DOI:
10.1039/C8QM00503F
(Research Article)
Mater. Chem. Front., 2019,
3, 137-144
Multi-length scale morphology of nonfullerene all-small molecule blends and its relation to device function in organic solar cells†
Received
2nd October 2018
, Accepted 19th November 2018
First published on 19th November 2018
Abstract
Nonfullerene all-small molecule organic solar cells (OSCs) have attracted significant attention due to their competitive performance compared to the well-studied polymer-based OSCs. Small molecules are considered to be materials of choice for industrial scale OSC production due to their relatively high purity, reproducibility, and well-defined molecular weights. Despite the recent progress in all-small molecule solar cells, the mesoscale morphology of these devices remains elusive. A fundamental understanding of the structure–property relationships of these systems is thus critical for further device performance enhancement. Here, we report a dramatic change in device performance of a nonfullerene all-small molecule bulk heterojunction system upon changing the side chains of the electron donor small molecules. Morphology analysis via resonant soft X-ray scattering shows that the average composition variation and the size of the smaller domains of the all-small molecule blends are the most influential factors that derive the photogeneration and collection efficiency of charges in efficient all-small molecule OSCs. The observed side-chain and processing initiated morphological changes resulted in distinct device efficiencies in these systems. Despite the fact that side chains are mainly meant to facilitate material dissolution, the current study clearly shows that they can also impose a significant effect on material organization and photovoltaic properties. The findings will give a clear understanding of the role of side chains and processing in material organization and device functionality, and the knowledge gained in this study is quite crucial in designing next-generation materials and devices.
1. Introduction
Unlike traditional inorganic solar cells, organic solar cells (OSCs) offer several unique features that enable integration of this technology with building infrastructures, vehicles and many other applications. This driving force has led to the synthesis of various electron donor and acceptor materials that have recently led to high-efficiency OSCs.1,2 The success of OSCs is highly influenced by the type of the donor/acceptor bulk heterojunction blend utilized as a photoactive layer. In particular, recent performance breakthroughs were made by replacing the benchmark fullerene molecules with non-fullerene small molecule electron acceptors (NFAs) that strongly convert red photons into electricity in addition to transporting free electrons.3–5 Following this line, the research of OSCs has also focused on utilizing an all-small molecule active layer by getting rid of polymer donors as well mainly due to the fact that small molecules can be relatively well purified and reproduced, and have well-defined molecular weights.6–8 Recent efforts have led to highly efficient nonfullerene all-small molecule OSCs whose performance is gradually approaching that of devices utilizing polymer electron donors.9–19 The most critical factors in OSC fabrication are not only designing the right materials or choosing the right combination of materials but also optimizing the device parameters by controlling the morphology of the active layers to optimize photocurrent production and transport.20–26 However, while the morphology of the polymer/acceptor blends has been relatively well studied and optimized, the fundamentals of the structure–property relationships at the mesoscale are still poorly understood for the nonfullerene all-small molecule systems.19,27
In both polymer and small molecule donors, the benzodithiophene (BDT) unit has been very popular over the past few years due to its enhanced electron delocalization and low highest occupied molecular orbital (HOMO) energy level.7,28–30 Following the high efficiency shown by two-dimensional conjugated D–A copolymers based on bithienylbenzodithiophene (BDTT) and fluorobenzotriazole (FBTA) as donors and acceptors, respectively, Bin et al. synthesized a high-performance A–D–A structured organic molecule H1131 using BDTT as the donor unit and FBTA as the acceptor unit with an ethylhexyl cyanoacetate unit as the end group to improve the electron affinity. As it has been shown for different polymers, having conjugated side groups in the BDT unit leads to a significant change in device performance.29 A control molecule H1231 with alkoxy side chains instead of alkylthienyl conjugated side chains was synthesized to investigate the effect of side chains on solar cell performance (Fig. 1) in small molecule donor systems. H11 with conjugated side chains shows better absorption, a low-lying HOMO energy level, better carrier mobility and bimodal crystallites in blends with IDIC compared to H12, which are favorable for device performance. The H11:IDIC blend has demonstrated a remarkable PCE of 9.73%, which was among the highest reported values for all-small molecule OSCs. Although the current efficiency has surpassed this limit, as shown in Fig. 1 due to the similarity of the backbone with BDTT, H11 seems to be a good and representative model system for an in-depth morphological study.
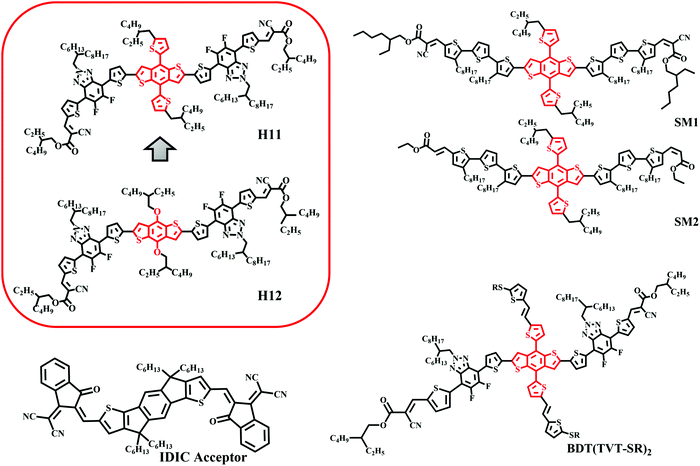 |
| Fig. 1 H11 and H12 small molecules (top left) used in the study and some of the small molecule donors based on a central BDT unit with thienyl substitution together with the common non-fullerene acceptor IDIC used in the nonfullerene all-small molecule OSCs. | |
Resonant soft X-ray scattering (R-SoXS)32 is an advanced tool that can be used to quantify the average composition variation and the size of the domains and help the OSC community to build new morphology–performance relationships in a large number of polymer-based OSCs. Although grazing incidence wide-angle X-ray scattering has been used to explain the observed difference in device performance of all-small molecule nonfullerene OSCs as a function of chemical structure,11,14,27,31 a clear resonant soft X-ray scattering (R-SoXS) study, which has become a standard to investigate the length scale of phase separation in organic photovoltaic research, is rarely performed in this field of research. Here, we discuss the quantitative morphology–performance relations for a pair of all-small molecule systems based on representative small molecule H11 and its analog H12, without conjugated side chains. In composite organic semiconductor films, charge generation and transport are directly influenced by the morphology of the photoactive film, and this eventually affects device performance. The change from alkoxy to conjugated side chain (alkylthienyl) in the donor molecule has shown a significant difference in performance. Therefore, an investigation on morphology–performance relationships will definitely be necessary for further development of the all-small molecule nonfullerene OSCs and their side chain engineering.
2. Experimental methods
Samples for measurements and the active layer of the devices were prepared under the same conditions as those used for device fabrication.31 The active layers were spin coated from a chloroform solution with a 2
:
1 weight ratio of donor
:
acceptor inside a N2 glove box. The active layers were thermally annealed at 120 °C for 10 minutes.
2.1 R-SoXS measurements
R-SoXS measurements were performed in transmission geometry with linearly polarized photons under high vacuum (1 × 10−7 Torr) at beamline 11.0.1.233,34 at the Advanced Light Source (ALS). The scattering 2D images were collected in a vacuum cooled 2D charge-coupled device (CCD) detector (Princeton Instruments, PI-MTE, 2048 × 2048 pixels). The soft X-ray energies were tuned between 270 and 285 eV in the R-SoXS experiments. Using a custom Nika35 analysis package, 2D scattering patterns were reduced to 1D scattering profiles and normalized for the X-ray flux. Since distance traveled through the samples by the X-rays affects the scattering intensity, it was normalized for absorption and film thickness. Samples for R-SoXS measurements were first prepared on a polystyrene sulfonate (PSS) modified Si substrate and floating in water to a 1.5 × 1.5 mm, 100 nm thick Si3N4 membrane supported by a 5 × 5 mm, 200 μm thick Si frame.
2.2 Photoluminescence measurements
Steady-state photoluminescence spectra were obtained using an FL/FS920 from Edinburgh Instruments, equipped with an excitation source of a 450 W xenon arc lamp. In all cases, long pass sorting filters were utilized to remove unwanted higher energy light that passes through the excitation monochromator. To probe blends, an excitation wavelength of 685 nm was used. In order to normalize for the film thickness, the obtained spectra were normalized with the absorption at the excitation wavelength.
2.3 Device characterization
The J–V characterization was performed inside a nitrogen-filled glove box with a computer-controlled Keithley 2400 source meter, while the samples were exposed to a white light of 100 mW cm−2 intensity generated by an AM 1.5G solar simulator (Oriel Sol3A, Newport Corporation) that was calibrated to 1 sun with an NREL certified KG-5 filtered silicon reference diode. A PAIOS system (Fluxim) was used to perform the light intensity dependent photovoltaic measurements.
3. Results and discussion
The morphology of the all-small molecule bulk heterojunction blends was thoroughly investigated using a synchrotron radiation-based technique, R-SoXS. The mesoscale properties of the molecular scale domains will be discussed in detail. Furthermore, inverted all-small molecule solar cells were fabricated and characterized and their outputs are correlated with morphological properties. Overall, the results help to investigate the detailed morphology–function relationship, a key element that is needed for material and device optimization.
3.1 Multi-length scale morphology
R-SoXS aids in realizing the variation in the average composition which is directly related to device properties. Therefore, R-SoXS serves as a complementary technique to grazing-incidence wide-angle X-ray scattering to get a complete morphological picture at the mesoscale and to build morphology–device performance relationships.
It is noted that improper miscibility of the materials in the blend is one of the major factors that adversely affects the device performance of all-small molecule OSCs, and this morphological factor is highly controlled by the donor–acceptor intermolecular interactions;36,37 highly miscible molecules lead to the formation of too impure domains while low miscibility leads to large and over-purified domains hindering the charge dissociation process. In the current report, this challenging question is investigated by tuning the interactions between the molecules in the blend through side chain engineering and device processing. To explore the phase separation at different length scales and to assess the role of morphology on device performance, the R-SoXS experiment was performed at the beamline 11.0.1.2 at the Advanced Light Source (ALS).
In R-SoXS measurements, differences in the material refractive index are used to distinguish between materials,38 and the average composition variation is proportional to the square root of the integrated scattering intensity. Completely mixed domains provide a negligible signal. According to our previously established protocols,32 the integrated scattering intensity (ISI) of organic blend films can be used to quantify the relative domain purity, which was found to be a mostly linear relation with device fill factor. The long period is often correlated with the short-circuit current (Jsc).
R-SoXS profiles were acquired (Fig. 2a) near the absorption edge where high material contrast is achieved and Lorentz corrected.39 All the energies ranging from 283 eV to 284.2 eV show consistent results (Fig. S1, ESI†) with dual peak fitting, one lognormal peak at high q and a Gaussian peak at low q (Fig. 2c) to achieve good fits. This bimodal size distribution with two well-defined peaks is related to phase separation at two different length scales. This has been observed in all polymer OSC systems.40 A lognormal peak, in general, occurs in nature41 when a number of factors are contributing to phase separation while a Gaussian peak is attributable to control over a single variable.40Fig. 2b and d show the normalized integrated scattering intensity (ISI) and the component scattering intensity (CSI)42 of the high q domains for each blend, both as-cast and annealed. The corresponding scattering intensity of each peak component is obtained by integrating the scattering intensities of the fitted profile. In multiphase systems, the square root of the CSI is proportional to the domain purity of the particular domain. The normalized ISI and the CSI in the H11 system are greater than those of the H12 system for both the as-cast and annealed blends. The long period values of both domains, normalized ISI, and CSI values of the two domains for all the samples are summarized in Table 1.
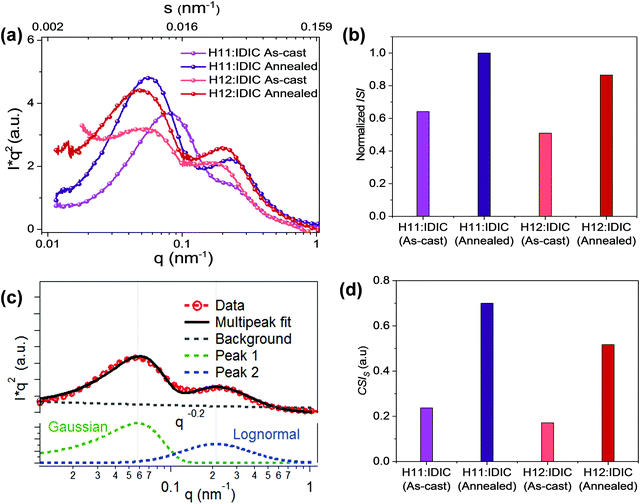 |
| Fig. 2 (a) Thickness/absorption normalized and Lorentz corrected R-SoXS profiles obtained at 283.6 eV. s is the spatial frequency, which can be transformed into momentum transfer with q = 2πs. (b) Plot of the ISI for various blend films. ISI normalized to the annealed H11:IDIC sample at 283.6 eV. (c) Multi-peak fit for the R-SoXS profile of the annealed H11:IDIC sample at 283.6 eV. Log-normal and Gaussian peaks are required to obtain a good fit. (d) Component scattering intensity (CSIS) of high q domains for each blend at 283.6 eV. | |
Table 1 Long period, total ISI, and CSI values for each peak at 283.6 eV
Sample |
Long period (nm) |
Normalized ISI |
CSI |
Low q peak |
High q peak |
Low q peak |
High q peak |
H11:IDIC (as-cast) |
83 |
34 |
0.64 |
0.26 |
0.37 |
H11:IDIC (annealed) |
108 |
29 |
1 |
0.3 |
0.7 |
H12:IDIC (as-cast) |
152 |
38 |
0.51 |
0.18 |
0.34 |
H12:IDIC (annealed) |
132 |
34 |
0.86 |
0.26 |
0.6 |
To confirm these morphological differences further in terms of domain purity, photoluminescence (PL) quenching studies were performed. The samples were prepared under the same processing conditions and the data obtained are normalized to material absorption at the excitation wavelength to eliminate the influence of the film thickness. The PL flux significantly increases with annealing compared to the as-cast blend (Fig. S3, ESI†). As a supplement to the reciprocal method, power spectral densities (PSD)43 of the AFM phase images (Fig. S4, ESI†), via the Fourier transform analysis were obtained to check the multi-length scale morphology of the blends. It confirms the existence of two domain sizes and the long periods of the domains also agree well with the value obtained from R-SoXS, which is around 0.15 nm−1 for the high-q peak and 0.03 nm−1 for the low-q peak. The AFM height image also shows the multi-length scale morphology which confirms that the bulk and surface morphologies are very similar.
3.2 Charge extraction and recombination
Excellent carrier generation and extraction efficiencies are crucial for achieving high efficiency in operational solar cells. These highly material-type and blend structure dependent processes need to be assessed and understood properly to develop material and device designs that enable the development of highly efficient OSCs. Here, to gain insight into the charge carrier generation dynamics of the all-small molecule solar cells, inverted devices with the ITO/ZnO/donor
:
acceptor (2
:
1, w/w)/MoO3/Al structure were fabricated and characterized (see Table 2). To probe charge generation dynamics, the photogenerated current (Jph) was measured by varying the device internal voltage (V0 = Vapplied − Vbuilt-in). Jph is estimated by subtracting the current measured in the dark from that measured in the light. Fig. 3a shows the Jph measured under illumination of one sun light intensity for both H11 and H12 based annealed devices. The Jph–V0 curves are characterized with varying slopes depending on the magnitude of V0. At a very low effective voltage (<0.2 V), Jph shows a linear dependence on voltage, while a square-root dependence is observed in the V0 range of 0.2–0.8 V originating from unbalanced electron and hole mobilities.44 The photocurrent saturates in a voltage range exceeding 0.8 V, and in this specific region the photocurrent is expressed as Jph = eGL,44,45 where e is the electric charge, G is the generation rate and L is the active layer thickness. The generation rate at the saturation region is 1.23 × 1027 m−3 s−1, and 8.26 × 1026 m−3 s−1 for the optimized H11 and H12 solar cells, respectively. Despite the similarities in backbone structure, these two materials have clearly resulted in a substantially different photocurrent yield in solar cells.
Table 2 Inverted device performance of H11 and H12 based inverted OSC devices
Device |
J
sc (mA cm−2) |
V
oc (V) |
FF (%) |
PCE (%) |
H11:IDIC as-cast |
8.7 ± 0.3 |
1.00 ± 0.01 |
36.3 ± 0.60 |
3.15 ± 0.13 |
H11:IDIC annealed |
13.8 ± 0.3 |
0.97 ± 0.01 |
59.5 ± 1.06 |
7.98 ± 0.21 |
H12:IDIC as-cast |
4.0 ± 0.2 |
0.89 ± 0.08 |
34.9 ± 1.37 |
1.25 ± 0.08 |
H12:IDIC annealed |
8.4 ± 0.4 |
0.87 ± 0.03 |
45.3 ± 0.45 |
3.30 ± 0.21 |
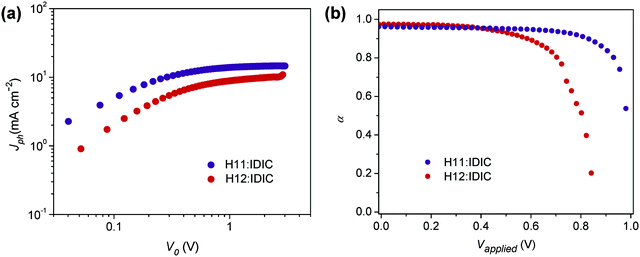 |
| Fig. 3 (a) Photocurrent (Jph) vs. effective applied voltage V0 under 1 sun illumination for annealed OSC devices; (b) α as a function of the voltage applied to the annealed OSC devices. | |
For further analysis of charge dynamics, Jph of the solar cells was measured at different light intensities and effective voltages (Fig. S2, ESI†). In this case, Jph is expected to have a power law dependence on light intensity Plight and should follow Jph ∝ Pαlight, where the exponent α may refer to charge collection or loss rates. Observing Fig. 3b, which shows α as a function of effective voltage, it can be seen that α assumes unity in the region corresponding to the saturation regime depicted in Fig. 3a, while its value drops dramatically near the open-circuit voltage (lower V0) indicating a strong bimolecular recombination in this region.46,47
It is also shown that α deviates from unity near open circuit voltage (Voc) more severely for the H12 system (Fig. S5, ESI†) than the H11-based devices, indicating that the former devices are subjected to more losses. The ideality factor for each system was also obtained at different light intensities (Fig. S6, ESI†). For devices measured under one sun illumination, the ideality factor was calculated to be 0.95 for the H11-based annealed solar cells indicating that losses at the open-circuit voltage are mostly bimolecular in nature. Corresponding H12-based devices are identified with an ideality factor of 1.49, which signals a strong effect of traps near the open-circuit voltage. The charge carrier generation and loss analyzed in this section will further be discussed in light of the observed morphological behaviors in the following section.
3.3 Fundamental connections and implications
The clear difference in the morphology of the two systems has clearly contributed to the photovoltaic properties. This section is dedicated to connecting the morphology–performance correlations to fully understand the role of side chain structures on device performance. In both systems, device parameters (FF and Jsc) increase upon annealing. The FF increases from 49.5% to 65.4% in the H11-based system and from 38.2% to 54.9% in the H12 based system. Jsc also shows a similar trend by increasing from 10.99 mA cm−2 to 15.21 mA cm−2 and from 4.94 mA cm−2 to 10.51 mA cm−2 for H11 and H12-based systems respectively in the conventional structure devices.31 The device performance parameters of inverted devices are provided in Table 2. It is clear that inverted and regular device configurations show consistent trends. According to the R-SoXS results summarized in Table 1, the ISI is increased upon annealing of each sample, which is indicative of domains getting purer48 and the long period of the smaller domains decreased over annealing which helps better charge dissociation. Therefore, the post-processing has optimized the phase seperation beteween the donor and the acceptor in these all small molecule systems, which is mostly the case in bulk-heterojunction solar cells. The size and purity of the nanoscale domains are important parameters in the charge generation and collection processes of OSCs. In the current study, we observed a clear linear correlation between the device fill factor (FF) and the ISI as depicted in Fig. 4a. Similarly, the FF was also found to be correlated with the component scattering intensity (CSI) of the high-q peak as shown in Fig. 4b, where the CSI describes the relative average composition variation of the two different length scales. Higher values indicate higher purity, which is beneficial for reducing bimolecular recombination. Such purer domains provide a longer pathway for the charge transport and increase device performance.
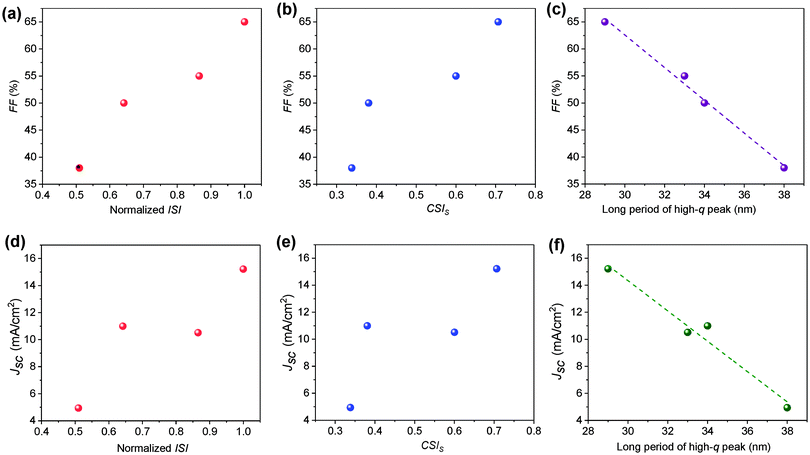 |
| Fig. 4 (a) Plot of the device FF as a function of the normalized ISI; (b) plot of the device FF as a function of the component scattering intensity of the high-q peak (CSIS); (c) plot of the device FF as a function of the long period of the high-q peak; (d) plot of Jsc as a function of the normalized ISI; (e) plot of Jsc as a function of the component scattering intensity of the high-q peak (CSIS); (f) plot of Jsc as a function of the long period of the high-q peak. Dashed lines represent linear fits to the raw data. | |
In this multi-length scale morphology, the length scale which contributes to charge dissociation at the donor–acceptor interface should mainly be attributed to the smaller domains, as the long period of the smaller domains is comparable to the typical exciton diffusion length of organic semiconductors. Thus, we see a clear correlation between the long period of the smaller domains and Jsc. Moreover, since the long period of the smaller domains is smaller for the H11-based blends (see Fig. 4c), better exciton diffusion and hence higher charge generation rate are expected in this particular system. This is indeed consistent with the highest charge carrier generation rate being achieved in the H11 system from the current–voltage characteristics discussed in Section 3.2. To have a deeper understanding of the role of the morphology on photocurrent generation, Jsc is depicted against domain properties as shown in Fig. 4d–f. In particular, Jsc was found to be linearly decreasing with the domain size (Fig. 4f), which is an indication of either less exciton dissociation in larger domains or poor charge carrier collection to electrodes. The H12-based system, which is identified by a less pure and larger domain size, obviously suffers from a smaller FF, a lower generation rate and hence a lower Jsc. As noted earlier, Flory–Huggins interaction parameters calculated from the solubility parameters for these two systems have shown that the H11:IDIC blend has a lower molecular miscibility. The estimated interaction parameter values are 2.52 and 2.35 for H11:IDIC and H12:IDIC systems respectively.31 A higher value for the interaction parameter indicates lower miscibility and hence higher purity. This observation clearly agrees with the domain purities obtained via R-SoXS, although it should be noted that estimates from solubility parameters are not always reliable.
Achieving purer domains after annealing was further confirmed by photoluminescence (PL) measurements. The comparison of the as-cast blend and annealed blend PL spectra (Fig. S3, ESI†) shows a clear reduction in the intensity in the as-cast samples compared to the annealed ones. This can be explained as the excitons in the as-cast blends find an interface to dissociate immediately. On the other hand, the increase in the PL intensity in the annealed samples indicates that the singlet excitons are not splitting efficiently as they radiatively recombine in the pure phase. This confirms our finding that R-SoXS domains get purer when annealed. It is also clear from the device parameters that annealing devices is critical to improving the device performance. This indicates the importance of achieving more pure and smaller domains for improved device performance. At the same time, the combined results indicate that the morphology is not yet optimized.
4. Conclusion
In this study, we applied R-SoXS to investigate the relationship between complex morphology and device performance in a pair of nonfullerene all-small molecule systems, which differ in the conjugation of the side chains. A multi-length scale morphology was observed in the blends of H11 and H12 with IDIC, and the high-q long period, as well as the composition variations, correlate well with Jsc and FF. FF and Jsc monotonically increase with the CSI of the high-q domains and ISI while decreasing almost linearly with the high-q long period. Notably, this is the first report that shows such a strong correlation between device parameters and morphological parameters at the smaller length scale in nonfullerene all-small molecule OSCs. The ideality factor of the devices with the H12 blend shows higher deviation from unity and low α values at higher light intensities which are attributed to the inefficiencies in charge collection due to recombination. Higher domain purity and small domain sizes in the H11 system reduce recombination and help charge dissociation respectively. Since the only difference between H11 and H12 is the conjugation of the side chains, side chain modification of a small molecule can create a tremendous effect on device performance by altering the molecular interaction and the multilength scale morphology. The system studied in this study is a clear example to show the ability of side chain modification to improve device performance and the importance of an understanding of structure–functional relations for functional blends based on nonfullerene small molecules. We anticipate that developing in situ R-SoXS will enable our understanding of the real-time evolution of the complex morphology of this type of emerging solar cell.
Conflicts of interest
There are no conflicts to declare.
Acknowledgements
The authors gratefully acknowledge the support from the U.S. Office of Naval Research (ONR, grant No. N000141712204) and the UNC General Administration Research Opportunity Initiative grant. Y. Li was supported by the Ministry of Science and Technology of China (973 project, No. 2014CB643501) and the Strategic Priority Research Program of the Chinese Academy of Sciences, Grant No. XDB12030200. X-ray data were acquired at beamline 11.0.1.2 at the Advanced Light Source, which is supported by the Director, Office of Science, Office of Basic Energy Sciences, of the U.S. Department of Energy under contract no. DE-AC02-05CH11231. C. Wang of the ALS (DOE) is acknowledged for assisting with the experimental setup and providing instrument maintenance and E. Danilov is acknowledged for maintaining the shared PL facility at the NCSU.
References
- S. Li, L. Ye, W. Zhao, H. Yan, B. Yang, D. Liu, W. Li, H. Ade and J. Hou, J. Am. Chem. Soc., 2018, 140, 7159–7167 CrossRef CAS PubMed
.
- L. Meng, Y. Zhang, X. Wan, C. Li, X. Zhang, Y. Wang, X. Ke, Z. Xiao, L. Ding, R. Xia, H. L. Yip, Y. Cao and Y. Chen, Science, 2018, 361, 1094–1098 CrossRef CAS PubMed
.
- J. Q. Zhang, H. S. Tan, X. G. Guo, A. Facchetti and H. Yan, Nat. Energy, 2018, 3, 720–731 CrossRef CAS
.
- C. Yan, S. Barlow, Z. Wang, H. Yan, A. K. Y. Jen, S. R. Marder and X. Zhan, Nat. Rev. Mater., 2018, 3, 18003 CrossRef CAS
.
- C. Sun, F. Pan, H. Bin, J. Zhang, L. Xue, B. Qiu, Z. Wei, Z.-G. Zhang and Y. Li, Nat. Commun., 2018, 9, 743 CrossRef PubMed
.
- D. Deng, Y. Zhang, J. Zhang, Z. Wang, L. Zhu, J. Fang, B. Xia, Z. Wang, K. Lu, W. Ma and Z. Wei, Nat. Commun., 2016, 7, 13740 CrossRef CAS PubMed
.
- J. E. Coughlin, Z. B. Henson, G. C. Welch and G. C. Bazan, Acc. Chem. Res., 2014, 47, 257–270 CrossRef CAS PubMed
.
- C. Cui, X. Guo, J. Min, B. Guo, X. Cheng, M. Zhang, C. J. Brabec and Y. Li, Adv. Mater., 2015, 27, 7469–7475 CrossRef CAS PubMed
.
- B. Qiu, L. Xue, Y. Yang, H. Bin, Y. Zhang, C. Zhang, M. Xiao, K. Park, W. Morrison, Z.-G. Zhang and Y. Li, Chem. Mater., 2017, 29, 7543–7553 CrossRef CAS
.
- H. Li, Y. Zhao, J. Fang, X. Zhu, B. Xia, K. Lu, Z. Wang, J. Zhang, X. Guo and Z. Wei, Adv. Energy Mater., 2018, 8, 1702377 CrossRef
.
- H. Bin, J. Yao, Y. Yang, I. Angunawela, C. Sun, L. Gao, L. Ye, B. Qiu, L. Xue, C. Zhu, C. Yang, Z.-G. Zhang, H. Ade and Y. Li, Adv. Mater., 2018, 30, 1706361 CrossRef PubMed
.
- J. Guo, H. Bin, W. Wang, B. Chen, J. Guo, R. Sun, Z.-G. Zhang, X. Jiao, Y. Li and J. Min, J. Mater. Chem. A, 2018, 6, 15675–15683 RSC
.
- M. Privado, P. de la Cruz, S. Biswas, R. Singhal, G. D. Sharma and F. Langa, J. Mater. Chem. A, 2018, 6, 11714–11724 RSC
.
- L. Yang, S. Zhang, C. He, J. Zhang, Y. Yang, J. Zhu, Y. Cui, W. Zhao, H. Zhang, Y. Zhang, Z. Wei and J. Hou, Chem. Mater., 2018, 30, 2129–2134 CrossRef CAS
.
- Y. Wang, M. Chang, B. Kan, X. Wan, C. Li and Y. Chen, ACS Appl. Energy Mater., 2018, 1, 2150–2156 CrossRef CAS
.
- N. Liang, D. Meng, Z. Ma, B. Kan, X. Meng, Z. Zheng, W. Jiang, Y. Li, X. Wan, J. Hou, W. Ma, Y. Chen and Z. Wang, Adv. Energy Mater., 2016, 7, 1601664 CrossRef
.
- O. K. Kwon, J.-H. Park, D. W. Kim, S. K. Park and S. Y. Park, Adv. Mater., 2015, 27, 1951–1956 CrossRef CAS PubMed
.
- L. Yang, S. Zhang, C. He, J. Zhang, H. Yao, Y. Yang, Y. Zhang, W. Zhao and J. Hou, J. Am. Chem. Soc., 2017, 139, 1958–1966 CrossRef CAS PubMed
.
- Z. Zhou, S. Xu, J. Song, Y. Jin, Q. Yue, Y. Qian, F. Liu, F. Zhang and X. Zhu, Nat. Energy, 2018, 3, 952–959 CrossRef CAS
.
- J. Shi, A. Isakova, A. Abudulimu, M. van den Berg, O. K. Kwon, A. J. Meixner, S. Y. Park, D. Zhang, J. Gierschner and L. Lüer, Energy Environ. Sci., 2018, 11, 211–220 RSC
.
- J. Hong, M. J. Sung, H. Cha, C. E. Park, J. R. Durrant, T. K. An, Y.-H. Kim and S.-K. Kwon, ACS Appl. Mater. Interfaces, 2018, 10, 36037–36046 CrossRef CAS PubMed
.
- G. Han and Y. Yi, Adv. Theory Simul., 2018, 1800091 CrossRef
.
- G. Long, B. Wu, A. Solanki, X. Yang, B. Kan, X. Liu, D. Wu, Z. Xu, W.-R. Wu, U. S. Jeng, J. Lin, M. Li, Y. Wang, X. Wan, T. C. Sum and Y. Chen, Adv. Energy Mater., 2016, 6, 1600961 CrossRef
.
- O. Alqahtani, M. Babics, J. Gorenflot, V. Savikhin, T. Ferron, A. Balawi, A. Paulke, Z. Kan, M. Pope, A. J. Clulow, J. Wolf, P. L. Burn, G. Ian, N. Dieter, M. F. Toney, F. Laquai, P. M. Beaujuge and B. A. Collins, Adv. Energy Mater., 2018, 8, 1702941 CrossRef
.
- J. Min, X. C. Jiao, I. Ata, A. Osvet, T. Ameri, P. Bauerle, H. Ade and C. J. Brabec, Adv. Energy Mater., 2016, 6, 1502579 CrossRef
.
- K. Gao, J. Miao, L. Xiao, W. Deng, Y. Kan, T. Liang, C. Wang, F. Huang, J. Peng, Y. Cao, F. Liu, T. P. Russell, H. Wu and X. Peng, Adv. Mater., 2016, 28, 4727–4733 CrossRef CAS PubMed
.
- C. He and J. Hou, Acta Phys. –Chim. Sin., 2018, 34, 1202–1210 Search PubMed
.
- Y. Li, Acc. Chem. Res., 2012, 45, 723–733 CrossRef CAS PubMed
.
- L. Ye, S. Zhang, L. Huo, M. Zhang and J. Hou, Acc. Chem. Res., 2014, 47, 1595–1603 CrossRef CAS PubMed
.
- Y. Chen, X. Wan and G. Long, Acc. Chem. Res., 2013, 46, 2645–2655 CrossRef CAS PubMed
.
- H. Bin, Y. Yang, Z. G. Zhang, L. Ye, M. Ghasemi, S. Chen, Y. Zhang, C. Zhang, C. Sun, L. Xue, C. Yang, H. Ade and Y. Li, J. Am. Chem. Soc., 2017, 139, 5085–5094 CrossRef CAS PubMed
.
- X. Jiao, L. Ye and H. Ade, Adv. Energy Mater., 2017, 7, 1700084 CrossRef
.
- E. Gann, A. T. Young, B. A. Collins, H. Yan, J. Nasiatka, H. A. Padmore, H. Ade, A. Hexemer and C. Wang, Rev. Sci. Instrum., 2012, 83, 045110 CrossRef CAS PubMed
.
- F. Liu, M. A. Brady and C. Wang, Eur. Polym. J., 2016, 81, 555–568 CrossRef CAS
.
- J. Ilavsky, J. Appl. Crystallogr., 2012, 45, 324–328 CrossRef CAS
.
- L. Ye, B. A. Collins, X. Jiao, J. Zhao, H. Yan and H. Ade, Adv. Energy Mater., 2018, 8, 1703058 CrossRef
.
- L. Ye, H. Hu, M. Ghasemi, T. Wang, B. A. Collins, J.-H. Kim, K. Jiang, J. H. Carpenter, H. Li, Z. Li, T. McAfee, J. Zhao, X. Chen, J. L. Y. Lai, T. Ma, J.-L. Bredas, H. Yan and H. Ade, Nat. Mater., 2018, 17, 253–260 CrossRef CAS PubMed
.
- J. H. Carpenter, A. Hunt and H. Ade, J. Electron Spectrosc. Relat. Phenom., 2015, 200, 2–14 CrossRef CAS
.
- F. Cser, J. Appl. Polym. Sci., 2001, 80, 2300–2308 CrossRef CAS
.
- L. Ye, Y. Xiong, S. Li, M. Ghasemi, N. Balar, J. Turner, A. Gadisa, J. Hou, B. T. O'Connor and H. Ade, Adv. Funct. Mater., 2017, 27, 1702016 CrossRef
.
- E. Limpert, W. A. Stahel and M. Abbt, BioScience, 2001, 51, 341–352 CrossRef
.
- S. Mukherjee, X. Jiao and H. Ade, Adv. Energy Mater., 2016, 6, 1600699 CrossRef
.
- L. Ye, Y. Xiong, H. Yao, A. Gadisa, H. Zhang, S. Li, M. Ghasemi, N. Balar, A. Hunt, B. T. O’Connor, J. Hou and H. Ade, Chem. Mater., 2016, 28, 7451–7458 CrossRef CAS
.
- V. D. Mihailetchi, J. Wildeman and P. W. M. Blom, Phys. Rev. Lett., 2005, 94, 126602 CrossRef CAS PubMed
.
- R. Sokel and R. C. Hughes, J. Appl. Phys., 1982, 53, 7414–7424 CrossRef CAS
.
- S. R. Cowan, A. Roy and A. J. Heeger, Phys. Rev. B: Condens. Matter Mater. Phys., 2010, 82, 245207 CrossRef
.
- A. C. Stuart, J. R. Tumbleston, H. Zhou, W. Li, S. Liu, H. Ade and W. You, J. Am. Chem. Soc., 2013, 135, 1806–1815 CrossRef CAS PubMed
.
- X. Y. Liu, L. Ye, W. C. Zhao, S. Q. Zhang, S. S. Li, G. M. Su, C. Wang, H. Ade and J. H. Hou, Mater. Chem. Front., 2017, 1, 2057–2064 RSC
.
Footnote |
† Electronic supplementary information (ESI) available. See DOI: 10.1039/c8qm00503f |
|
This journal is © the Partner Organisations 2019 |