DOI:
10.1039/C8RA08694J
(Paper)
RSC Adv., 2019,
9, 1726-1733
Influence of magnetic field on electrical and thermal transport in the hole doped ferromagnetic manganite: La0.9Na0.1MnO3
Received
20th October 2018
, Accepted 7th January 2019
First published on 14th January 2019
Abstract
We report the magnetization (M), magnetostriction, electrical resistivity (ρ), thermal conductivity (κ) and thermopower (S) of polycrystalline La0.9Na0.1MnO3 over a wide temperature range of 5 to 360 K. This sample undergoes a paramagnetic to ferromagnetic transition around TC = 274 K and electrical resistivity ρ shows an insulator–metal transition around TIM = 292 K. The sign of thermopower S is positive in the entire temperature range which indicates that majority charge carriers are holes. Thermopower exhibits a peak and thermal conductivity shows a dip at TC in the absence of magnetic field. Large difference between the experimentally determined activation energies of ρ and S in the insulating state indicates small polaron hopping dominant conduction above TIM. Polaron formation above TC, was further confirmed from the anomaly observed in thermal expansion (ΔL/L0) which shows a change in slope at TIM. In the vicinity of TC at 3 T applied field, magneto-thermopower (∼61.5%) is larger than magnetothermal conductivity (∼12.7%) and magnetoresistance (∼49%).
1. Introduction
Perovskites containing Mn ions (i.e. La1−xAxMnO3, A = Ca2+, Ba2+, Sr2+ etc.) have been one of the hot areas of research in physics and materials chemistry because of their colossal magnetoresistance (CMR) property and its prospective applications.1–3 Researchers are intrigued by the rich distinctive physical properties of every member of the manganite-family. From a fundamental point of view, manganites show diverse phenomena such as ferromagnetism, antiferromagnetism, multiferroism, magnetically coupled or independent structural transitions, nano- meso- and micro-scale phase separation, insulator–metal (IM) transition, spin-, charge- and orbital ordering etc.1,4
LaMnO3 (LMO) containing Mn3+ (t32ge1g) cations is a layered A-type antiferromagnetic (AFM) insulator with a Neel temperature (TN) of 140 K. Substitution with divalent alkaline earth cations (A = Ca2+, Sr2+ etc.) at the La3+ site transforms Mn3+ into an equal amount of Mn4+ (t32ge0g) and introduces ferromagnetic (FM) spin ordering for x = ∼0.18–0.45 in La1−xAxMnO3.5 Ferromagnetism and metallic conduction in the divalent ion doped manganites are understood in terms of Zener's double exchange (DE) model.6 Ferromagnetic metallic state can also be induced by substitution of monovalent cations such as Na1+, K1+ or Ag1+ for La3+ which theoretically should introduce twice the number of holes (or Mn4+) in the structure compared to the same concentration of divalent cation substitution and hence ferromagnetism can be obtained for a smaller amount of Na1+ or K1+ compared to any divalent ions.
Although thermal transport of hole doped LMO has been investigated in the past few years, the role of magnetic field on the thermal transport of Na1+ doped LMO system has been scarcely studied so far. Thermopower (S) is sensitive to band structure and also can probe majority charge carriers. Also S is less affected by the grain boundaries and hence probe intrinsic electrical conduction within grains unlike dc resistivity whose magnitude and temperature dependence is severely modified by grain boundaries. Therefore, S is an effective way to study the transport properties in polycrystalline samples.7–9 Both Shimura et al.10 and Ahmed et al.11 obtained a negative S in La1−xAxMnO3 (x ≥ 0.10) above ferromagnetic transition temperature, but they did not shed light on the trend of thermopower or thermal conductivity under the influence of magnetic field. Studies show that the sign of S(T) can be negative or positive both reflecting holelike or electronlike transport, depending on the temperature and the degree of elemental substitution. 10% Na doping is a critical composition form the point of view that in Ca2+ or Sr2+ doped compositions S alters the sign with lowering temperature as Mn4+ content becomes as high as ∼26% (ref. 12) or ∼25%,13 respectively. La0.9Na0.1MnO3 belongs to space group R
c like the parent compound with a small decrease in Mn–O bond length and increase in 〈Mn–O–Mn〉 angle compared to undoped LMO.14–16 A doping level exceeding 20% changes the structure from rhombohedral to orthorhombic one.16 Considering tight-binding approximation for ABO3 structures, doping Na1+ at La3+ site affects charge-carrier (eg electron) bandwidth, W (
where θ = 180 − 〈Mn–O–Mn〉 and dMn–O is the Mn–O bond length) advancing DE interaction in the structure.17 W rises rapidly with a small increase in 〈Mn–O–Mn〉 angle or decrease in Mn–O length favoring the evolution of the insulator–metal transition16 which explains the enhancement of TC by ∼150 K in only 10% Na doped LMO.18
Recently, nearly 100% suppression of thermopower (negative magneto-thermopower) was reported in antiferromagnetic Nd0.75Na0.25MnO3.19 An interesting correlation between magneto-thermopower and magnetoresistance was also reported in the same. There has been no report on combined study of thermopower, thermal conductivity and resistivity in Na-doped LaMnO3. In this work, we report simultaneous measurements of the dc electrical resistivity (ρ), thermal conductivity (κ) and thermopower (S) of polycrystalline La0.9Na0.1MnO3 as a function of temperature without and with an external magnetic field. In addition, magnetization, linear thermal expansion and field dependence of magnetostriction are also reported. Our investigation on temperature dependence of magnetization indicates paramagnetic (PM) to ferromagnetic transition at TC = 274 K upon cooling. Magnetic ordering is accompanied by an insulator–metal transition identified by the peak in resistivity curve at TIM = 292 K. Thermopower S reveals a hole doping characteristics above and below TIM. The theoretical fitting of S(T) curve indicates that at the FM metallic region, diffusion; phonon drag; magnon drag all coexist whereas at low temperatures (below 80 K), magnon drag dominates the thermopower value. Temperature and field dependent measurements show dominant lattice contribution to the heat conductivity κ.
2. Experimental details
Polycrystalline La0.9Na0.1MnO3 sample was synthesized by solid-state method using high purity La2O3, MnO2 and NaCO3 powders. Stoichiometric amount of the well mixed powders was calcined at 1000 °C for 24 h followed by sintering at 1150 °C for 48 h in few steps of intermediate grinding. The Rietveld refinement of the XRD data reveals the sample belongs to space group R
c of the rhombohedral crystal structure. Refined lattice constants of the unit cell are a = b = 5.5226 Å; c = 13.3481 Å. Magnetization measurements were performed using a Physical Property Measuring System (PPMS), equipped with a vibrating sample magnetometer. The temperature dependence of linear thermal expansion and field dependence of thermal expansion (Joule magnetostriction) were measured using a miniaturized capacitance dilatometer probe designed for PPMS.20 Four probe dc resistivity, thermopower and thermal conductivity were measured simultaneously at each stabilized temperature using the standard thermal transport option (TTO) for PPMS. In TTO, thermal conductivity (κ) is measured directly from the applied heater power used to create temperature gradient (ΔT) between two ends of the sample. Any heat loss during the measurement is also estimated by the software and the errors due to heat loss is excluded during calculation. However, for thermopower (S) the voltage drop (ΔV) across the hot and cold thermometer probes is also monitored by the TTO system and measured once a stable temperature gradient is created across these two thermometer probes due to the application of heat. PPMS served as a platform to vary temperature and magnetic field. The error for S is almost negligible, whereas, for κ it is <±0.1% at T = 10–100 K and <±5% at higher temperatures. Density of the pellet used for TTO measurement was estimated using Archimedes' principle which was close to 95% of its theoretical value. Magnetoresistance (MR), magnetothermal conduction (MTC) and magneto-thermopower (MTEP) at 3 T magnetic field of the sample is calculated using the standard relations:
respectively.
3. Results and discussion
3.1 Magnetic properties
Fig. 1 shows the temperature dependent magnetization (M) of polycrystalline La0.9Na0.1MnO3 (LNMO) sample measured in an applied field of μ0H = 0.1 T. The rapid increase of magnetization at T = TC indicates the onset of ferromagnetism. The ferromagnetic Curie temperature TC = 274 K is determined from the minimum of dM/dT curve as shown in the same figure. The temperature variation of inverse susceptibility (1/χ) and high temperature Curie–Weiss fit, 1/χ = C/(T − θW) of the data points are shown in the inset (a) of Fig. 1. C is the Curie constant given by C= Nμ0μB2μeff2/3kB, where N is the total number of PM entities per cubic meter. Best fitting above TC in the linear region of 1/χ, gives effective moment μeff = 5.98μB and Weiss temperature θW of 279.3 K which is few kelvin higher than the TC obtained from M–T curve. The theoretical effective magnetic moment is calculated using the equation,
where, g is the Lande factor and S is the total spin quantum number. In La1−xNaxMnO3, as we have both Mn3+ and Mn4+ spin, the modified S will be, Savg = 2x × SMn4+ + (1 − 2x) × SMn3+. As both Mn ions are in the high spin state, i.e. SMn4+ = 3/2 and SMn3+ = 2, the experimentally estimated μeff is higher than the theoretically expected value of 4.68μB, considering effective moment of non-interacting Mn3+ and Mn4+ ions are 4.89μB and 3.87μB, respectively. Enhanced μeff indicates that spins are not completely independent in the PM state. It is reported that dynamical ferromagnetic nanoclusters do exists in many manganites such as in (Sm0.65Sr0.35)MnO3 and Pr0.65Ca0.25Ba0.1MnO3 above TC.21,22 Therefore, the above mentioned findings (high values of μeff and θW > TC) indicate existence of small fraction of ferromagnetic cluster above TC in LNMO. Inset (b) of Fig. 1 shows field dependence of M at 10 K. It shows soft FM behavior (coercive field = 58.05 Oe) with a maximum moment of 3.7μB/f.u at 3 T field, which could reach the saturation value of 3.8μB/f.u expected for 20% Mn4+ doped LMO at higher magnetic field. Hence, 10% Na1+ substitution dopes nearly 20% of Mn4+ in the system.
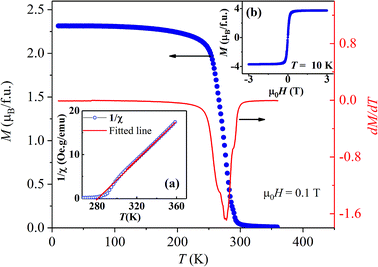 |
| Fig. 1 Temperature dependence of magnetization (M) and dM/dT at an applied field μ0H = 0.1 T, inset (a) shows inverse susceptibility vs. temperature plot, here solid line represents the Curie–Weiss fitting and (b) shows field dependent magnetization of La0.9Na0.1MnO3 at 10 K. | |
3.2 Thermal expansion properties
Fig. 2 shows the linear thermal expansion (ΔL/L0) of LNMO upon cooling from 320 K to 200 K at an external magnetic field of μ0H = 0, 1 and 3 T. L0 is the value of L at 320 K (normalizing temperature), the maximum operating temperature of the capacitance dilatometer. At zero field, ΔL/L0 decreases with lowering temperature, which indicates a contraction in length of the sample. However, ΔL/L0 changes slope at the onset of paramagnetic–ferromagnetic transition similar to La0.6Y0.07Ca0.33MnO3.23 As the external field is applied, the value of ΔL/L0 decreases around TC and the change of slope also shifts towards higher temperature because TC itself increases with increasing strength of the magnetic field. The anomaly in ΔL/L0 around TC can be attributed to the localization–delocalization transition of magnetic polarons as in La0.6Y0.07Ca0.33MnO3 (ref. 23) as well as in La0.67Ca0.33MnO3.24 Magnetic polaron is an entity in which Mn3+ (d4) spins are ferromagnetically aligned locally around a Mn4+ (d3) ions: eg carrier hops freely within these superparamagnetic clusters. Due to Jahn–Teller nature of Mn3+, lattice distortion also accompanies local spin polarization. Localization of magnetic polarons above TC causes extra contribution to thermal expansion, which is released when magnetic polarons collapses below TC leading to decrease in volume. Upon application of magnetic field above TC, size of magnetic polaron expands and they start percolating which results in enhancement of TC and shift of the thermal expansion anomaly towards higher temperature. As the magnetic field strength exceeds a critical value, the anomaly is suppressed as the crossover between the low-volume FM and high-volume PM state disappears.
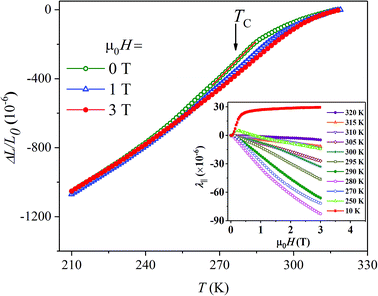 |
| Fig. 2 Temperature dependence of thermal expansion, ΔL/L0 (normalizing temperature 320 K) at 0, 1 and 3 T applied fields of La0.9Na0.1MnO3 sample. Inset shows magnetostriction, λ∥ (= [L(H) − L(H = 0)]/L0) at various temperatures. The measurement uncertainty is about <±3%. The error bar is not shown for purposes of clarity. | |
The inset of Fig. 2 shows the field dependence of longitudinal magnetostriction i.e., λ∥ (= [L(H) − L(H = 0)]/L0) at selected temperatures covering both paramagnetic and ferromagnetic regions. At 10 K, λ∥ increases rapidly in the low magnetic fields and saturates above 0.5 T. However, in the proximity of TC (250–310 K), λ∥ decreases with increasing strength of the magnetic field and does not show saturation. λ∥ decreases continuously, reaches a minima of −83 × 10−6 near TC with a subsequent increase for higher temperatures indicating strong spin–lattice and charge–lattice coupling at TC. The positive magnetostriction at 10 K is caused by increase in length (anisotropic in nature) due to spin–orbit interaction below TC. However, negative magnetostriction at and above TC is due to the collapse of magnetic polarons.
The maximum volume magnetostriction is given by ω = λ∥ + 2λ⊥, where λ∥ and λ⊥ are the longitudinal and transverse components of magnetostriction. They are usually measured using strain gauge technique. It was not possible to measure λ⊥ in our dilatometer probe as it can only measure magnetostriction along field direction, i.e. λ∥. In manganites,23 magnetostriction in the PM state and close to TC is isotropic, i.e., λ∥ ∼ λ⊥. Therefore, ω near TC in LNMO can also be expressed as ω = 3λ∥ which decreases at TC in zero field.
3.3 Electrical and thermal transport properties
Temperature dependence of the direct-current (DC) resistivity (ρ) at μ0H = 0 and 3 T magnetic fields are shown in Fig. 3(a). Upon lowering the temperature, ρ(T, H = 0 T) initially increases as like a semiconductor and exhibits a sharp peak at TIM = 292 K followed by metallic-like behaviour with lowering the temperature further. The insulator–metal (IM) transition temperature TIM i.e. the position of resistivity peak is higher than TC (= 274 K), determined from magnetization measurements, is similar to the report by Ye et al.25 The IM transition temperature is also higher than θW (= 279.31 K) estimated from the Curie–Weiss fitting with inverse magnetic susceptibility. Previous reports on similar compounds showed a broad maximum below the resistivity peak due to grain boundaries, those act as regions of enhanced scattering for the conduction electrons and such maximum is absent in our sample.15,16,18 The peak value of resistivity is reduced and TIM is shifted up under the application of magnetic field.
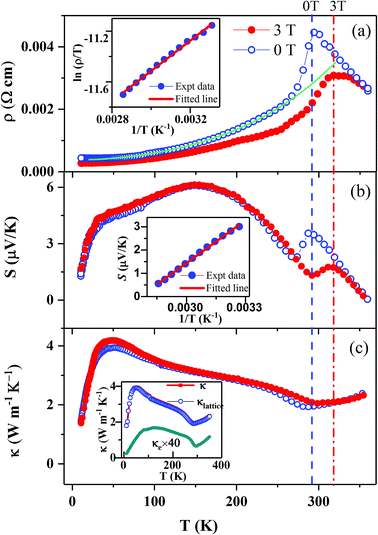 |
| Fig. 3 Variation of (a) resistivity ρ (b) thermoelectric power S and (c) thermal conductivity κ with temperature of La0.9Na0.1MnO3 at zero field (open symbol) and 3 T (closed symbol). The solid curve in (a) is the function ρ(T) = ρ0 + AT2 + BT4.5. Inset (a) shows ln(ρ/T) vs. 1/T plot, and the solid line is obtained by fitting the data according to the small polaron hopping model. Inset (b) includes S vs. 1/T plot and the solid line is a fitting based on the data. Inset (c) shows plot of κ, κe and κlattice vs. temperature. | |
Fig. 3(b) and (c) show the temperature dependence of thermopower S and thermal conductivity κ, respectively, measured together with dc resistivity. In zero field, sign of S is positive throughout the whole temperature region, which reflects eg hole of Mn4+: t32ge0g is the majority charge carrier. Shimura et al.10 obtained a negative S above TC for La0.88Na0.09Mn0.96O3 and La0.84Na0.12Mn0.97O3 which was attributed to doping induced alteration of conduction from charge transfer type to the Mott-type. In the PM state, S(T) increases with lowering temperature and exhibits a peak at the IM transition and below which it decreases rapidly. However, in the FM state S increases again and shows a broad maximum around 154 K. S(T) shows a striking resemblance with ρ in the presence of an external magnetic field. Applied magnetic field lowers S value prominently in the vicinity of TIM and shifts the peak position to high temperature but it neither influences the value nor the position of the broad maximum at low temperature.
Thermal conductivity (κ) in the paramagnetic state decreases with decreasing temperature (i.e. dκ/dT > 0) which is unusual for a crystalline compound showing insulating like behavior (Fig. 3(c)) but similar to other hole-doped manganites.26,27 κ(T) shows a dip in the vicinity of the magnetic ordering and a few kelvins below the peak in ρ(T) and S(T). Within the FM state below TC, κ(T) increases down to ∼43 K, where it reaches a peak and below that κ decreases rapidly. The magnitude of κ in the metallic region varies in the range of 2–4.13 W m−1 K−1 which is higher than the parent compound (<1 W m−1 K−1 below room temperature)28 but comparable with the divalent ion doped systems with similar Mn4+ concentration (κ ∼ 2.3 W m−1 K−1 in La0.8Ca0.2MnO3).8 The behavior of κ in the paramagnetic region is amorphous like i.e., κ decreases with decreasing T. Such characteristic of κ is attributed to the local anharmonic lattice distortions associated with small polarons or dynamical JT distortion in A-site doped manganites.26 In general, κ has lattice component κlattice due to phonon vibrations, electronic component κe and the spin wave component κm, κ = κlattice + κe + κm. Above TC, κm is negligible and the thermal conductivity is primarily due to the other two components. Measured value of ρ is used to calculate κe using the Wiedemann–Franz law, expressed by κe = L0T/ρ, where L0 = π2kB2/3e2 is the Lorentz constant for metals where charge carriers behave like free-electrons (degenerate limit).29 The estimated κe is two orders of magnitude smaller than the total κ in the LNMO sample, which indicates phonon contribution to κ (inset of Fig. 3(c)) is dominant than heat carried by electrical charges. Enhancement of κ on entering the metallic state was argued due to the weakening of JT distortion and delocalization of the charge carriers.26 The pair distribution function based on the neutron scattering data shows that MnO6 octahedra in La1−xCaxMnO3 (x = 0.2 and 0.25) has uniform Mn–O and O–O bond lengths at low-temperature and when the temperature rises towards IM transition the disorder of Mn–O bond lengths increases followed by the formation of small polarons.30 Reduction of ρ under magnetic field should have increased κe contribution, but in the whole temperature range κe value is negligible. A dip in κ(T) at TC or TN is also seen in other magnetic oxides such as MnO,31 and it is likely to be caused by decrease in scattering of thermal phonon by spin-fluctuations, which decreases with lowering temperature. The peak at the temperature ∼ 43 K is due to transition from umklapp scattering to defect dominated scattering at lower temperatures.
Fig. 4 gives a clear picture of the influence of magnetic field on the physical properties of the system. MR increases with lowering temperature in the paramagnetic state and it reaches a peak value (∼49%) at TC. MR remains significant below TC and reaches ∼40% at 10 K. In the ordered state, ρ rises sharply due to narrowing band width which leads to localization of charges and the formation of small polarons. Application of external magnetic field reduces the relative angle between spins which increases the electron bandwidth and enhances mobility of electrons. In addition to this, destruction of magnetic polarons under applied magnetic field gives rise to a large negative MR. For T ≪ TC, the magnetoresistance is due to grain boundary effect: tunnelling of spin-polarized electrons between ferromagnetic grains via thin grain boundaries.32 MTEP curve (Fig. 4(b)) reveals very interesting fact that S is affected by the external field mostly around IM transition temperature, whereas S in the FMM region (T < 260 K) remains unaffected unlike ρ(T). MTEP is much larger than MR in the temperature interval between TC (=274 K) and TIM (=292 K) where the spin ordering is destroyed. Unlike MR which is proportional to the density of states (DOS) at the Fermi level, MTEP depends on the asymmetry of DOS around the Fermi level due to the spin-up and spin-down states.33 Hence, in the FMM state MR is higher than MTEP but as the magnetic ordering collapses MTEP value enhances more than MR. However, MTEP observed here at comparatively low magnetic field (61.5% at 3 T) is comparable with the value (∼80–100%) reported for antiferromagnetic Nd0.75Na0.25MnO3 system obtained at 5 T.19 The magnitude of MTEP of LNMO is also close to the similar amount of hole doped La0.8Ca0.2MnO3 which shows 80% change in thermopower but at 5.7 T magnetic field8 and single crystal La0.92Sr0.18MnO3 showing MTEP of almost 90% at 7 T.13 Suppression of S with magnetic field as in LNMO, is observed in few other oxides too.34,35 Yamamoto et al.34 have shown that in the weakly ferromagnetic CaRu0.8Sc0.2O3 external magnetic field suppresses S, with little influence on ρ. They have also showed a correlation between the spin entropy and magnetothermopower. A similar explanation was also put forward by Repaka et al.36 for the room temperature ferromagnet. In antiferromagnetic Nd0.75Na0.25MnO3, a significant magnetothermopower was found above the Neel temperature even though magnetoresistance was negligible.19 However in the present study, ρ and S both are significantly affected by the magnetic field around TIM (much above TC). It appears that S is affected by the change in magnetization to a larger extend than the resistivity. A possible explanation is the decrease in the magnitude of thermopower is partly from the suppression of spin entropy. Another possibility is that the magnetic field affects the spin-dependent band structure around the Fermi level and it is reflected in magnetothermopower as mentioned before. Theoretical modelling is certainly needed to have deeper understanding.
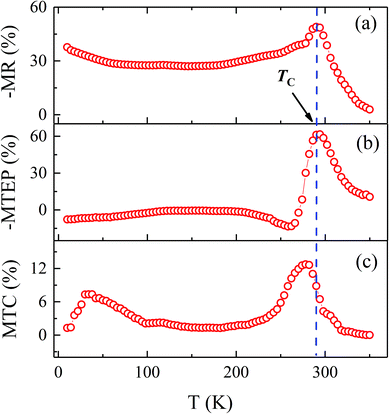 |
| Fig. 4 (a) Plot of magnetoresistance (MR), (b) magneto-thermal conduction (MTC) and (c) magneto-thermopower (MTEP) of La0.9Na0.1MnO3. | |
At first sight, thermal conductivity seemed to have very small dependency on external magnetic field (Fig. 3(c)). However, measurement of κ(T) in magnetic field makes it easier to understand the influence of spins on the heat transport property of LNMO. Temperature dependence of κ in the hole doped manganites is attributed to two different processes; first one is considered due to the scattering of phonons by Jahn–Teller (JT) distorted Mn3+O6 octahedra26 and second is to the scattering of phonons by spin fluctuations.8 At 3 T field, reduction of spin fluctuation facilitates heat flow in the material produces a positive MTC value of about 12.7% near TC, suggesting spin–phonon scattering as a decisive factor in κ (Fig. 4(c)). Under magnetic field, suppression of the dip in κ around TC and marginal enhancement in conductivity can be attributed to the scattering of phonons by spin-wave. MR and MTC results unquestionably establish a strong electron–phonon–spin coupling in the Na doped LMO compound.
In manganites, high temperature transport mechanism is often analyzed using fitting of variable-range hopping or small-polaron hopping model with the ρ(T) data. However, in some cases, it is difficult to reach to a satisfactory conclusion based on the fitting results of ρ(T) alone, such as in electron doped La0.9Te0.1MnO3 system37 which makes it necessary to fit the S(T) data as well. In our sample, high temperature (TIM ≤ T ≤ 370 K) ρ(T) and S(T) data fitted well with the small-polaron hopping (SPH) model, given by
|
ρ(T) = ρ0T exp(Eρ/kBT)
| (1) |
and
|
S(T) = kB/e(ES/kBT + α),
| (2) |
respectively, where
ρ0 and
α are constants,
kB is the Boltzmann constant, e is the electron's charge and
Eρ and
ES are the activation energy values obtained from resistivity and thermopower data fitting, respectively (inset of
Fig. 3(a) and (b)). We found that
Eρ (103.14 meV) is an order of magnitude higher than
ES (6.13 meV).
Eρ ≫
ES is a hall mark of electrical conduction by small polaron hopping between the neighboring sites.
38,39 ES corresponds to the hopping energy of carriers between energetically equivalent sites with Fermi level as a reference point for the entropy, while
Eρ contains energy to create the carriers too. Obtained value of
Eρ agrees well with the proper stoichiometric La
0.87Na
0.13MnO
3 compound studied by Malavasi
et al.40 Thus, the electrical conduction in LNMO is governed by hopping of small polarons with the energy given by 2(
Eρ −
ES) = 194.02 meV.
8
The thermal variation of resistivity below the upturn near TC (T < 260 K) is dominated by electron–electron scattering (T2 dependency)41 and electron–magnon or, spin wave scattering in the ferromagnetic phase (T4.5 dependency).41,42 We have fitted the present experimental data in the FMM phase using an expression of the form, ρ(T) = ρ0 + AT2 + BT4.5 nearly perfectly as shown by the solid line in Fig. 3(a), where ρ0 is the residual resistivity due to the temperature independent scattering processes. The fitting parameter A (∼2.17 × 10−8 Ω cm K−2) obtained for our samples is similar to that reported by Urushibara et al.41 for La–Sr–Mn–O system and suggests an important role of the electron–electron scattering process below 260 K in the resistivity. Value of B (∼4.73 × 10−15 Ω cm K−4.5), related to the T4.5 behavior suggests that electron–magnon scattering too strongly contributes to the electrical conduction in the FMM region and causes the observed trend in ρ at this temperature range.
The thermopower data in the low temperature metallic region is analyzed using an equation,37
|
S = S0 + S1T + S3/2T3/2 + S3T3 + S4T4
| (3) |
Here, the first term S0 is estimated from the high temperature data extrapolation at T = 0 K. Second term represents contribution from diffusion, the third term of the equation denotes magnon drag or the single-magnon scattering processes, while the fourth term with T3 dependency is related to the phonon drag contribution and the last term represents the spin-wave fluctuation in the FM phase below TC. In general, S(T) of manganites in FM region is analysed without considering the diffusion and phonon drag terms which was quite logical considering the position of low temperature broad peak. Phonon drag peak in thermopower is usually observed near temperature θD/5, where Debye temperature θD is about 320 K for LMO.43 Thus, the phonon drag contribution is supposed to show maximum around 60 K much below the observed thermopower peak here at 150 K. To ratify further, LNMO data below T ≪ TC was fitted with equations considering (i) all the terms in eqn (3) and (ii) excluding the diffusion and phonon drag contribution terms in eqn (3). Fig. 5(a) shows a better fitting for the first case throughout a wide temperature range (49–260 K) whereas, the latter one fits the experimental data points only between 150–260 K. Best fitting results (S0 = 5.7 μV K−1, S1 = −0.116 μV K−2, S3/2 = 0.013 μV K−5/2, S3 = −2.77 × 10−6 μV K−4 and S4 = 4.06 × 10−9 μV K−5) show S3/2 ≫ S3, suggesting that the electron–magnon scattering strongly affects the broad peak in the S vs. T curve but phonon drag is not negligible either. However, in the temperature range below the broad peak (80–30 K) thermopower decreases rapidly showing a T3/2 dependence (inset of 4(a)) like in other hole doped manganites.9,44
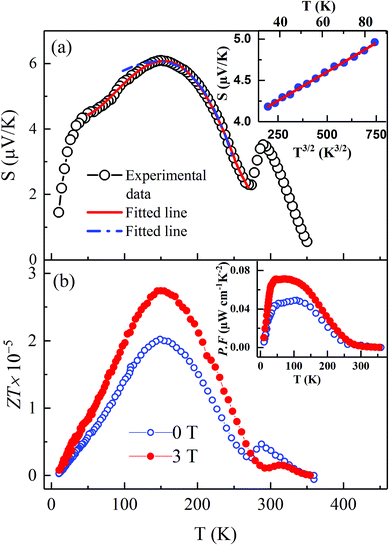 |
| Fig. 5 (a) Temperature dependence of the thermopower for the La0.9Na0.1MnO3 sample. The solid line (red) corresponds to the expression (i) S = S0 + S1T + S3/2T3/2 + S3T3 + S4T4 and dashed line (blue) represent the fitting with (ii) S = S0 + S3/2T3/2 + S4T4 as discussed in the text, (b) temperature dependence of figure of merit, ZT for the same sample. Inset of (a) shows T3/2 dependency of S at low temperature and (b) power factor (S2/ρ) at 0 and 3 T fields. | |
Fig. 5(b) shows the figure of merit ZT, expressed by
a characteristic parameter for the thermoelectric materials. As ZT has a square term of thermopower, it shows a peak around the maxima of S(T). 10% Na doped LMO exhibits better value of ZT compared to La0.9Te0.1MnO3 at room temperature37 but it is still quite low to be useful for the practical purpose of a thermoelectric material for which ZT ≥ 1. In the inset of Fig. 5(b), the temperature variation of power factor
is shown. At zero and 3 T applied field, P.F. shows a similar trend with decreasing temperature. It starts increasing rapidly below TC, goes through a maximum value and then decreases to zero. P.F. has highest value of 0.07 μW cm−1 K−2 around 47 K at 3 T field.
4. Conclusions
To conclude, effects of magnetic field on the thermal expansion, charge transport and thermal conduction mechanism for aliovalent Na1+ doped LaMnO3 were investigated. We have found anomaly in thermal expansion behavior around TC. Electrical resistivity and thermopower data analysis in the insulating region reveal that conductivity at high temperature is governed by small-polaron hopping mechanism in La0.9Na0.1MnO3. Anomaly of κ around paramagnetic–ferromagnetic transition suggests coupling between thermal conductivity and magnetism. Application of magnetic field enhances thermal conductivity while suppresses the magnitude of thermopower and resistivity. The magnitude of magneto-thermopower (∼61.5%) is larger than the magnetoresistance (∼49%) and magnetothermal conductivity (∼12.7%).
Conflicts of interest
There are no conflicts to declare.
Acknowledgements
R. M. thanks the Ministry of Education for supporting this work (Grant no. MOE2014-T2-48/R144-000-349-112 and MOE2016-T2-2-098/R144-000-381-112).
References
- C. N. R. Rao and B. Raveau, Colossal Magnetoresistance, Charge Ordering, and Related Properties of Manganese Oxides, World Scientific, Singapore, 1998 Search PubMed.
- J. Hu, L. Shi, Q. Liu, H. Huang and T. Jiao, Improved oxygen reduction activity on silver modified LaMnO3–graphene via shortens the conduction path of adsorbed oxygen, RSC Adv., 2015, 5, 92096 RSC.
- H. Xu, Y. Ma, S. Zhao, W. Huang, Z. Qua and N. Yan, Enhancement of Ce1−xSnxO2 support in LaMnO3 for the catalytic oxidation and adsorption of elemental mercury, RSC Adv., 2016, 6, 63559 RSC.
- Y. Tokura, Critical features of colossal magnetoresistive manganites, Rep. Prog. Phys., 2006, 69, 797 CrossRef CAS.
- G. H. Jonker and J. H. Van Santen, Ferromagnetic Compounds of Manganese with Perovskite Structure, Physica, 1950, 16, 337–349 CrossRef CAS.
- C. Zener, Interaction between the d-Shells in the Transition Metals. II. Ferromagnetic Compounds of Manganese with Perovskite Structure, Phys. Rev., 1951, 82, 403 CrossRef CAS.
- A. Bhaskar, M.-S. Huang and C. –J. Liu, Effects of Fe doping on the thermal hysteresis of the La0.5Ca0.5MnO3 system, RSC Adv., 2017, 7, 11543 RSC.
- B. Chen, A. G. Rojo, C. Uher, H. L. Ju and R. L. Greene, Magnetothermal conductivity of La0.8Ca0.2MnO3, Phys. Rev. B: Condens. Matter Mater. Phys., 1997, 55, 15471 CrossRef CAS.
- P. Mandal, Temperature and doping dependence of the thermopower in LaMnO3, Phys. Rev. B: Condens. Matter Mater. Phys., 2000, 61, 14675 CrossRef CAS.
- T. Shimura, T. Hayashi, Y. Inaguma and M. Itoh, Magnetic and Electrical Properties of LayAxMnwO3 (A = Na, K, Rb, and Sr) with Perovskite-Type Structure, J. Solid State Chem., 1996, 124, 250–263 CrossRef CAS.
- A. M. Ahmed, S. A. Saleh, E. M. M. Ibrahim and H. F. Mohamed, Crystal structure and some transport properties of Na-doped LaMnOy, J. Magn. Magn. Mater., 2006, 301, 452–457 CrossRef CAS.
- R. Mahendiran, S. K. Tiwary, A. K. Raychaudhuri, R. Mahesh and C. N. R. Rao, Thermopower and nature of the hole-doped states in LaMnO3 and related systems showing giant magnetoresistance, Phys. Rev. B: Condens. Matter Mater. Phys., 1996, 54, R9604 CrossRef CAS.
- A. Asamitsu, Y. Moritomo and Y. Tokura, Thermoelectric effect in La1−xSrxMnO3, Phys. Rev. B: Condens. Matter Mater. Phys., 1996, 53, R2952 CrossRef CAS.
- M. P. Sharma, et al., Electric transport behaviour of sodium-substituted perovskites La1−xNaxMnO3 (for x = 0.1 and 0.2) and the effect of magnetic fields, J. Phys.: Condens. Matter, 2008, 20, 425220 CrossRef.
- S. Das and T. K. Dey, Structural and magnetocaloric properties of La1−yNayMnO3 compounds prepared by microwave processing, J. Phys. D: Appl. Phys., 2007, 40, 1855 CrossRef CAS.
- S. Roy, Y. Q. Guo, S. Venkatesh and N. Ali, Interplay of structure and transport properties of sodium-doped lanthanum manganite, J. Phys.: Condens. Matter, 2001, 13, 9547 CrossRef CAS.
- P. G. Radaelli, G. Iannone, M. Marezio, H. Y. Hwang, S.-W. Cheong, J. D. Jorgensen and D. N. Argyrion, Structural effects on the magnetic and transport properties of perovskite A1−xAx′MnO3 (x = 0.25, 0.30), Phys. Rev. B: Condens. Matter Mater. Phys., 1997, 56, 8265 CrossRef CAS.
- G. H. Rao, J. R. Sun, K. Bärner and N. Hamad, Crystal structure and magnetoresistance of Na doped LaMnO3, J. Phys.: Condens. Matter, 1999, 11, 1523 CrossRef CAS.
- D. V. Maheswar Repaka and R. Mahendiran, Giant magnetothermopower in charge ordered Nd0.75Na0.25MnO3, Appl. Phys. Lett., 2013, 103, 162408 CrossRef.
- R. Küchler, T. Bauer, M. Brando and F. Steglich, A compact and miniaturized high resolution capacitance dilatometer for measuring thermal expansion and magnetostriction, Rev. Sci. Instrum., 2012, 83, 095102 CrossRef PubMed.
- R. P. Borges, F. Ott, R. M. Thomas, V. Skumryev, J. M. D. Coey, J. I. Arnaudas and L. Ranno, Field-induced transition in the paramagnetic state of (Sm0.65Sr0.35)MnO3 associated with magnetic clusters, Phys. Rev. B: Condens. Matter Mater. Phys., 1999, 60, 12847 CrossRef CAS.
- A. Mleiki, R. Hanen, H. Rahmouni, N. Guermazi, K. Khirouni, E. K. Hlile and A. Cheikhrouhou, Study of magnetic and electrical properties of Pr0.65Ca0.25Ba0.1MnO3 manganite, RSC Adv., 2018, 8, 31755 RSC.
- M. R. Ibarra, P. A. Algarabel, C. Marquina, J. Blasco and J. García, Large Magnetovolume Effect in Yttrium Doped La-Ca-Mn-O perovskite, Phys. Rev. Lett., 1995, 75, 3541 CrossRef PubMed.
- C. Zhu, R. Zheng, J. Su and J. He, Ultrasonic anomalies in La0.67Ca0.33MnO3 near the Curie temperature, Appl. Phys. Lett., 1999, 74, 3504 CrossRef CAS.
- S. L. Ye, W. H. Song, J. M. Dai, K. Y. Wang, S. G. Wang, J. J. Du, Y. P. Sun, J. Fang, J. L. Chen and B. J. Gao, Large room-temperature magnetoresistance and phase separation in La1−xNaxMnO3 with 0.1 ≤ x ≤ 0.3, J. Appl. Phys., 2001, 90, 2943 CrossRef CAS.
- D. W. Visser, A. P. Ramirez and M. A. Subramanian, Thermal Conductivity of Manganite Perovskites: Colossal Magnetoresistance as a Lattice-Dynamics Transition, Phys. Rev. Lett., 1997, 78, 3947 CrossRef CAS.
- A. Ray and T. K. Dey, Thermal conductivity of La0.67(CaxSr1−x)0.33MnO3 (x = 0, 0.5, 1) and La0.6Y0.07Ca0.33MnO3 pellets between 10 and 300 K, Solid State Commun., 2003, 126, 147 CrossRef CAS.
- J. L. Cohn, J. J. Neumeier, C. P. Popoviciu, K. J. McClellan and Th. Leventouri, Local lattice distortions and thermal transport in perovskite manganites, Phys. Rev. B: Condens. Matter Mater. Phys., 1997, 56, R8495 CrossRef CAS.
- H.-S. Kim, Z. M. Gibbs, Y. Tang, H. Wang and G. J. Snyder, Characterization of Lorenz number with Seebeck coefficient measurement, APL Mater., 2015, 3, 041506 CrossRef.
- S. J. L. Billinge, R. G. DiFrancesco, G. H. Kwei, J. J. Neumeier and J. D. Thompson, Direct Observation of Lattice Polaron Formation in the Local Structure of La1−xCaxMnO3, Phys. Rev. Lett., 1996, 77, 715 CrossRef CAS PubMed.
- G. A. Slack and R. Newman, Thermal Conductivity of MnO and NiO, Phys. Rev. Lett., 1958, 1, 359 CrossRef CAS.
- R. Mahesh, R. Mahendiran, A. K. Raychaudhuri and C. N. R. Rao, Effect of particle size on the giant magnetoresistance of La0.7Ca0.3MnO3, Appl. Phys. Lett., 1996, 68, 2291 CrossRef CAS.
- M. Czerner, M. Bachmann and C. Heiliger, Spin caloritronics in magnetic tunnel junctions: ab initio studies, Phys. Rev. B: Condens. Matter Mater. Phys., 2011, 83, 132405 CrossRef.
- T. D. Yamamoto, H. Taniguchi, Y. Yasui, S. Iguchi, T. Sasaki and I. Terasaki, Magneto-thermopower in the Weak Ferromagnetic Oxide CaRu0.8Sc0.2O3: An Experimental Test for the Kelvin Formula in a Magnetic Material, J. Phys. Soc. Jpn., 2017, 86, 104707 CrossRef.
- A. Kumar, C. V. Tomy and A. D. Thakur, Magnetothermopower, magnetoresistance and magnetothermal conductivity in La0.95Sr0.05Co1−xMnxO3 (0.00 ≤ x ≤ 1.00), Mater. Res. Express, 2018, 5, 086110 CrossRef.
- D. V. Maheswar Repaka, T. S. Tripathi, M. Aparnadevi and R. Mahendiran, Magnetocaloric effect and magnetothermopower in the room temperature ferromagnet Pr0.6Sr0.4MnO3, J. Appl. Phys., 2012, 112, 123915 CrossRef.
- J. Yang, Y. P. Sun, W. H. Song and Y. P. Lee, Thermopower and thermal conductivity of the electron-doped manganite La0.9Te0.1MnO3, J. Appl. Phys., 2006, 100, 123701 CrossRef.
- A. J. Millis, P. B. Littlewood and B. I. Shraiman, Double Exchange Alone Does Not Explain the Resistivity of La1−xSrxMnO3, Phys. Rev. Lett., 1995, 74, 5144 CrossRef CAS PubMed.
- M. Jaime, M. B. Salamon, K. Pettit, M. Rubinstein, R. E. Treece, J. S. Horwitz and D. B. Chrisey, Magnetothermopower in La0.67Ca0.33MnO3 thin films, Appl. Phys. Lett., 1996, 68, 1576 CrossRef.
- L. Malavasi, M. Cristina Mozzati, P. Ghigna, C. B. Azzoni and G. Flor, Lattice Disorder, Electric Properties, and Magnetic Behavior of La1−xNaxMnO3+δ Manganites, J. Phys. Chem. B, 2003, 107, 2500–2505 CrossRef CAS.
- A. Urushibara, Y. Moritomo, T. Arima, A. Asamitsu, G. Kido and Y. Tokura, Insulator-metal transition and giant magnetoresistance in La1−xSrxMnO3, Phys. Rev. B: Condens. Matter Mater. Phys., 1995, 51, 14103 CrossRef CAS.
- K. Kubo and N. Ohata, A Quantum Theory of Double Exchange. I, J. Phys. Soc. Jpn., 1972, 33, 21 CrossRef CAS.
- B. F. Woodfield, M. L. Wilson and J. M. Byers, Low-Temperature Specific Heat of La1−xSrxMnO3+δ, Phys. Rev. Lett., 1997, 78, 3201 CrossRef CAS.
- M. Jaime, P. Lin, M. B. Salamon and P. D. Han, Low-temperature electrical transport and double exchange in La0.67(Pb, Ca)0.33MnO3, Phys. Rev. B: Condens. Matter Mater. Phys., 1998, 58, R5901 CrossRef CAS.
|
This journal is © The Royal Society of Chemistry 2019 |