DOI:
10.1039/C8RA09851D
(Paper)
RSC Adv., 2019,
9, 2645-2649
Strain-effected physical properties of ferromagnetic insulating La0.88Sr0.12MnO3 thin films
Received
30th November 2018
, Accepted 11th January 2019
First published on 21st January 2019
Abstract
The functional perovskite La1−xSrxMnO3 (LSMO) possesses various exotic phases owing to competing physical parameters and internal degrees of freedom. In particular, the nature of the ferromagnetic insulating phase (FMI) has not been adequately explored, resulting in a limited understanding of the relationship between crystal structure and magnetism. To investigate this structure–property relationship, epitaxial La0.88Sr0.12MnO3 thin films were grown on two different substrates, (001) SrTiO3 and (001) (LaAlO3)0.3(Sr2AlTaO6)0.7, by pulsed laser deposition. Element-specific and surface-sensitive techniques were applied in conjunction with bulk magnetometry to investigate the inextricable link between the structures and magnetic properties of the films and the effects of tuning the strain. The results unambiguously demonstrate that structure–property relationship of a FMI LSMO tuned by strain has a crucial role for manipulating the properties in the FMI regime.
Introduction
La1−xSrxMnO3 (LSMO) has attracted considerable attention over recent years due to not only its exotic chemical and physical properties in various complex phases but also its great potential for a variety of applications. LSMO is widely regarded as a useful material in practical areas such as spintronics and energy device materials because the perovskite manganite structure results in the colossal magnetoresistance (CMR) effect and half-metal properties with potential application to magnetic memory devices.1,2 Furthermore, some hole-doped LSMO materials exhibit high Curie temperatures (Tc) above room temperature.3 LSMO also possesses a high conductivity and good thermal expansion coefficient, making it a suitable cathode material for commercial solid oxide fuel cells (SOFCs).4,5 In addition, the Jahn–Teller (JT) distortion forms polarons above the Tc (≈370 K), as changing the electronic and atomic structures is associated with the conductivity of materials.6 With respect to physical properties, the complex phases of hole-doped manganites have numerous different ground states caused by competing internal degrees of freedom and applied parameters such as temperature, electric field, magnetic field, and strain.7,8 The exotic phases include various magnetic and electronic phases, a hole-doping metal–insulator transition, and a Griffiths phase.3,9,10
LSMO has complex electronic and magnetic ground states such as the paramagnetic insulating (PMI) phase, ferromagnetic metallic (FMM) phase, and ferromagnetic insulating (FMI) phase.11,12 In particular, the nature of the FMI phase is not well understood compared with that of the FMM phase, which can be described using the double-exchange model. Furthermore, the relationships between the applied parameters and the resulting magnetic states are not yet well established. To further investigate the FMI phase of La1−xSrxMnO3, the low-doping region (x ≤ 0.15) is interesting since the exotic FMI phase is stabilized under these conditions. Thus, this material represents a very attractive model for exploring the correlation between the crystal structure and magnetism.
In an effort to elucidate the interplay between the crystal structure and magnetism of perovskite oxides, numerous studies have investigated the thickness dependence of the structural, electrical, and magnetic properties of epitaxial thin films such as BiFeO3, LiFe5O8, and La1−xSrxMnO3.13–16 However, the FMI phase of La0.88Sr0.12MnO3 has not been adequately investigated. Few studies were conducted partially with transport measurements.11,17
In this work, we report on the exotic magnetic properties caused by substrate-effected strain from two distinct substrates with various experimental methods. Since the applied parameters can be conveniently used to tune the physical properties such as crystallographic structure and magnetism of epitaxial LSMO thin films,6,7 we performed structural characterization, X-ray spectroscopy, and magnetic measurements to observe the effects of substrate-effected strain on the films.
Experimental
Sample preparation
Lightly doped manganite La0.88Sr0.12MnO3 belongs to the space group Pbnm and its lattice parameters are a = 5.5425 Å, b = 5.5346 Å, and c = 7.7857 Å.18 We grew epitaxial thin films of (001) orthorhombic La0.88Sr0.12MnO3 on cubic (001) SrTiO3 (STO, a = 3.905 Å) and (LaAlO3)0.3(Sr2AlTaO6)0.7 (LSAT, a = 3.868 Å) single-crystal substrates by pulsed laser deposition (Q-switched pulsed Nd:YAG laser with λ = 355 nm). The laser fluence was 0.62 J cm−2. We used a La0.88Sr0.12MnO3 target from Toshima, Japan. The growth conditions were 600 °C at 100 mTorr of an oxygen partial pressure. We also optimized the growth process by using a 300 Torr oxygen partial pressure to remove oxygen vacancies upon cooling.
Characterization of thin films
To examine the crystal structural properties of the epitaxial LSMO thin films deposited on single-crystal STO and LSAT substrates, we performed X-ray reflectivity (XRR) and X-ray diffraction (XRD) measurements with a wavelength (λ) of 1.54 Å using a high-resolution X-ray diffractometer (HR-XRD, SmartLab, Rigaku). Fig. 1(a) and (b) show the XRR patterns for the epitaxial LSMO/STO and LSMO/LSAT films, respectively. The results revealed a well-defined film thickness of 17 nm on STO and 18 nm on LSAT, allowing a reasonable comparison to be made between the two films owing to their similar thickness. Fig. 1(c) and (d) show the XRD patterns, which clearly demonstrated that the films were epitaxial and free from impurities. Since the crystal structure of LSMO is orthorhombic, based on a pseudocubic model, the lattice constant for an LSMO thin film is 3.916 Å. As the lattice constants of the substrates are 3.905 Å for STO and 3.868 Å for LSAT, the lattice mismatches were −0.28% for LSMO/STO and −1.24% for LSMO/LSAT, where the negative sign indicates compressive strain.
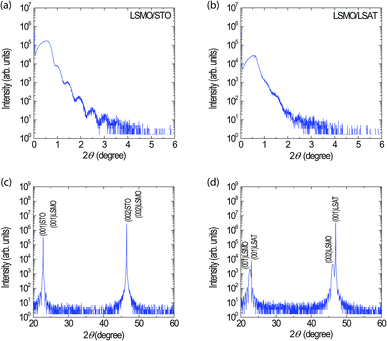 |
| Fig. 1 XRR patterns of the epitaxial thin films of (a) LSMO/STO and (b) LSMO/LSAT. θ–2θ XRD patterns of the epitaxial thin films of (c) LSMO/STO and (d) LSMO/LSAT. These results confirm the successful growth of uniform thin films. | |
To confirm the existence of substrate-effected strain in the LSMO films, we used the reciprocal space mapping (RSM) method. The RSM measurements were performed for the (103) STO and (103) LSAT substrates. Fig. 2(a) and (b) show the corresponding RSM images. The results clearly confirmed that the epitaxial LSMO films exhibited the same in-plane lattice constants for each substrate, indicating the presence of compressive substrate-effected strain in the epitaxial thin films.
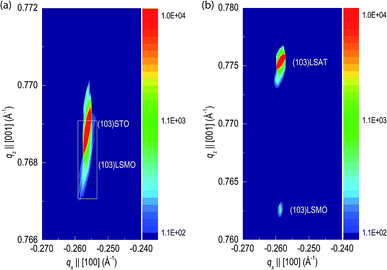 |
| Fig. 2 RSM images for the (a) (103) STO substrate and (b) (103) LSAT substrate. The square box in (a) is a guide to the eye for LSMO. These contour maps confirm the presence of strain in the films. | |
Results and discussion
The temperature and field dependences of the magnetization were measured using a superconducting quantum interference device (SQUID) magnetometer. We used an applied magnetic field of 100 Oe and field cooling to clearly observe the Tc in the temperature dependence of magnetization (M–T) curves for the epitaxial LSMO/LSAT and LSMO/STO thin films. The applied magnetic field is perpendicularly to the plane of thin films. Fig. 3(a) shows that the Tc values were 170 K and 190 K for the LSMO/LSAT and LSMO/STO films, respectively. It should be noted that an anomaly of magnetization was observed for the LSMO/LSAT film, in that the magnetization initially increased up to ∼106 K and thereafter decreased. The low temperature behavior of LSMO on LSAT is reminding us of spin glass and/or cluster glass.19 However, in this work, we have focused on biaxial substrate-induced strain effect on magnetism. This indicates that charge-orbital ordering (COO) occurred in this film.20 Since the in-plane compressive strain reduces the bond distance of Mn3+–O2−–Mn4+ by stretching the MnO6 octahedra in the out-of-plane direction, the value of Tc decreases owing to the stronger double exchange.21 Furthermore, elongation of the MnO6 octahedra also stimulate the competition of the internal degrees of freedom and shift the Tc to a lower temperature. This is caused by the stabilization of the d3z2–r2 with reduced ferromagnetic interaction in the ab plane since Tc links inextricably to delocalize the eg orbitals.17,21
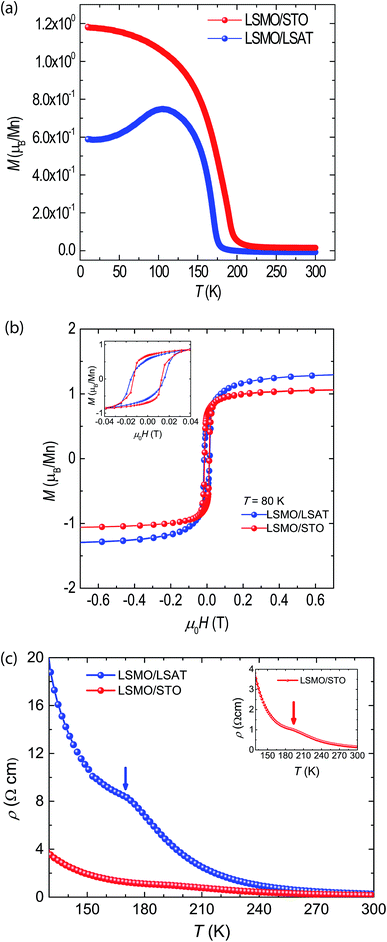 |
| Fig. 3 (a) Magnetization curves as a function of temperature, obtained at an applied magnetic field of 100 Oe. COO occurred in the epitaxial LSMO/LSAT thin film, as revealed by the anomalous increase in magnetization up to ∼106 K and subsequent decrease, which was not observed for the epitaxial LSMO/STO thin film. (b) Magnetic hysteresis loops of the LSMO films under different in-plane compressive strains at 80 K. The magnetization of LSMO/LSAT is greater than that of LSMO/STO, which implies that increased compressive strain reduces the magnetization. (c) Resistivity vs. temperature curves of LSMO thin films. | |
Fig. 3(b) shows the magnetization as a function of the applied field (M–H hysteresis loops), which was measured at 80 K in the fully FMI phase for both substrates below their Tc. These loops revealed distinct differences in the saturated magnetic moments (Ms) of the two films. The magnetization of the epitaxial LSMO thin film grown on STO was smaller than that of the LSMO film grown on LSAT. This implies that a greater compressive strain leads to a higher Ms. This means that the in-plane compressive strain can control magnetic properties. It should also be noted that, as shown in the inset of Fig. 3(b), the coercive field was higher for LSMO/LSAT than for LSMO/STO. This may also be the result of the complex competition between the COO and JT interactions.
We also measured resistivity as a function of temperature as shown Fig. 3(c). These curves indicate that the thin films show indeed insulator behavior. Also, note that there are little humps, pointed by arrows, around 170 K on LSMO/LSAT and 190 K around on LSMO/STO. These present that there is the ferromagnetic phase transition which agrees well to the result of magnetization measurements in terms of temperature shown in Fig. 3(a).
To examine the influence of the substrate-effected strain on the magnetic properties of the films, we performed X-ray magnetic circular dichroism (XMCD) with X-ray absorption spectroscopy (XAS) on the 2A beamline of Pohang Accelerator Laboratory. The XAS and XMCD spectra were obtained in total electron yield mode using an elliptically polarized undulator. XAS and XMCD are very powerful techniques for studying the electronic and magnetic structures of transition-metal materials owing to their element-specific excitation.22,23 XAS provides direct information concerning the valence states of transition-metal materials such as Mn ions, because the core electrons in the 2p orbitals are excited to the 3d orbitals upon absorption.22 XMCD is a unique tool for separately determining the spin and orbital magnetic moments for the element-specific moment.24–26
For these experiments, the base pressure of the experimental chamber was maintained below 5 × 10−10 Torr during the measurements. The applied temperature was 80 K for consistency with the M–H measurements. To obtain the XMCD spectra, an external magnetic field of H ≈ 0.7 T was applied in the out-of-plane direction of the sample. The applied filed direction is the same to the SQUID measurement. The field was flipped to be parallel (μ+) and antiparallel (μ−) to the circularly polarized photon helicity.25,27 Then, the dichroism (Δμ = μ+ − μ−) was calculated from the difference between the two values at each data point. The XAS spectra were normalized for comparison of the XMCD results. Fig. 4 presents the obtained Mn L2,3-edge spectra of the FMl LSMO thin films on the two substrates, illustrating the XAS and XMCD spectra of the Mn ions in the LSMO/STO and LSMO/LSAT thin films. From the XAS results, Mn L3-edge spectra are roughly 641.95 eV. This confirms that our samples were well grown as La0.88Sr0.12MnO3 since the x of Sr in the thin films is 0.12 in good agreement with the spectra of various Sr concentrations studied by Abbate et al.23
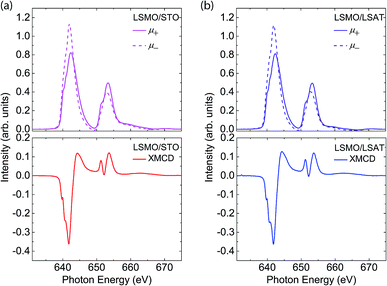 |
| Fig. 4 Mn L2,3-edge XAS and XMCD spectra of the epitaxial thin films of (a) LSMO/STO and (b) LSMO/LSAT. The XAS spectra were normalized to allow easier comparison of the XMCD results for the two samples. The dichroism is Δμ = μ+ − μ−. Both spectra were recorded at 80 K in the presence of an external magnetic field of approximately 0.7 T. | |
According to the XMCD sum rules, the spin magnetic moment Mspin and orbital magnetic moment Morb can be expressed as follows:24–26
|
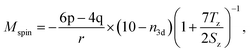 | (1) |
|
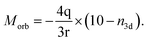 | (2) |
where

.
L3 and L2 are the integration range, and n3d is the occupation number of 3d electrons in a Mn ion. The 〈Tz〉/〈Sz〉 term can be neglected in this analysis.24,25 Here, we select n3d = 4 since Mn3+ is the dominant ion influencing the magnetic structure in La0.88Sr0.12MnO3 films. For the LSMO/STO film, Mspin and Morb were estimated to be 1.44 μB/Mn and −1.71 × 10−2 μB/Mn, respectively. These give Morb/Mspin = −1.19 × 10−2. For the LSMO/LSAT film, Mspin and Morb were estimated to be 1.50 μB/Mn and −1.51 × 10−2 μB/Mn, respectively. These give Morb/Mspin = −1.01 × 10−2. The negative sign indicates that the spin and orbital moments are antiparallel to each other as a result of Hund's rule. The spin magnetic moments are comparable to the results of the M–H measurements and in the same order as expected. The Ms values were approximately 1.06 μB/Mn for the LSMO/STO film and 1.29 μB/Mn for the LSMO/LSAT film. These are summarized in Table 1.
Table 1 Comparison of the lattice mismatches, spin magnetic moments, and orbital magnetic moments for the LSMO/STO and LSMO/LSAT thin films. The spin and orbital magnetic moments were calculated from the XMCD results (Mn3+ ions; n3d = 4) for each epitaxial thin film. The negative sign of the mismatch indicates that the films were subjected to in-plane compressive strain. The Ms values were obtained from the SQUID measurements
|
Lattice mismatch |
Ms (μB/Mn) |
Mspin (μB/Mn) |
Morb (μB/Mn) |
Morb/Mspin |
LSMO/STO |
−0.28% |
1.06 |
1.44 |
−1.71 × 10−2 |
−1.19 × 10−2 |
LSMO/LSAT |
−1.24% |
1.29 |
1.50 |
−1.51 × 10−2 |
−1.01 × 10−2 |
As demonstrated by the XMCD results, the different spin magnetic moments of the Mn ions on the two substrates correspond to the degree of compressive strain effected by the substrates. These are in good agreement with the SQUID results. With a bulk measurement of SQUID, the results of XMCD supports strongly to the structure–property relationship. Unlike the FMM of LSMO, the FMI cannot be explained simply by using the double-exchange interaction, as mentioned earlier in the text. Instead, the situation is more complicated as the long-range COO must be considered, since an epitaxial La0.88Sr0.12MnO3 film on a substrate is a lightly doped manganite with lattice distortion.11,20 Previous studies reported that the COO of lightly doped La1−xSrxMnO3 is associated with lattice distortion and the result of a complex interplay between electron–electron correlation, double exchange, and JT distortion in the Mn3+–O2−–Mn4+ bond.11,12,20,28 This can apply to our material model in this work. The orbital configuration associated with the electronic structure is related to the competition between these factors in the FMI phase. This causes a change in the magnetic structure of the epitaxial thin film. Thus, our results indicate that a large compressive strain effected by a substrate presents the COO transition with the JT distortion and the structure deformation. Like the M–H curves shown in Fig. 3(b), the XMCD results similarly demonstrated that the Mspin of the LSMO/LSAT thin film was larger than that of the LSMO/STO film. In contrast, the opposite was true for the orbital magnetic moments, where the Morb of LSMO/LSAT was smaller than that of LSMO/STO (Table 1). A large in-plane compressive strain gives rise to distortion of the MnO6 octahedra, resulting in different magnetic properties.
Consequently, this implies that a large compressive strain can afford unconventional properties with the electron configuration dependent on the complex interactions between the COO, JT distortion, and structural deformation. The consistent experimental results obtained from the bulk measurements and the surface-sensitive techniques support the idea that lattice distortion is intrinsically linked to magnetism in the FMI phase of La0.88Sr0.12MnO3 as a result of the complex interactions discussed above.
Conclusions
In summary, we have used X-ray spectroscopy and magnetic measurements to examine the magnetic structures of FMI La0.88Sr0.12MnO3 thin films, which are a potential model for the FMI phase of LSMO at low temperature. The structural deformation of the epitaxial thin films grown on two different substrates effected changes in the magnetic properties. Our results indicate an inextricable link between crystal structure and physical properties, particularly magnetic properties. In the epitaxial LSMO thin films, a large compressive strain caused displacement of the MnO6 octahedra in the out-of-plane direction and suppressed it in the in-plane direction. Distortion of the MnO6 octahedra influenced the double-exchange interaction in Mn3+–O2−–Mn4+ via a complex interplay with COO and JT distortion. This structural deformation links degrees of freedom such as spin, charge, and orbital in the lightly doped La1−xSrxMnO3. The correlation between the structural, electrical, and magnetic properties can be explained by the competition between the COO, JT distortion, and double-exchange interaction. This study provides an opportunity to improve our understanding of the nature of the FMI phase of lightly doped La1−xSrxMnO3 by exploiting the advantages of XMCD, a surface-sensitive technique. It suggests that further intensive study of a FMI LSMO will contribute to enhance our understanding of exotic physical and chemical properties in the regime for great potential applications.
Conflicts of interest
There are no conflicts to declare.
Acknowledgements
This research was supported by the Basic Science Research Program through the National Research Foundation of Korea (NRF), funded by the Ministry of Education (NRF-2018R1D1A1B07046980). H. J. J. and S. K. R. were supported by NRF Korea (2017K1A3A7A09016305).
Notes and references
- A. P. Ramirez, J. Phys.: Condens. Matter, 1997, 9, 8171 CrossRef CAS.
- J.-H. Park, E. Vescovo, H.-J. Kim, C. Kwon, R. Ramesh and T. Venkatesan, Nature, 1998, 392, 794 CrossRef CAS.
- A. Urushibara, Y. Moritomo, T. Arima, A. Asamitsu, G. Kido and Y. Tokura, Phys. Rev. B: Condens. Matter Mater. Phys., 1995, 51, 14103 CrossRef CAS.
- S. P. Jiang, J. Mater. Sci., 2008, 43, 6799 CrossRef CAS.
- J. Sacanell, J. H. Sánchez, A. E. R. López, H. Martinelli, J. Siepe, A. G. Leyva, V. Ferrari, D. Juan, M. Pruneda, A. M. Gómez and D. G. Lamas, J. Phys. Chem. C, 2017, 121, 6533 CrossRef CAS.
- N. Mannella, A. Rosenhahn, C. H. Booth, S. Marchesini, B. S. Mun, S.-H. Yang, K. Ibrahim, Y. Tomioka and C. S. Fadley, Phys. Rev. Lett., 2004, 92, 166401 CrossRef CAS PubMed.
- M. B. Salamon and M. Jaime, Rev. Mod. Phys., 2001, 73, 583 CrossRef CAS.
- M. Uehara, S. Mori, C. H. Chen and S. W. Cheong, Nature, 1999, 399, 560 CrossRef CAS.
- J. Deisenhofer, D. Braak, H.-A. Krug von Nidda, J. Hemberger, R. M. Eremina, V. A. Ivanshin, A. M. Balbashov, G. Jug, A. Loidl, T. Kimura and Y. Tokura, Phys. Rev. Lett., 2005, 95, 257202 CrossRef CAS PubMed.
- M. Paraskevopoulos, F. Mayr, J. Hemberger, A. Loidl, R. Heichele, D. Maurer, V. Müller, A. A. Mukhin and A. M. Balbashov, J. Phys.: Condens. Matter, 2000, 12, 3993 CrossRef CAS.
- Y. Endoh, K. Hirota, S. Ishihara, S. Okamoto, Y. Murakami, A. Nishizawa, T. Fukuda, H. Kimura, H. Nojiri, K. Kaneko and S. Maekawa, Phys. Rev. Lett., 1999, 82, 4328 CrossRef CAS.
- H. Nojiri, K. Kaneko, M. Motokawa, K. Hirota, Y. Endoh and K. Takahashi, Phys. Rev. B: Condens. Matter Mater. Phys., 1999, 60, 4142 CrossRef CAS.
- D. S. Rana, K. Takahashi, K. R. Mavani, I. Kawayama, H. Murakami, M. Tonouchi, T. Yanagida, H. Tanaka and T. Kawai, Phys. Rev. B: Condens. Matter Mater. Phys., 2007, 75, 060405 CrossRef.
- R. Prasad, H. K. Singh, M. P. Singh, W. Prellier, P. K. Siwach and A. Kaur, J. Appl. Phys., 2008, 103, 083906 CrossRef.
- R. Zhang, M. Liu, L. Lu, S.-B. Mi and H. Wang, J. Mater. Chem., 2015, 3, 5598 CAS.
- P. Dey, T. K. Nath and A. Taraphder, Appl. Phys. Lett., 2007, 91, 012511 CrossRef.
- L. Hu, Z. Sheng, X. Hu, R. Zhang, B. Wang, W. Song and Y. Sun, J. Phys. D: Appl. Phys., 2012, 45, 175002 CrossRef.
- D. E. Cox, T. Iglesias, E. Moshopoulou, K. Hirota, K. Takahashi and Y. Endoh, Phys. Rev. B: Condens. Matter Mater. Phys., 2001, 64, 024431 CrossRef.
- E. Dagotto, New J. Phys., 2005, 7, 67 CrossRef.
- B. Dabrowski, X. Xiong, Z. Bukowski, R. Dybzinski, P. W. Klamut, J. E. Siewenie, O. Chmaissem, J. Shaffer, C. W. Kimball, J. D. Jorgensen and S. Short, Phys. Rev. B: Condens. Matter Mater. Phys., 1999, 60, 7006 CrossRef CAS.
- A. Sadoc, B. Mercey, C. Simon, D. Grebille, W. Prellier and M.-B. Lepetit, Phys. Rev. Lett., 2010, 104, 046804 CrossRef PubMed.
- F. M. F. de Groot, J. C. Fuggle, B. T. Thole and G. A. Sawatzky, Phys. Rev. B: Condens. Matter Mater. Phys., 1990, 42, 5459 CrossRef CAS.
- M. Abbate, F. M. F. de Groot, J. C. Fuggle, A. Fujimori, O. Strebel, F. Lopez, M. Domke, G. Kaindl, G. A. Sawatzky, M. Takano, Y. Takeda, H. Eisaki and S. Uchida, Phys. Rev. B: Condens. Matter Mater. Phys., 1992, 46, 4511 CrossRef CAS.
- B. T. Thole, P. Carra, F. Sette and G. van der Laan, Phys. Rev. Lett., 1992, 68, 1943 CrossRef CAS PubMed.
- C. T. Chen, Y. U. Idzerda, H.-J. Lin, N. V. Smith, G. Meigs, E. Chaban, G. H. Ho, E. Pellegrin and F. Sette, Phys. Rev. Lett., 1995, 75, 152 CrossRef CAS PubMed.
- Y. Teramura, A. Tanaka, B. T. Thole and T. Jo, J. Phys. Soc. Jpn., 1996, 65, 3056 CrossRef CAS.
- J.-Y. Kim, T. Y. Koo and J.-H. Park, Phys. Rev. Lett., 2006, 96, 047205 CrossRef PubMed.
- S. Uhlenbruck, R. Teipen, R. Klingeler, B. Büchner, O. Friedt, M. Hücker, H. Kierspel, T. Niemöller, L. Pinsard, A. Revcolevschi and R. Gross, Phys. Rev. Lett., 1999, 82, 185 CrossRef CAS.
Footnote |
† These authors contributed equally to this work. |
|
This journal is © The Royal Society of Chemistry 2019 |
Click here to see how this site uses Cookies. View our privacy policy here.