantner, H. Wang, X. Shu, J. Yang, N. Si, L. Han, H. Zhao and B. Bian, Chemical profiling analysis of Maca using UHPLC-ESI-Orbitrap MS coupled with UHPLC-ESI-QqQ MS and the neuroprotective study on its active ingredients, Sci. Rep., 2017, 7, 44660 CrossRef PubMed.
- X. Yan, T. Chen, L. Zhang and H. Du, Protective effects of Forsythoside A on amyloid beta-induced apoptosis in PC12 cells by downregulating acetylcholinesterase, Eur. J. Pharmacol., 2017, 810, 141–148 CrossRef CAS PubMed.
- Z. Hajdu, S. Nicolussi, M. Rau, L. Lorántfy, P. Forgo, J. Hohmann, D. Csupor and J. Gertsch, Identification of endocannabinoid system-modulating N-alkylamides from Heliopsis helianthoides var. scabra and Lepidium meyenii, J. Nat. Prod., 2014, 77, 1663–1669 CrossRef CAS PubMed.
- H. Wu, C. J. Kelley, A. Pino-Figueroa, H. D. Vu and T. J. Maher, Macamides and their synthetic analogs: evaluation of in vitro FAAH inhibition, Bioorg. Med. Chem., 2013, 21, 5188–5197 CrossRef CAS PubMed.
- H. Almukadi, H. Wu, M. Böhlke, C. J. Kelley, T. J. Maher and A. Pino-Figueroa, The macamide N-3-methoxybenzyl-linoleamide is a time-dependent fatty acid amide hydrolase (FAAH) inhibitor, Mol. Neurobiol., 2013, 48, 333–339 CrossRef CAS PubMed.
- J. Hwang, C. Adamson, D. Butler, D. R. Janero, A. Makriyannis and B. A. Bahr, Enhancement of endocannabinoid signaling by fatty acid amide hydrolase inhibition: a neuroprotective therapeutic modality, Life Sci., 2010, 86, 615–623 CrossRef CAS PubMed.
- D. Piomelli, G. Tarzia, A. Duranti, A. Tontini, M. Mor, T. R. Compton, O. Dasse, E. P. Monaghan, J. A. Parrott and D. Putman, Pharmacological profile of the selective FAAH inhibitor KDS-4103 (URB597), CNS Drug Rev., 2006, 12, 21–38 CrossRef CAS PubMed.
- S. H. Su, Y. Q. Wang, Y. F. Wu, D. P. Wang, Q. Lin and J. Hai, Cannabinoid receptor agonist win55,212-2 and fatty acid amide hydrolase inhibitor URB597 may protect against cognitive impairment in rats of chronic cerebral hypoperfusion via PI3K/AKT signaling, Behav. Brain Res., 2016, 313, 334–344 CrossRef CAS PubMed.
- S. H. Su, Y. F. Wu, Q. Lin, F. Yu and J. Hai, Cannabinoid receptor agonist win55,212-2 and fatty acid amide hydrolase inhibitor URB597 suppress chronic cerebral hypoperfusion-induced neuronal apoptosis by inhibiting c-Jun N-terminal kinase signaling, Neuroscience, 2015, 301, 563–575 CrossRef CAS PubMed.
- L. R. Vilela, P. H. Gobira, T. G. Viana, D. C. Medeiros, T. H. Ferreira-Vieira, J. G. Doria, F. Rodrigues, D. C. Aguiar, G. S. Pereira, A. R. Massessini, F. M. Ribeiro, A. C. P. de Oliveira, M. F. D. Moraes and F. A. Moreira, Enhancement of endocannabinoid signaling protects against cocaine-induced neurotoxicity, Toxicol. Appl. Pharmacol., 2015, 286, 178–187 CrossRef CAS PubMed.
- B. Zeng, Y. Li, B. Niu, X. Wang, Y. Cheng, Z. Zhou, T. You, Y. Liu, H. Wang and J. Xu, Involvement of PI3K/Akt/FoxO3a and PKA/CREB Signaling Pathways in the Protective Effect of Fluoxetine Against Corticosterone-Induced Cytotoxicity in PC12 Cells, J. Mol. Neurosci., 2016, 59, 567–578 CrossRef CAS PubMed.
- Z. Zeng, X. Wang, S. K. Bhardwaj, X. Zhou, P. J. Little, R. Quirion, L. K. Srivastava and W. Zheng, The Atypical Antipsychotic Agent, Clozapine, Protects Against Corticosterone-Induced Death of PC12 Cells by Regulating the Akt/FoxO3a Signaling Pathway, Mol. Neurobiol., 2017, 54, 3395–3406 CrossRef CAS PubMed.
- H. A. Elgebaly, N. M. Mosa, M. Allach, K. F. El-Massry, A. H. El-Ghorab, A. M. Al Hroob and A. M. Mahmoud, Olive oil and leaf extract prevent fluoxetine-induced hepatotoxicity by attenuating oxidative stress, inflammation and apoptosis, Biomed. Pharmacother., 2018, 98, 446–453 CrossRef CAS PubMed.
- I. Reznik, L. Volchek, R. Mester, M. Kotler, I. Sarova-Pinhas, B. Spivak and A. Weizman, Myotoxicity and Neurotoxicity during Clozapine Treatment, Clin. Neuropharmacol., 2000, 23, 276–280 CrossRef CAS PubMed.
- A. Goto, A. Mouri, T. Nagai, A. Yoshimi, M. Ukigai, T. Tsubai, H. Hida, N. Ozaki and Y. Noda, Involvement of the histamine H4 receptor in clozapine-induced hematopoietic toxicity: Vulnerability under granulocytic differentiation of HL-60 cells, Toxicol. Appl. Pharmacol., 2016, 306, 8–16 CrossRef CAS PubMed.
- Y. Jiang, Z. Li, Y. Liu, X. Liu, Q. Chang, Y. Liao and R. Pan, Neuroprotective effect of water extract of Panax ginseng on corticosterone-induced apoptosis in PC12 cells and its underlying molecule mechanisms, J. Ethnopharmacol., 2015, 159, 102–112 CrossRef CAS PubMed.
- L. J. McIntosh and R. M. Sapolsky, Glucocorticoids Increase the Accumulation of Reactive Oxygen Species and Enhance Adriamycin-Induced Toxicity in Neuronal Culture, Exp. Neurol., 1996, 141, 201–206 CrossRef CAS PubMed.
- R. M. Sapolsky, D. R. Packan and W. W. Vale, Glucocorticoid toxicity in the hippocampus: in vitro demonstration, Brain Res., 1988, 453, 367–371 CrossRef CAS PubMed.
- Y. Z. Zhou, X. Li, W. X. Gong, J. S. Tian, X. X. Gao, L. Gao, X. Zhang, G. H. Du and X. M. Qin, Protective effect of isoliquiritin against corticosterone-induced neurotoxicity in PC12 cells, Food Funct., 2017, 8, 1235–1244 RSC.
- H. Kim, K. Youn, E. Y. Yun, J. S. Hwang, W. S. Jeong, C. T. Ho and M. Jun, Oleic acid ameliorates Aβ-induced inflammation by downregulation of COX-2 and iNOS via NFκB signaling pathway, J. Funct. Foods, 2015, 14, 1–11 CrossRef CAS.
- A. Y. Lee, M. H. Lee, S. Lee and E. J. Cho, Neuroprotective effect of alpha-linolenic acid against Aβ-mediated inflammatory responses in C6 glial cell, J. Agric. Food Chem., 2018, 66, 4853–4861 CrossRef CAS PubMed.
- T. A. Kosten, M. P. Galloway, R. S. Duman, D. S. Russell and C. D'Sa, Repeated Unpredictable Stress and Antidepressants Differentially Regulate Expression of the Bcl-2 Family of Apoptotic Genes in Rat Cortical, Hippocampal, and Limbic Brain Structures, Neuropsychopharmacology, 2007, 33, 1545–1558 CrossRef PubMed.
- J. Yang, X. Liu, K. Bhalla, C. N. Kim, A. M. Ibrado, J. Cai, T. I. Peng, D. P. Jones and X. Wang, Prevention of Apoptosis by Bcl-2: Release of Cytochrome c from Mitochondria Blocked, Science, 1997, 275, 1129–1132 CrossRef CAS PubMed.
- R. M. Kluck, E. Bossy-Wetzel, D. R. Green and D. D. Newmeyer, The Release of Cytochrome c from Mitochondria: A Primary Site for Bcl-2 Regulation of Apoptosis, Science, 1997, 275, 1132–1136 CrossRef CAS PubMed.
- P. J. Lucassen, M. B. Müller, F. Holsboer, J. Bauer, A. Holtrop, J. Wouda, W. J. G. Hoogendijk, E. R. D. Kloet and D. F. Swaab, Hippocampal apoptosis in major depression is a minor event and absent from subareas at risk for glucocorticoid overexposure, Am. J. Pathol., 2001, 158, 453–468 CrossRef CAS PubMed.
- H. Dudek, S. R. Datta, T. F. Franke, M. J. Birnbaum, R. Yao, G. M. Cooper, R. A. Segal, D. R. Kaplan and M. E. Greenberg, Regulation of Neuronal Survival by the Serine-Threonine Protein Kinase Akt, Science, 1997, 275, 661–665 CrossRef CAS PubMed.
- R. Ma, N. Xiong, C. Huang, Q. Tang, B. Hu, J. Xiang and G. Li, Erythropoietin protects PC12 cells from β-amyloid25–35-induced apoptosis via PI3K/Akt signaling pathway, Neuropharmacology, 2009, 56, 1027–1034 CrossRef CAS PubMed.
- Y. Q. Xu, L. Long, J. Q. Yan, L. Wei, M. Q. Pan, H. M. Gao, P. Zhou, M. Liu, C. S. Zhu, B. S. Tang and Q. Wang, Simvastatin induces neuroprotection in 6-OHDA-lesioned PC12 via the PI3K/Akt/caspase 3 pathway and anti-inflammatory responses, CNS Neurosci. Ther., 2013, 19, 170–177 CrossRef CAS PubMed.
- W. Zhang, R. Wang, S. F. Han, L. Bu, S. W. Wang, H. Ma and G. L. Jia, α-Linolenic acid attenuates high glucose-induced apoptosis in cultured human umbilical vein endothelial cells via PI3K/Akt/eNOS pathway, Nutrition, 2007, 23, 762–770 CrossRef CAS PubMed.
- H. Gao, P. Yan, S. Zhang, H. Huang, F. Huang, T. Sun, Q. Deng, Q. Huang, S. Chen, K. Ye, J. Xu and L. Liu, Long-Term Dietary Alpha-Linolenic Acid Supplement Alleviates Cognitive Impairment Correlate with Activating Hippocampal CREB Signaling in Natural Aging Rats, Mol. Neurobiol., 2016, 53, 4772–4786 CrossRef CAS PubMed.
- S. Finkbeiner, CREB couples neurotrophin signals to survival messages, Neuron, 2000, 25, 11–14 CrossRef CAS.
- Y. Wang, J. Zhang, M. Han, B. Liu, Y. Gao, P. Ma, S. Zhang, Q. Zheng and X. Song, SMND-309 promotes neuron survival through the activation of the PI3K/Akt/CREB-signalling pathway, Pharm. Biol., 2016, 54, 1982–1990 CrossRef CAS PubMed.
Footnote |
† Electronic supplementary information (ESI) available. See DOI: 10.1039/c9ra03268a |
|
This journal is © The Royal Society of Chemistry 2019 |