DOI:
10.1039/C9RA01071H
(Paper)
RSC Adv., 2019,
9, 9093-9098
A mild and efficient method for the synthesis of pyrroles using MIL-53(Al) as a catalyst under solvent-free sonication†
Received
10th February 2019
, Accepted 11th March 2019
First published on 19th March 2019
Abstract
A highly efficient method for the synthesis of pyrroles using MIL-53(Al) as a catalyst has been developed under solvent-free sonication. This reaction has a broad substrate scope and high yields were obtained within a short reaction time. Remarkably, no additional additives and volatile organic solvent are required for this method and the MIL-53(Al) could be recovered and reused several times without significant drop-off in catalytic activity.
Introduction
Metal–organic frameworks (MOFs) are a class of crystalline porous materials containing metal clusters (termed secondary building units, SBUs) connected by a variety of organic bridges.1,2 These materials have attracted much interest for many potential applications in heterogeneous catalysis due to their exceptional properties including crystallinity, active-site uniformity, and high porosity with permeable channels.3–7 Especially, aluminum based-MOFs with highly robust structures have recently emerged as promising catalysts in several organic transformations.8,9 Among them, the well-known catalyst is MIL-53(Al), whose porous 3D structure with 1D lozenge-shaped channels (Fig. 1) is constructed from a connection of infinite trans-chains of corner-sharing (via OH groups) AlO4(OH)2 octahedra with ditopic organic linker, 1,4-benzenedicarboxylate (BDC).10 Because of the exceptional structural properties, the material could play a role as a Brønsted-type11,12 (OH sites) and Lewis-type13 (Al3+ sites) acid heterogeneous catalyst. Although there have been a wide number of reported researches used MIL-53(Al) for catalysis in alkylation,13 cycloaddition,14 Mannich reaction,12 and cellulose transformation,11 the catalytic activity of MIL-53(Al) for organic transformations remains an attractive field.
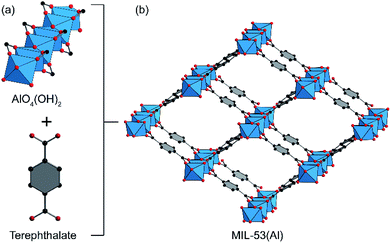 |
| Fig. 1 The combination of an infinite octahedral trans-chain metal building unit and ditopic linker (a) to form the porous 3D structure with 1D rhombic channels (b). Atom colors: C, black; O, red; Al, blue; H atoms are omitted for clarity. | |
Pyrroles are important structural motifs present in various biologically active molecules and natural products.15–23 There are three commonly used approaches for the synthesis of these essential compounds including Barton–Zard, Paal–Knorr, and Hantzsch syntheses. Among these approaches, the Paal–Knorr reaction is known as an extremely efficient pathway to produce pyrroles from primary amines and 1,4-dicarbonyl compounds under acidic conditions. Over the past decade, several typical acidic catalysts have been widely employed in the Paal–Knorr reaction including Lewis acids,24–28 metal–organic frameworks,29 deep eutectic solvents,30 Brønsted acids.31,32 Despite the potential utility of these catalysts, many of them still suffer from certain drawbacks, including long reaction times, volatile organic solvents, low yields, expensive and unrecyclable catalysts. Due to the advantages of high activity and easy recovery, heterogeneous catalysts have been receiving much attention in the organic synthesis. Herein, we describe the direct synthesis of pyrrole using primary amines and hexa-1,4-dione catalyzed by MIL-53(Al) under solvent-free sonication. The mild reaction conditions, no additional additives, work-up simplicity, catalytic recyclability are the outstanding features of the present work.
Experimental
General methods
Powder X-ray diffraction (PXRD) patterns were recorded using a D8 Advance diffractometer equipped with a LYNXEYE detector (Bragg–Brentano geometry, Cu Kα radiation λ = 1.54056 Å). Thermal gravimetric analysis (TGA) was performed using a TA Instruments Q-500 thermal gravimetric analyzer under airflow with a temperature ramp of 5 °C min−1. Fourier transform infrared (FT-IR) spectra were measured on a Bruker E400 FT-IR spectrometer using potassium bromide pellets. Low-pressure N2 and CO2 adsorption measurements were carried out on a Quantachrome Autosorb iQ volumetric gas adsorption analyzer. A liquid N2 bath was used for measurements at 77 K. Helium was used as an estimation of dead space. Ultrahigh-purity-grade N2, and He (99.999% purity) were used throughout adsorption experiments. Solution NMR spectra were recorded on a Bruker Advance-500 MHz NMR spectrometer. Scanning Electron Microscopy (SEM) images were performed on a JEOL JSM-75FCT. Gas chromatography (GC) analyses were performed using Agilent 6890 Series equipped with a flame ionization detector (FID) and a DB-5 column (length = 30 m, inner diameter = 320 μm, film thickness = 0.25 μm). GC-MS analyses were performed on Agilent GC System 7890 Series, equipped with a mass selective detector Agilent 5973N and a capillary DB-5MS column (length = 30 m, inner diameter = 320 μm, film thickness = 0.25 μm).
Preparation of MIL-53(Al) catalyst
MIL-53(Al) was hydrothermally synthesized following the reported procedure.33 Al(NO3)3·9H2O (375 mg, 0.50 mmol) and 1,4-benzenedicarboxylic acid, H2BDC, (83.1 mg, 0.50 mmol) were dissolved in 1.5 mL deionized (DI) water in a Teflon-lined steel autoclave. The solution was subsequently heated at 220 °C for 3 days in an isothermal oven to yield a white precipitate. After cooling the autoclave to room temperature, the white precipitate was obtained by centrifugation and washed five times with DI water. The as-synthesized sample was then evacuated at room temperature for 24 h. The solid was then heated in air at 330 °C for 3 days for removal of the excessive H2BDC adsorbed in the pores.
General procedure for Paal–Knorr reaction
MIL-53(Al) (10 mg, 5 mol%), aromatic amine (1.0 mmol), actonylacetone (1.2 mmol) was added into a 10 mL pressurized glass tube. The reaction mixture was sonicated at 80 °C for an appropriate time. The progress of the reaction was monitored by TLC and GC. After completion of the reaction, the catalyst was filtered from the reaction mixture. The filtrate was diluted with ethyl acetate (25 mL), washed with H2O (5 × 15 mL), and dried over Na2SO4. The solvent was removed under vacuum on a rotary evaporator. The pure product was isolated by flash chromatography (n-hexane, then 10% ethyl acetate in n-hexane). The purity and identity of the pyrrole were confirmed by GC-MS spectrometry, 1H and 13C NMR spectroscopy.
Results and discussion
The MIL-53(Al) was prepared by a hydrothermal reaction of aluminum chloride and the ditopic linker, 1,4-benzenedicarboxylic acid. After the reaction finished, the suspension was cooled to room temperature, and then the white precipitate was obtained by centrifugation and washed five times with DI water. As-synthesized MIL-53 was heated at 330 °C for 3 days and activated at 120 °C for a day to yield the guest-free material. After activation, the purity of MIL-53(Al) framework was determined by powder X-ray diffraction (PXRD) in which the activated PXRD pattern for MIL-53(Al) was in good agreement to that of the simulated MIL-53(Al) (ESI, Fig. S1†).10 Thermogravimetric analysis (TGA) was utilized to access the activation procedure. The TGA curve of the activated sample with a single step of the linker decomposition above 500 °C demonstrated the complete removal of guest molecules in the framework (ESI, Fig. S2†). The nitrogen adsorption isotherm of MIL-53(Al) at low pressure and 77 K exhibited a type-I behavior due to the presence of micropore within the structure and an internal surface area of 1120 m2 g−1 based on Brunauer–Emmett–Teller (BET) method (ESI, Fig. S3†).
Subsequently, we employed MIL-53(Al) directly to synthesize pyrroles selectively and the desired product was afforded in 96% yield (Table 1, entry 18). To evaluate the efficiency of catalyst, the model reaction of acetonylacetone with aniline was carried out under solvent-free sonication in the presence of various catalysts.
Table 1 The effect of different catalysts in the Paal–Knorr reactiona

|
Entry |
Catalyst |
Isolated yield (%) |
Reaction condition: aniline (1.0 mmol), acetonylacetone (1.2 mmol) in the presence of the catalyst (5 mol%) under solvent-free sonication. |
1 |
CuCl2 |
67 |
2 |
FeCl2 |
76 |
3 |
HfCl4 |
83 |
4 |
FeCl3 |
78 |
5 |
AlCl3 |
83 |
6 |
ZnCl2 |
77 |
7 |
Fe2O3 |
74 |
8 |
ZnO |
70 |
9 |
Al2O3 |
55 |
10 |
TiO2 |
73 |
11 |
MgO |
14 |
12 |
CuO |
72 |
13 |
MOF-890 |
80 |
14 |
MOF-891 |
82 |
15 |
MOF-177 |
81 |
16 |
ZIF-8 |
83 |
17 |
HKUST-1 |
89 |
18 |
MIL-53(Al) |
96 |
19 |
Non-catalyst |
25 |
As can be seen in Table 1, the corresponding product was afforded in a good yield of 67–83% in the presence of metal halides (Table 1, entries 1–6), while low yields were observed when metal oxides were employed (Table 1, entries 7–12). HfCl4 and AlCl3 provided the corresponding product in high yields, but these metal halides were not recovered after the aqueous work-up. Then, MOFs including MOF-890, MOF-891, MOF-177, ZIF-8, and HKUST-1 were tested in the model reaction and good yields of 80–89% were observed (Table 1, entries 13–17). MIL-53(Al) displayed the best catalytic activity and high stability for the Paal–Knorr synthesis (Table 1, entry 18). However, the reaction could hardly proceed in the absence of a catalyst (Table 1, entry 19). According to these results, we reason that MIL-53(Al) acted as an efficient heterogeneous Lewis acid catalyst in this reaction (as shown in Scheme S1†). Thus, the reaction conditions were optimized in the presence of MIL-53(Al) catalyst under solvent-free sonication (see Table S1–S4 in the ESI†).
With the optimized conditions in hand, the scope of was investigated (Table 2). The electronic properties of aromatic amines have little influence on the Paal–Knorr synthesis. Reactions with anilines bearing dihalo groups (Table 2, entries 2–5) could provide the desired products in good yields (>80%) with prolonged the reaction times (20–30 min). Interestingly, the yield of expected product increased to 96% when 4-iodoaniline (Table 2, entry 6) was employed as the substrate. As expected, reactions with anilines bearing electron-donating groups in the aromatic ring (Table 2, entries 7–10) could effectively deliver the corresponding products in excellent yields (>90%) with 100% conversion of substrates. Interestingly, when 4-nitro-o-phenylenediamine was employed as the substrate and the yield of major product was obtained in 75% (Table 2, entry 11). Remarkably, after the reaction mixtures were exposed to ultrasound for 15–20 min, anilines bearing electron-withdrawing groups can be converted into the corresponding products (Table 2, entry 12–15). The expected products were afforded in moderate yields with phenylhydrazine (Table 2, entry 16). No product was detected with 2,4-dinitrophenylhydrazine (Table 2, entry 17). As expected, the use of 1,2-ethanediamine as the substrate provided the corresponding bispyrrole in 55% yield; attempts to improve the reaction yield by using the molar of acetonylacetone to greater than two equiv. were successful (Table 2, entry 18). Furthermore, the current protocol was also investigated on a 10 mmol scale reaction between aniline and acetonylacetone, which provided the corresponding product in 95% yield. Consequently, the present method can be potentially applied to the industrial processes.
Table 2 Synthesis of pyrroles catalyzed by MIL-53(Al)a
Entry |
Amine |
Time (min) |
Product |
Isolated yieldb (%) |
The reaction conditions: amines (1 mmol), acetonylacetone (1.2 mmol). Isolated yield. Yield of another isomer was obtained in 15%. Yield in parenthesis was reported by the reaction with 2.4 equiv. of acetonylacetone. |
1 |
 |
15 |
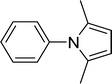 |
96 |
2 |
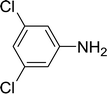 |
20 |
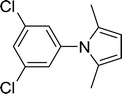 |
86 |
3 |
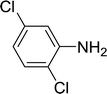 |
30 |
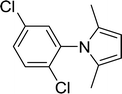 |
85 |
4 |
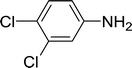 |
30 |
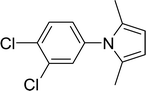 |
88 |
5 |
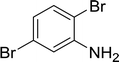 |
30 |
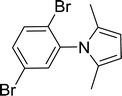 |
80 |
6 |
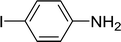 |
15 |
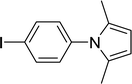 |
96 |
7 |
 |
15 |
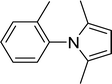 |
98 |
8 |
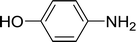 |
15 |
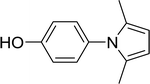 |
95 |
9 |
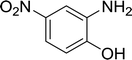 |
15 |
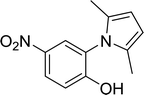 |
98 |
10 |
 |
15 |
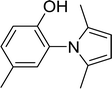 |
98 |
11 |
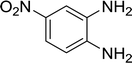 |
15 |
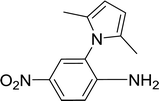 |
75c |
12 |
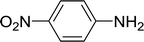 |
20 |
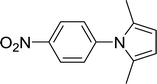 |
90 |
13 |
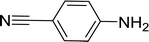 |
15 |
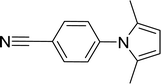 |
98 |
14 |
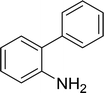 |
15 |
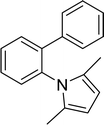 |
95 |
15 |
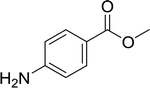 |
15 |
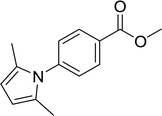 |
98 |
16 |
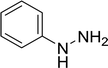 |
15 |
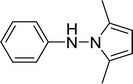 |
50 |
17 |
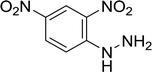 |
20 |
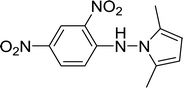 |
Trace |
18 |
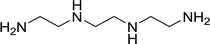 |
15 |
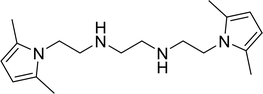 |
55 (92)d |
The recovery and reuse of the developed MIL-53(Al) catalyst in the Paal–Knorr synthesis can be achieved by a simple filtration. A little decrease of the catalytic activity was observed after the fourth cycle (Fig. 2). These results demonstrated that the MIL-53(Al) could be reused at least four times without significant decline of catalytic activity. PXRD analysis of MIL-53(Al) revealed that the crystallinity and integrity of this catalyst was maintained after the fourth cycle (please see Fig. S6 in ESI†).
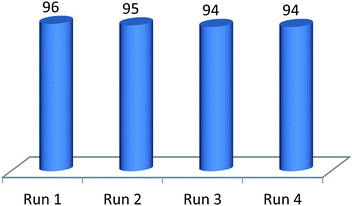 |
| Fig. 2 Recycling of MIL-53(Al). | |
Conclusion
In conclusion, we have developed a highly efficient protocol for cyclocondensation between primary amines and acetonylacetone using cheap and easily-prepared MIL-53(Al) under solvent-free sonication. To the best of our knowledge, this is the first example of Paal–Knorr synthesis catalyzed by a heterogeneous MIL-53(Al) under sonication. It is noteworthy that the additional additives and volatile organic solvents are not required for this method. Moreover, MIL-53(Al) employed in catalytic amount and can be recycled at least four times without significant decrease in its catalytic activity. The detailed mechanistic study is in progress within our laboratory.
Conflicts of interest
There are no conflicts to declare.
Notes and references
- A. Schoedel, M. Li, D. Li, M. O'Keeffe and O. M. Yaghi, Chem. Rev., 2016, 116, 12466–12535 CrossRef CAS PubMed.
- C. Wang, X. Liu, N. Keser Demir, J. P. Chen and K. Li, Chem. Soc. Rev., 2016, 45, 5107–5134 RSC.
- J. Liu, L. Chen, H. Cui, J. Zhang, L. Zhang and C.-Y. Su, Chem. Soc. Rev., 2014, 43, 6011–6061 RSC.
- Z. Hu and D. Zhao, CrystEngComm, 2017, 19, 4066–4081 RSC.
- L. H. T. Nguyen, T. T. Nguyen, H. L. Nguyen, T. L. H. Doan and P. H. Tran, Catal. Sci. Technol., 2017, 7, 4346–4350 RSC.
- H. L. Nguyen, T. T. Vu, D.-K. Nguyen, C. A. Trickett, T. L. H. Doan, C. S. Diercks, V. Q. Nguyen and K. E. Cordova, Communications Chemistry, 2018, 1, 70 CrossRef.
- T. A. To, Y. H. Vo, H. T. T. Nguyen, P. T. M. Ha, S. H. Doan, T. L. H. Doan, S. Li, H. V. Le, T. N. Tu and N. T. S. Phan, J. Catal., 2019, 370, 11–20 CrossRef CAS.
- L. M. Aguirre-Díaz, D. Reinares-Fisac, M. Iglesias, E. Gutiérrez-Puebla, F. Gándara, N. Snejko and M. Á. Monge, Coord. Chem. Rev., 2017, 335, 1–27 CrossRef.
- A. U. Czaja, N. Trukhan and U. Muller, Chem. Soc. Rev., 2009, 38, 1284–1293 RSC.
- T. Loiseau, C. Serre, C. Huguenard, G. Fink, F. Taulelle, M. Henry, T. Bataille and G. Férey, Chem.–Eur. J., 2004, 10, 1373–1382 CrossRef CAS PubMed.
- G. Zi, Z. Yan, Y. Wang, Y. Chen, Y. Guo, F. Yuan, W. Gao, Y. Wang and J. Wang, Carbohydr. Polym., 2015, 115, 146–151 CrossRef CAS PubMed.
- M. Zongcheng, Q. Chao, W. Liangshi, A. M. Wensley and L. Yi, Appl. Organomet. Chem., 2017, 31, e3569 CrossRef.
- E. Rahmani and M. Rahmani, Ind. Eng. Chem. Res., 2018, 57, 169–178 CrossRef CAS.
- R. Li, Y. Jiang, J. Zhao, D. Ramella, Y. Peng and Y. Luan, RSC Adv., 2017, 7, 34591–34597 RSC.
- S. Michlik and R. Kempe, Nat. Chem., 2013, 5, 140–144 CrossRef CAS PubMed.
- B. T. Parr, C. Economou and S. B. Herzon, Nature, 2015, 525, 507–510 CrossRef CAS PubMed.
- X. B. Ding, M. A. Brimble and D. P. Furkert, Org. Biomol. Chem., 2016, 14, 5390–5401 RSC.
- L. Tao, Z.-J. Wang, T.-H. Yan, Y.-M. Liu, H.-Y. He and Y. Cao, ACS Catal., 2016, 959–964, DOI:10.1021/acscatal.6b02953.
- B. Xu, G. Li, J. Li and Y. Shi, Org. Lett., 2016, 18, 2028–2031 CrossRef CAS PubMed.
- M. Fleige and F. Glorius, Chem.–Eur. J., 2017, 23, 10773–10776 CrossRef CAS PubMed.
- Z. Gong, Y. Lei, P. Zhou and Z. Zhang, New J. Chem., 2017, 41, 10613–10618 RSC.
- A. Kornienko and J. J. La Clair, Nat. Prod. Rep., 2017, 34, 1051–1060 RSC.
- L. Zhang, J. Zhang, J. Ma, D.-J. Cheng and B. Tan, J. Am. Chem. Soc., 2017, 139, 1714–1717 CrossRef CAS PubMed.
- A. Rahmatpour, Appl. Organomet. Chem., 2011, 25, 585–590 CrossRef CAS.
- K. Aghapoor, L. Ebadi-Nia, F. Mohsenzadeh, M. Mohebi Morad, Y. Balavar and H. R. Darabi, J. Organomet. Chem., 2012, 708–709, 25–30 CrossRef CAS.
- A. A. Jafari and H. Mahmoudi, Environ. Chem. Lett., 2012, 11, 157–162 CrossRef.
- A. Rahmatpour, J. Organomet. Chem., 2012, 712, 15–19 CrossRef CAS.
- A. Kamal, S. Faazil, M. Shaheer Malik, M. Balakrishna, S. Bajee, M. R. H. Siddiqui and A. Alarifi, Arabian J. Chem., 2016, 9, 542–549 CrossRef CAS.
- N. T. S. Phan, T. T. Nguyen, Q. H. Luu and L. T. L. Nguyen, J. Mol. Catal. A: Chem., 2012, 363–364, 178–185 CrossRef CAS.
- S. Handy and K. Lavender, Tetrahedron Lett., 2013, 54, 4377–4379 CrossRef CAS.
- L. Akelis, J. Rousseau, R. Juskenas, J. Dodonova, C. Rousseau, S. Menuel, D. Prevost, S. Tumkevičius, E. Monflier and F. Hapiot, Eur. J. Org. Chem., 2016, 2016, 31–35 CrossRef CAS.
- F. Bonyasi, M. Hekmati and H. Veisi, J. Colloid Interface Sci., 2017, 496, 177–187 CrossRef CAS PubMed.
- X. Qian, B. Yadian, R. Wu, Y. Long, K. Zhou, B. Zhu and Y. Huang, Int. J. Hydrogen Energy, 2013, 38, 16710–16715 CrossRef CAS.
Footnote |
† Electronic supplementary information (ESI) available. See DOI: 10.1039/c9ra01071h |
|
This journal is © The Royal Society of Chemistry 2019 |