DOI:
10.1039/C9NR08454A
(Paper)
Nanoscale, 2020,
12, 210-219
Palladium nanosheet-knotted injectable hydrogels formed via palladium–sulfur bonding for synergistic chemo-photothermal therapy†
Received
1st October 2019
, Accepted 7th November 2019
First published on 7th November 2019
Abstract
Nanoparticle (NP)-based hydrogels that can introduce synergistic advantages to the novel three-dimensional scaffold have garnered much attention recently. However, the application of NP-crosslinked hydrogels still remains challenging due to the complicated synthesis and/or modification of the NPs and the changed properties of the NPs after gelation. Herein, a novel palladium nanosheet (Pd NS)-based hydrogel (Pd Gel) with Pd NSs as crosslinkers was obtained by simply mixing Pd NSs with thiol-terminated four-arm polyethylene glycol (4arm-PEG-thiol). It was found that the formed Pd Gel was injectable, possibly due to the dynamic Pd–S bonds formed between Pd NSs and 4arm-PEG-thiol. In addition, compared with free Pd NSs, the Pd NSs within the hydrogel exhibited a significantly higher stability. We have further demonstrated that the formed hydrogel could encapsulate the commonly used anticancer drug doxorubicin (DOX) to form DOX@Pd Gel for combined chemo-photothermal therapy. Particularly, Pd NSs with a high absorption in the near-infrared (NIR) region could convert the energy of NIR laser into heat with a high efficiency, which is beneficial for photothermal therapy. Moreover, DOX@Pd Gel could maintain a sustainable release of DOX and the NIR laser irradiation could accelerate this drug release process. Then, the explosively released DOX and the hyperthermia generated from Pd NSs under NIR laser irradiation acted in a synergistic way to realize the combined therapeutic effect of the chemo-photothermal treatment. Finally, the in vivo anticancer effect and safety of the combined therapy were also verified by the tumor-bearing mouse model. Taken together, this work constructs a NP-crosslinked, NIR laser-activatable and injectable photothermal hydrogel via dynamic Pd–S bonding, and demonstrates that the hydrogel allows us to release DOX more precisely, eliminate tumor more effectively and inhibit tumor metastasis more persistently, which will advance the development of novel anticancer strategies.
Introduction
Hydrogels, the three-dimensional water-swollen polymer networks, have been widely used in various aspects such as sensing applications, diagnostic devices, quantitative detection, drug delivery, cell culture and tissue engineering.1–6 Nanoparticle (NP)–hydrogel composites, which innovatively combine two entirely different types of materials, are thought to not only generate structural diversity but also achieve significant enhancements of a variety of properties such as tissue adhesive property, mechanical stiffness and bioactivity. Besides, this combination may lead to a synergistic property improvement for each component including the increased mechanical strength of the hydrogel and the decreased aggregation of the NPs.7 However, in most studies, NPs are simply dispersed in the reservoir of hydrogels; few studies have prepared NP-based hydrogels in which NPs act as crosslinking knots to form the hydrogels. Nonetheless, it has been reported that crosslinking using NPs provides multiple bonds within the gel networks,7 which makes the hydrogel formation more convenient compared with traditional hydrogel formation reactions. In addition, localized chemotherapy has been widely used for different cancer treatments.8,9 Recently, there has been increasing interest in designing hydrogels as a scaffold for local anticancer drug delivery to realize localized chemotherapy because of their capability to maintain high drug concentrations at the focal area over an extended time period.10–13 However, there are still challenges for researchers to design hydrogel systems with the controlled and consistent drug release property to avoid the fast release of drugs via free diffusion or hydrolytic gel degradation for localized chemotherapy. Meanwhile, controlled drug release can be realized in stimulus-responsive systems under the trigger of either endogenous stimuli including pH, enzymes and redox gradients,14–18 or exogenous ones such as near-infrared (NIR) light, temperature and ultrasound.9,19,20 Among these stimuli, NIR light is an attractive stimulus because it can be regulated remotely and controlled accurately with deep tissue penetration.21 Therefore, it is promising to integrate the stimulus-responsive components into hydrogels for designing smart anticancer systems, which can trigger the release of anticancer drugs under the specific stimuli in an on-demand and spatio-temporally controllable way.
Recently, two-dimensional (2D) palladium nanosheets (Pd NSs) have emerged as a promising candidate for cancer treatment due to the following advantages: (1) strong optical absorption in the NIR region and high photothermal conversion efficiency at 808 nm (52.0%),22 (2) outstanding photothermal stability,23 (3) ease of surface modification through palladium–sulfur (Pd–S) bonding,24 and (4) excellent biocompatibility both in vitro and in vivo.25 However, the prepared Pd NSs usually require further modification with polyvinyl pyrrolidone (PVP) or polyethylene glycol (PEG) to maintain their stability. On the other hand, photothermal therapy (PTT) has been developed as a promising strategy that usually employs light-absorbing agents to generate local hyperthermia to ablate tumour cells with a higher selectivity and fewer side effects than the conventional radiotherapy and chemotherapy.26,27 Meanwhile, the effectiveness of any single therapy in realizing ideal therapeutic effect is usually limited.28 Combined therapy, which integrates the advantages of various therapies, is considered as a promising strategy to improve cancer treatment outcomes and minimize side effects.29–31 Among various combined therapies, the combination of PTT with many other treatment modalities such as chemotherapy, photodynamic therapy, gene therapy and immunotherapy have attracted tremendous attention in recent years.32–42 Moreover, hyperthermia can also enhance the sensitivity of cells to chemotherapeutic drugs.43 Collectively, developing a novel NP-based hydrogel system with the ability of NIR laser-controllable drug release to realize combined chemo-photothermal therapy is highly desirable for achieving satisfactory anticancer therapeutic outcomes.
Here, we report the facile preparation of a novel Pd NS-knotted hydrogel. Pd NSs were prepared by simply mixing a dinuclear PdI carbonyl chloride complex with water according to a previous report,44 and were then mixed with 4arm-PEG-thiol to form the hydrogel (abbreviated as Pd Gel) via Pd–S bonding. Different from those traditional NP-based hydrogels, in which the NPs are just attached somewhere on the strands of the hydrogel network, the NP-knotted hydrogel prepared in this work avoids the use of additional crosslinking reagents during gelation and utilizes instead the NPs themselves as the only crosslinkers. Besides, such a preparation method ensures that the NPs are stably linked in the hydrogel, which prevents them from diffusing out of the hydrogel, avoiding potential toxic effects of the NPs if they are used in biomedical applications. Moreover, the common methods which use NPs as crosslinkers usually require appropriate surface functionalization of the NPs; however, the Pd NSs in this work can be crosslinked with thiol-terminated PEG directly through Pd–S bonding, leading to the convenient preparation and good injectability of the formed Pd Gel. Furthermore, doxorubicin (DOX), a common anticancer drug, was introduced into the above system to form the DOX-containing hydrogel (termed DOX@Pd Gel). On the one hand, the as-formed Pd Gel was injectable (which possibly reflected the dynamic nature of the Pd–S bonding) and significantly improved the stability of Pd NSs, which is beneficial for the intratumoral administration and photothermal conversion performance of the Pd Gel. On the other hand, NIR laser irradiation could not only activate the photothermal effect of Pd NSs, but also accelerate the DOX release from the DOX@Pd Gel, both of which acted in a synergistic way to realize the combined therapeutic effect of the chemo-photothermal treatment, and the superior synergistic anticancer performance was also verified in in vitro experiments and in vivo assays (Scheme 1). This work develops a very simple strategy to prepare a novel Pd NS-knotted hydrogel, which holds great promise in combination therapy of cancer.
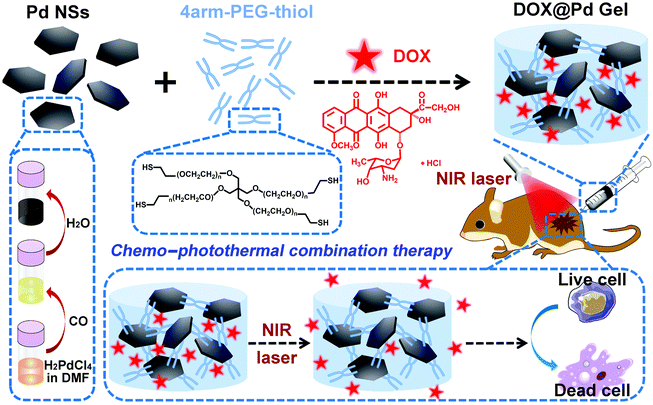 |
| Scheme 1 Schematic illustration of the preparation of DOX@Pd Gel and the anticancer capacity of combined chemo-photothermal therapy. | |
Results and discussion
The gelation was confirmed through a vial tilting method. Different from the fluid Pd NS dispersion (Fig. 1a, I) and 4arm-PEG-thiol solution (Fig. 1a, II), no flow or significant movement of the Pd Gel (Fig. 1a, III) and DOX@Pd Gel (Fig. 1a, IV) was observed in the two vials, indicating the successful preparation of hydrogels. In addition, the dark-blue color of the Pd Gel (Fig. 1a, III) was similar to that of Pd NS dispersion (Fig. 1a, I) due to the presence of the component Pd NSs. Besides, compared with the unchanged state of the Pd Gel after storage at 4 °C for 1 week, the aggregation of bare Pd NSs within 1 day and the aggregation of PEG-modified Pd NSs (PEG-Pd NSs) within 1 week indicated the increased stability of Pd NSs in the Pd Gel (Fig. S1†). Scanning electron microscopy (SEM) images of the DOX@Pd Gel revealed the well-defined porous structure of the hydrogel (Fig. 1b). Besides, the DOX@Pd Gel could be easily ejected through a syringe to form the characters “GEL” on the glass substrate without clogging (Fig. 1c). Furthermore, the thermal image showed the elevated temperature of the DOX@Pd Gel after NIR laser irradiation (808 nm, 0.6 W cm−2, 10 min) (Fig. 1d), indicating that the DOX@Pd Gel possessed an excellent photothermal conversion capability, which was attributed to the presence of the photothermal nanoagents Pd NSs in the hydrogel. To investigate the formation mechanism of the Pd Gel, Fourier transform infrared (FTIR) spectroscopy was used to characterize the solid powders of 4arm-PEG-thiol and the Pd Gel, and the results in Fig. 1e showed that the peak at ∼529 cm−1, which can be assigned to the stretching vibration of the disulfide (S–S) bonds,45,46 underwent a decline (using the intensity of the C–C stretching vibration peak residing at ∼842 cm−1 as an internal reference) when 4arm-PEG-thiol (and Pd NSs) formed the Pd Gel, indicating the cleavage of S−S bonds and the possible formation of Pd−S bonds during the gelation. More importantly, in the high-resolution X-ray photoelectron spectroscopy (XPS) curve of S 2p in Fig. 1f, we observed a peak at 162.2 eV, which is attributed to metal–sulfur bonds,47–49 indicating the successful conjugation between the Pd NSs and 4arm-PEG-thiol via the Pd–S bonding. Additionally, in the high-resolution Pd 3d curve (Fig. 1g), the two peaks at 335.1 and 340.4 eV can be attributed to Pd(0), and the two peaks at 337.2 and 342.4 eV suggest the presence of Pd(II),25,47,50–52 indicating that a small portion of Pd atoms (possibly in the outer layer of Pd NSs) were oxidized by the thiol groups of 4arm-PEG-thiol to form Pd–S bonds. Besides, the failure of the “methoxyl-terminated four-arm polyethylene glycol (4arm-PEG) + Pd NSs” mixture and 4arm-PEG-thiol itself in forming hydrogels demonstrated the indispensable roles of the thiol groups and the Pd NSs in gelation (Fig. S2†). We have also characterized the viscoelastic properties of the Pd Gel and the DOX@Pd Gel via rheological measurements. The decrease in the viscosity of the Pd Gel versus the shear rate demonstrated the shear thinning behavior in the hydrogel (Fig. 1h), indicating that the hybrid aggregates within the hydrogel were disrupted by the shear forces. This phenomenon also means that the state of the hydrogel can be changed through strong forces (e.g., the forces generated during the injection treatments),6 in accordance with the result of Fig. 1c, which may be due to the relatively weak Pd–S bonds. Moreover, compared with the loss modulus (G′′) values of the Pd Gel, the higher values of the storage modulus (G′) showed the good mechanical stability of the Pd Gel (Fig. 1i), which may be beneficial for prolonging the retention time of the hydrogel in the tumor region. For the DOX@Pd Gel, a similar tendency to the Pd Gel can be observed from Fig. 1h and j, indicating that the introduction of DOX induced negligible changes of the resultant DOX@Pd Gel as compared with the DOX-free Pd Gel. In addition, the photothermal effects of the Pd Gel and the DOX@Pd Gel were monitored through a thermal imaging camera (Fig. 1k); a temperature elevation of ∼11 °C for both hydrogels indicated that the introduction of DOX into the Pd Gel also did not affect the photothermal effect of the resultant DOX@Pd Gel. Furthermore, the unchanged temperature elevation of the DOX@Pd Gel after 3 “on/off” cycles of NIR laser irradiation (Fig. 1l) demonstrated the good photostability of the DOX@Pd Gel, which makes it suitable for repeated treatments. Furthermore, as shown in Fig. 1m, the release of DOX from the DOX@Pd Gel was significantly accelerated under the NIR laser irradiation, while only a small portion of DOX molecules were released from the DOX@Pd Gel without irradiation. This accelerated release of DOX from the DOX@Pd Gel under laser irradiation can be attributed to the laser-induced local hyperthermia of the Pd NSs in the DOX@Pd Gel, indicating that the DOX@Pd Gel can be utilized for the on-demand release of DOX molecules.
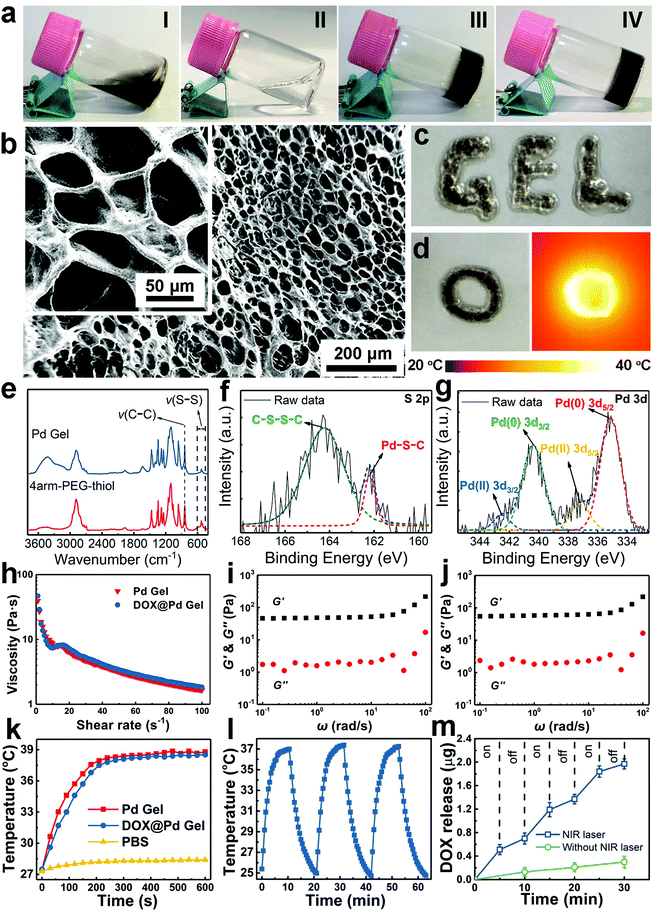 |
| Fig. 1 Characterization of DOX@Pd Gel. (a) Photographs of Pd NS aqueous dispersion (I), 4arm-PEG-thiol solution (II), Pd Gel (III) and DOX@Pd Gel (IV). (b) Typical SEM images of DOX@Pd Gel. (c) “GEL” made of DOX@Pd Gel written by a syringe on a glass slide. (d) Photograph of the circle made of DOX@Pd Gel and corresponding thermal image taken after laser irradiation (808 nm, 0.6 W cm−2, 10 min). (e) FTIR spectra of 4arm-PEG-thiol and Pd Gel dry powders. High-resolution XPS curves of (f) S 2p and (g) Pd 3d of Pd Gel. (h) Viscosity of Pd Gel or DOX@Pd Gel versus shear rate. Storage modulus (G′) and loss modulus (G′′) of Pd Gel (i) or DOX@Pd Gel (j) versus frequency (ω). (k) Temperature changes of Pd Gel, DOX@Pd Gel and phosphate-buffered saline (PBS) under continuous irradiation by an 808 nm laser (0.6 W cm−2). (l) Temperature changes of DOX@Pd Gel during the 3 “on/off” cycles of laser irradiation (808 nm, 0.6 W cm−2, 10 min for each “laser-on” duration). (m) Effect of NIR laser on the release of DOX from DOX@Pd Gel. NIR laser: 808 nm, 0.6 W cm−2, 5 min for each irradiation duration. | |
To study the cytotoxicity of the DOX@Pd Gel, the methylthiazolyldiphenyl-tetrazolium bromide (MTT) assay was conducted using murine breast cancer cells (4T1 cells). According to the results in Fig. 2a, the 100% lixivium from the DOX@Pd Gel elicited similar toxicity to 4T1 cells compared with ∼1 μg mL−1 free DOX. Moreover, results in Fig. 2b showed the enhanced anticancer effect of the DOX@Pd Gel with NIR laser irradiation compared with the “DOX + laser” and “Pd Gel + laser” groups, suggesting that the combination of chemotherapy and PTT has better anticancer performance than single therapy.
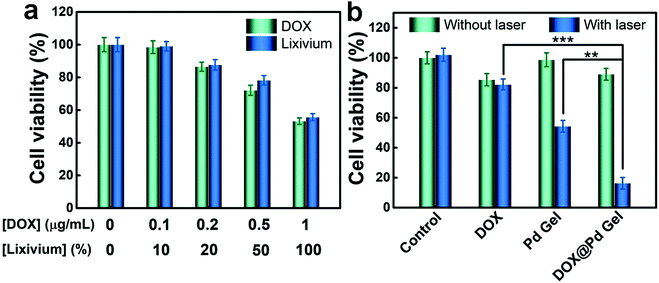 |
| Fig. 2 (a) Viability evaluation results of 4T1 cells after treatment with different concentrations of DOX or lixivium. (b) Viability evaluation results of 4T1 cells after receiving different treatments. Control: untreated, DOX: 1 μg mL−1, Pd Gel: 60 μg mL−1 of Pd, DOX@Pd Gel: 1 μg mL−1 of DOX and 60 μg mL−1 of Pd and laser irradiation: 808 nm, 0.6 W cm−2, 10 min. Error bars indicate ± S.D. (n = 3). ** and *** indicate statistically significant differences at P < 0.01 and P < 0.001, respectively. | |
To intuitively observe the phototoxicity caused by various treatments, the live/dead staining assay was carried out. Specifically, the 4T1 cells after different treatments were stained with calcein acetoxymethyl ester (calcein-AM) and propidium iodide (PI). The live cells were stained green with calcein-AM and the dead ones were stained red with PI. The confocal imaging results in Fig. 3a showed that almost all the cells were stained green in the “control”, “laser”, or “Pd Gel” groups. In addition, both green and red fluorescence could be observed in the cells after DOX, “DOX + laser”, “Pd Gel + laser”, or DOX@Pd Gel treatment, suggesting their insufficient cancer cell killing performance. In contrast, negligible green fluorescence could be observed in the “DOX@Pd Gel + laser” group and all the cells were stained red with PI, demonstrating the markedly enhanced ability of “DOX@Pd Gel + laser” in killing cancer cells due to the combined chemo-photothermal treatment. Considering that reactive oxygen species (ROS) generation can also lead to oxidative stress and apoptosis in different types of cells,53 an ROS detection kit was used to quantify the ROS levels within 4T1 cells after different treatments. In this ROS detection assay, a stronger cellular fluorescence intensity indicates enhanced cellular ROS generation.54 The results in Fig. 3b, c and S3† indicated that the cellular ROS level in the “DOX@Pd Gel + laser” group was significantly up-regulated compared with that in other groups. Furthermore, the apoptosis induced by the DOX@Pd Gel-mediated combined therapy was also evaluated using the annexin V-fluorescein isothiocyanate (FITC)/PI staining assay and flow cytometry. The data in Fig. 3d demonstrated that compared with the “control”, “laser”, “DOX”, “DOX + laser”, “Pd Gel”, “Pd Gel + laser” and “DOX@Pd Gel” groups, the lowest rate of healthy cells (20.78%) was observed in the “DOX@Pd Gel + laser” group. In addition, the flow cytometric results indicated that DOX@Pd Gel plus NIR laser irradiation induced cell death mainly through apoptosis/necrosis (78.62%).
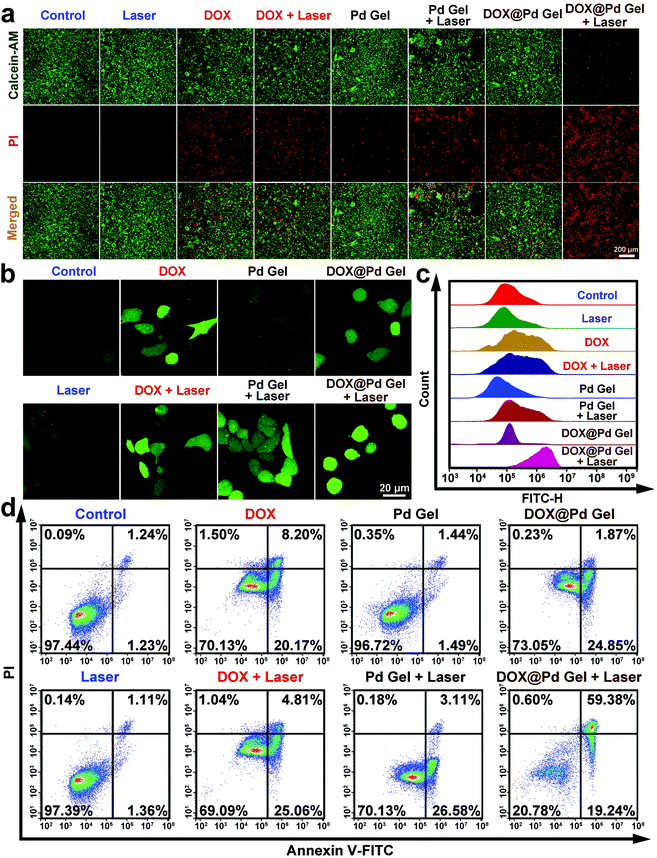 |
| Fig. 3 (a) Live/dead staining results of 4T1 cells left untreated (control) or subjected to various treatments. Laser: 808 nm, 0.6 W cm−2, 10 min. (b) Confocal microscopic and (c) flow cytometric evaluation results of ROS generation in 4T1 cells subjected to various treatments. (d) Flow cytometry analysis results of annexin V-FITC/PI-stained 4T1 cells subjected to various treatments. | |
Encouraged by the excellent in vitro anticancer performance of the DOX@Pd Gel plus laser irradiation, we carried out in vivo experiments using 4T1 tumor-bearing mice as the animal models. The mice were grouped (n = 6) and subjected to the following different treatments: control (untreated), laser, DOX, “DOX + laser”, Pd Gel, “Pd Gel + laser”, DOX@Pd Gel, or “DOX@Pd Gel + laser” (laser: 808 nm, 0.6 W cm−2, 10 min). The relative tumor volumes in Fig. 4a showed that the “DOX@Pd Gel + laser” treatment could completely inhibit the tumor growth and even eliminate the solid tumors, while neither the DOX@Pd Gel nor “Pd Gel + laser” could completely inhibit the tumor growth, demonstrating the excellent antitumor performance of the combined chemo-photothermal therapy. In addition, negligible influence was observed in the body weights of the “DOX@Pd Gel + laser” treated mice (Fig. 4b), indicating the good biocompatibility of the combined treatment. Moreover, the hematoxylin and eosin (H&E) staining results of tumor slices from mice subjected to different treatments are shown in Fig. 4c, in which the dense structures could be seen in the “laser”, “DOX + laser” and “Pd Gel + laser” groups, while the tumor tissues from mice after “DOX@Pd Gel + laser” treatment elicited extensive necrosis, which can be attributed to the cell lysis induced by the released DOX and hyperthermia caused by Pd NSs under NIR laser irradiation.
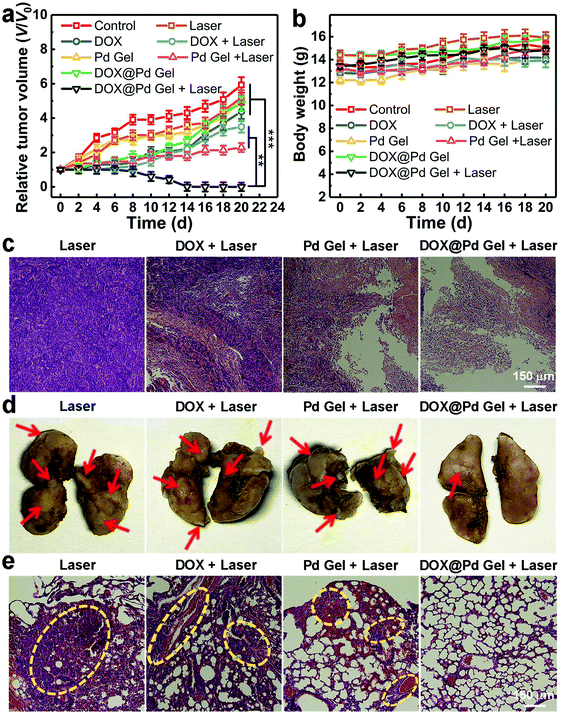 |
| Fig. 4
In vivo experiments. (a) Relative tumor volumes and (b) body weights of 4T1 tumor-bearing mice subjected to various treatments as indicated. Error bars indicate ± S.D. (n = 6). ** and *** indicate statistically significant differences at P < 0.01 and P < 0.001, respectively. (c) H&E staining results of the tumor tissues separated from representative mice subjected to different treatments as indicated. (d) Photographs of Bouin's solution-stained lungs and (e) H&E staining results of the lungs from representative mice subjected to different treatments as indicated. | |
Interestingly, apart from the notable performance of “DOX@Pd Gel + laser” in eliminating solid tumors, the DOX@Pd Gel-mediated combined therapy was also effective at inhibiting the tumor metastasis. Specifically, the lungs separated from mice in each group at the end of the experiments were fixed with Bouin's solutions. The lungs can be stained yellow except the metastatic nodules. As shown in Fig. 4d, decreased nodules in the lungs from the “DOX@Pd Gel + laser” group can be observed compared with the other groups, indicating the successful inhibition of tumor metastasis in lungs. Furthermore, according to the H&E-stained lung slices (Fig. 4e), the sparse lung structure in the “DOX@Pd Gel + laser” group meant the successful defense against tumor metastasis, while the dense structures in the other groups indicated the sign of pulmonary metastasis of the tumor. The above results collectively demonstrated that the DOX@Pd Gel plus NIR laser irradiation could not only ablate the subcutaneous solid tumors effectively, but also inhibit cancer cell migration persistently.
The in vivo biocompatibility of the DOX@Pd Gel under NIR laser irradiation was further systematically investigated to explore the potential for clinical translation. To test the biosafety of “DOX@Pd Gel + laser” treatment to mice, the major organs separated from the healthy nude mice and the tumor-bearing nude mice after the “DOX@Pd Gel + laser” treatment were stained with H&E. The staining results in Fig. 5a demonstrated that the tissues maintained normal structures and no obvious organ damage or inflammatory lesions were observed, indicating the good biosafety of the “DOX@Pd Gel + laser” treatment. Furthermore, there were no significant differences regarding the levels of the numbers of red blood cells (RBC) and white blood cells (WBC), concentration of hemoglobin (HGB), mean corpuscular volume (MCV), mean corpuscular hemoglobin (MCH), mean corpuscular hemoglobin concentration (MCHC) and platelet (PLT) count between the “DOX@Pd Gel + laser”-treated mice and the healthy nude mice (Fig. 5b–h). Therefore, the above results collectively indicate the negligible systemic toxicity of the DOX@Pd Gel, which may be highly beneficial for its potential clinical uses.
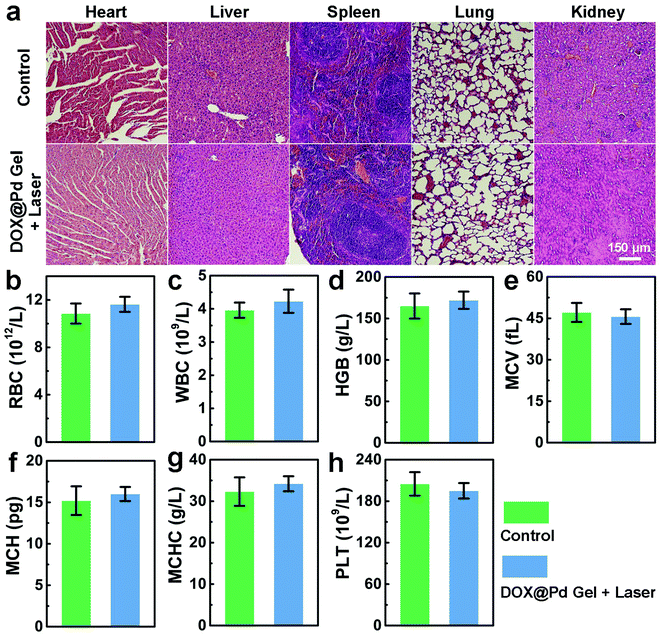 |
| Fig. 5
In vivo biosafety evaluation. (a) Photographs of H&E-stained slices of main organs separated from healthy mice (control) and “DOX@Pd Gel + laser”-treated mice. (b–h) Hemanalysis results of the numbers of red blood cells (RBC) and white blood cells (WBC), concentration of hemoglobin (HGB), mean corpuscular volume (MCV), mean corpuscular hemoglobin (MCH), mean corpuscular hemoglobin concentration (MCHC) and platelet (PLT) count in healthy mice (control) and “DOX@Pd Gel + laser”-treated mice. | |
Conclusion
In summary, we have constructed a multifunctional hydrogel with injectable, photothermal and chemotherapeutic properties for the inhibition of tumor growth and metastasis. Different from conventional NP-containing hydrogels in which the NPs are simply dispersed in the reservoir of the hydrogels, the present DOX@Pd Gel was prepared by the crosslinking between Pd NSs and multi-arm thiol-terminated polymers via Pd–S bonding accompanied by the loading of the anticancer drug DOX. It was found that the stability of the Pd NSs was remarkably improved after the formation of the hydrogel and the dynamic and reversible nature of the Pd–S bonds endowed the hydrogel with injectable property. In vitro cellular experiments confirmed the excellent anticancer performance of the chemo–photothermal combination therapy. Specifically, NIR laser could not only activate the photothermal effect of Pd NSs to partially kill the cancer cells via PTT, but also generate local hyperthermia to accelerate the DOX release for chemotherapy, realizing synergistic chemo-photothermal therapy. Furthermore, in vivo experiments further demonstrated that the DOX@Pd Gel could be facilely injected into the solid tumors of mice for efficiently eliminating the tumors and inhibiting tumor metastasis after NIR laser irradiation. More importantly, the histological analysis and hemanalysis results verified the good biosafety of the combined treatment with DOX@Pd Gel and laser. We anticipate that such a type of multifunctional and biocompatible hydrogel will show great promise in the development of new approaches for effective cancer therapies.
Ethical statement
All animal experimental procedures were conducted under the protocols approved by the Animal Care Committee of Southeast University and performed in compliance with the Regulations for the Administration of Affairs Concerning Experimental Animals of China.
Conflicts of interest
The authors declare no competing financial interest.
Acknowledgements
This work was supported by the National Natural Science Foundation of China (21673037 and 21574020), the Natural Science Foundation of Jiangsu Province (BK20170078), the Fundamental Research Funds for the Central Universities and the Scientific Research Foundation of the Graduate School of Southeast University (YBJJ1778).
Notes and references
- N. A. Peppas, J. Z. Hilt, A. Khademhosseini and R. Langer, Adv. Mater., 2006, 18, 1345–1360 CrossRef CAS.
- R. D. Liu, Y. S. Huang, Y. L. Ma, S. S. Jia, M. X. Gao, J. X. Li, H. M. Zhang, D. M. Xu, M. Wu, Y. Chen, Z. Zhu and C. Y. Yang, ACS Appl. Mater. Interfaces, 2015, 7, 6982–6990 CrossRef CAS PubMed.
- R. R. Xing, K. Liu, T. F. Jiao, N. Zhang, K. Ma, R. Y. Zhang, Q. L. Zou, G. H. Ma and X. H. Yan, Adv. Mater., 2016, 28, 3669–3676 CrossRef CAS PubMed.
- S. B. Seif-Naraghi, J. M. Singelyn, M. A. Salvatore, K. G. Osborn, J. J. Wang, U. Sampat, O. L. Kwan, G. M. Strachan, J. Wong, P. J. Schup-Magoffin, R. L. Braden, K. Bartels, J. A. DeQuach, M. Preul, A. M. Kinsey, A. N. DeMaria, N. Dib and K. L. Christman, Sci. Transl. Med., 2013, 5, 173ra25 CrossRef PubMed.
- X. D. Zhang, L. Y. Xia, X. K. Chen, Z. Chen and F. G. Wu, Sci. China Mater., 2017, 60, 487–503 CrossRef CAS.
- L. Y. Xia, X. D. Zhang, M. Cao, Z. Chen and F. G. Wu, Biomacromolecules, 2017, 18, 3073–3081 CrossRef CAS PubMed.
- P. Thoniyot, M. J. Tan, A. A. Karim, D. J. Young and X. J. Loh, Adv. Sci., 2015, 2, 1400010 CrossRef.
- F. P. Seib, E. M. Pritchard and D. L. Kaplan, Adv. Funct. Mater., 2013, 23, 58–65 CrossRef CAS PubMed.
- H. J. Yu, Z. R. Cui, P. C. Yu, C. Y. Guo, B. Feng, T. Y. Jiang, S. L. Wang, Q. Yin, D. F. Zhong, X. L. Yang, Z. W. Zhang and Y. P. Li, Adv. Funct. Mater., 2015, 25, 2489–2500 CrossRef CAS.
- Y. L. Cheng, C. L. He, J. X. Ding, C. S. Xiao, X. L. Zhuang and X. S. Chen, Biomaterials, 2013, 34, 10338–10347 CrossRef CAS PubMed.
- A. Fakhari and J. A. Subramony, J. Controlled Release, 2015, 220, 465–475 CrossRef CAS PubMed.
- P. Z. Elias, G. W. Liu, H. Wei, M. C. Jensen, P. J. Horner and S. H. Pun, J. Controlled Release, 2015, 208, 76–84 CrossRef CAS PubMed.
- H. C. Wu, S. S. Liu, L. Y. Xiao, X. D. Dong, Q. Lu and D. L. Kaplan, ACS Appl. Mater. Interfaces, 2016, 8, 17118–17126 CrossRef CAS PubMed.
- M. M. Pakulska, K. Vulic, R. Y. Tam and M. S. Shoichet, Adv. Mater., 2015, 27, 5002–5008 CrossRef CAS PubMed.
- M. M. Liang, K. L. Fan, M. Zhou, D. M. Duan, J. Y. Zheng, D. L. Yang, J. Feng and X. Y. Yan, Proc. Natl. Acad. Sci. U. S. A., 2014, 111, 14900–14905 CrossRef CAS.
- H. Ding, Y. J. Cai, L. Z. Gao, M. M. Liang, B. P. Miao, H. W. Wu, Y. Liu, N. Xie, A. F. Tang, K. L. Fan, X. Y. Yan and G. H. Nie, Nano Lett., 2019, 19, 203–209 CrossRef CAS PubMed.
- J. Y. He, K. L. Fan and X. Y. Yan, J. Controlled Release, 2019, 311–312, 288–300 CrossRef CAS.
- Y. L. Jin, J. Y. He, K. L. Fan and X. Y. Yan, Nanoscale, 2019, 11, 12449–12459 RSC.
- C. Yao, Z. Liu, C. Yang, W. Wang, X. J. Ju, R. Xie and L. Y. Chu, Adv. Funct. Mater., 2015, 25, 2980–2991 CrossRef CAS.
- S. J. Zhang, X. Q. Qian, L. L. Zhang, W. J. Peng and Y. Chen, Nanoscale, 2015, 7, 7632–7643 RSC.
- G. Gao, Y. W. Jiang, H. R. Jia and F. G. Wu, Biomaterials, 2019, 188, 83–95 CrossRef CAS.
- S. H. Tang, M. Chen and N. F. Zheng, Small, 2014, 10, 3139–3144 CrossRef CAS.
- X. Q. Huang, S. H. Tang, B. J. Liu, B. Ren and N. F. Zheng, Adv. Mater., 2011, 23, 3420–3425 CrossRef CAS PubMed.
- M. Chen, S. Z. Chen, C. Y. He, S. G. Mo, X. Y. Wang, G. Liu and N. F. Zheng, Nano Res., 2017, 10, 1234–1248 CrossRef CAS.
- X. Q. Huang, S. H. Tang, X. L. Mu, Y. Dai, G. X. Chen, Z. Y. Zhou, F. X. Ruan, Z. L. Yang and N. F. Zheng, Nat. Nanotechnol., 2011, 6, 28–32 CrossRef CAS PubMed.
- T. T. Sun, J. F. Han, S. Liu, X. Wang, Z. Y. Wang and Z. G. Xie, ACS Nano, 2019, 13, 7345–7354 CrossRef CAS PubMed.
- X. Wang, W. H. Lin, W. Zhang, C. Li, T. T. Sun, G. Chen and Z. G. Xie, J. Colloid Interface Sci., 2019, 536, 208–214 CrossRef CAS.
- X. X. Yao, P. Yang, Z. K. Jin, Q. Jiang, R. R. Guo, R. H. Xie, Q. J. He and W. L. Yang, Biomaterials, 2019, 197, 268–283 CrossRef CAS PubMed.
- W. H. Chen, C. X. Yang, W. X. Qiu, G. F. Luo, H. Z. Jia, Q. Lei, X. Y. Wang, G. Liu, R. X. Zhuo and X. Z. Zhang, Adv. Healthcare Mater., 2015, 4, 2247–2259 CrossRef CAS.
- L. Wen, L. Chen, S. M. Zheng, J. F. Zeng, G. X. Duan, Y. Wang, G. L. Wang, Z. F. Chai, Z. Li and M. Y. Gao, Adv. Mater., 2016, 28, 5072–5079 CrossRef CAS PubMed.
- J. Lee, K. J. Choi, S. U. Moon and S. Kim, Biomaterials, 2016, 74, 109–118 CrossRef CAS PubMed.
- Q. Pei, X. L. Hu, X. H. Zheng, R. Xia, S. Liu, Z. G. Xie and X. B. Jing, Nano Res., 2019, 12, 877–887 CrossRef CAS.
- J. Jin, M. Y. Guo, J. M. Liu, J. Liu, H. G. Zhou, J. Y. Li, L. M. Wang, H. B. Liu, Y. L. Li, Y. L. Zhao and C. Y. Chen, ACS Appl. Mater. Interfaces, 2018, 10, 8436–8442 CrossRef CAS.
- Y. T. Li, J. Jin, D. W. Wang, J. W. Lv, K. Hou, Y. L. Liu, C. Y. Chen and Z. Y. Tang, Nano Res., 2018, 11, 3294–3305 CrossRef CAS.
- X. J. Song, C. Liang, H. Gong, Q. Chen, C. Wang and Z. Liu, Small, 2015, 11, 3932–3941 CrossRef CAS PubMed.
- C. N. Li, W. H. Lin, S. Liu, W. Zhang and Z. G. Xie, J. Mater. Chem. B, 2019, 7, 4655–4660 RSC.
- F. Yin, K. Hu, Y. Z. Chen, M. Y. Yu, D. Y. Wang, Q. Q. Wang, K. T. Yong, F. Lu, Y. Y. Liang and Z. G. Li, Theranostics, 2017, 7, 1133–1148 CrossRef CAS PubMed.
- W. Cheng, J. P. Nie, N. S. Gao, G. Liu, W. Tao, X. J. Xiao, L. J. Jiang, Z. G. Liu, X. W. Zeng and L. Mei, Adv. Funct. Mater., 2017, 27, 1704135 CrossRef.
- Q. Chen, L. G. Xu, C. Liang, C. Wang, R. Peng and Z. Liu, Nat. Commun., 2016, 7, 13193 CrossRef CAS.
- J. R. Peng, Y. Xiao, W. T. Li, Q. Yang, L. W. Tan, Y. P. Jia, Y. Qu and Z. Y. Qian, Adv. Sci., 2018, 5, 1700891 CrossRef PubMed.
- W. F. Chen, M. Qin, X. Y. Chen, Q. Wang, Z. R. Zhang and X. Sun, Theranostics, 2018, 8, 2229–2241 CrossRef CAS PubMed.
- R. P. Wang, Z. S. He, P. J. Cai, Y. Zhao, L. Gao, W. Z. Yang, Y. L. Zhao, X. Y. Gao and F. P. Gao, ACS Appl. Mater. Interfaces, 2019, 11, 13964–13972 CrossRef CAS PubMed.
- Z. H. Li, H. B. Wang, Y. J. Chen, Y. Wang, H. Li, H. J. Han, T. T. Chen, Q. Jin and J. Ji, Small, 2016, 12, 2731–2740 CrossRef CAS PubMed.
- H. Li, G. X. Chen, H. Y. Yang, X. L. Wang, J. H. Liang, P. X. Liu, M. Chen and N. F. Zheng, Angew. Chem., Int. Ed., 2013, 52, 8368–8372 CrossRef CAS PubMed.
- Y. C. Chung, Y. H. Chiu, Y. W. Wu and Y. T. Tao, Biomaterials, 2005, 26, 2313–2324 CrossRef CAS PubMed.
- A. G. Young, D. P. Green and A. J. McQuillan, Langmuir, 2007, 23, 12923–12931 CrossRef CAS.
- J. E. Park, S. G. Park, A. Koukitu, O. Hatozaki and N. Oyama, Synth. Met., 2004, 140, 121–126 CrossRef CAS.
- H. Tada, T. Soejima, S. Ito and H. Kobayashi, J. Am. Chem. Soc., 2004, 126, 15952–15953 CrossRef CAS PubMed.
- Y. W. Yang and L. J. Fan, Langmuir, 2002, 18, 1157–1164 CrossRef CAS.
- A. Gniewek, A. M. Trzeciak, J. J. Ziółkowski, L. Kępiński, J. Wrzyszcz and W. Tylus, J. Catal., 2005, 229, 332–343 CrossRef CAS.
- K. R. Priolkar, P. Bera, P. R. Sarode, M. S. Hegde, S. Emura, R. Kumashiro and N. P. Lalla, Chem. Mater., 2002, 14, 2120–2128 CrossRef CAS.
- G. Liu, M. Q. Hou, T. B. Wu, T. Jiang, H. L. Fan, G. Y. Yang and B. X. Han, Phys. Chem. Chem. Phys., 2011, 13, 2062–2068 RSC.
- J. J. Li, D. Hartono, C. N. Ong, B. H. Bay and L. Y. L. Yung, Biomaterials, 2010, 31, 5996–6003 CrossRef CAS PubMed.
- H. W. Yang, K. J. Hwang, H. C. Kwon, H. S. Kim, K. W. Choi and K. S. Oh, Hum. Reprod., 1998, 13, 998–1002 CrossRef CAS PubMed.
Footnotes |
† Electronic supplementary information (ESI) available. See DOI: 10.1039/c9nr08454a |
‡ These authors contributed equally to this work. |
|
This journal is © The Royal Society of Chemistry 2020 |