DOI:
10.1039/C9NJ04763H
(Paper)
New J. Chem., 2020,
44, 162-170
Peroxynitrite (ONOO−) generation from the HA-TPP@NORM nanoparticles based on synergistic interactions between nitric oxide and photodynamic therapies for elevating anticancer efficiency†
Received
18th September 2019
, Accepted 19th November 2019
First published on 19th November 2019
Abstract
Due to biological safety and negligible toxicity, nitric oxide (NO) therapy has gained increasing interest in the field of cancer therapy during the past few years. However, individual NO cancer therapy normally exhibited limited therapeutic efficiency. In order to acquire satisfactory therapeutic outcomes, the NO therapy is usually combined with other therapeutic treatments, mostly chemotherapy. Herein, we constructed HA-TPP@NORM nanoparticles based on the co-assembly of an NO donor (NORM) and tetraphenylporphyrin (TPP)-modified hyaluronic acid, which can efficiently generate a highly biocidal molecule of peroxynitrite (ONOO−) via the synergistic interactions of the nitric oxide (NO) therapy and photodynamic therapy (PDT) to enhance the cancer therapeutic efficiency. In addition, MTT results exhibited that without light irradiation, the HA-TPP@NORM nanoparticles have favourable biocompatibility with the cell viability above 95% at the maximum TPP concentration (20 μg mL−1). Under simultaneous irradiation with 365 nm and 650 nm light, ONOO− can be efficiently produced in cancer cells via the direct coupling reaction of the generated NO and superoxide anion radical (O2˙−), which significantly enhanced the anticancer effect, when compared with individual NO therapy or PDT therapy. Therefore, the HA-TPP@NORM nanoparticles may provide a new insight into the design of efficiently NO-related cancer therapeutic systems.
Introduction
As a “star” molecule, nitric oxide (NO) is involved in various physiological and pathological processes, such as immune responses, apoptosis, angiogenesis, cardiovascular homeostasis and neurotransmission.1–5 Due to its biological safety and negligible toxicity, NO has gained increasing interest during the past few years for applications in cancer therapy.6–12 However, individual NO therapy normally exhibited a limited cancer therapeutic efficiency. High concentrations of NO release (>1 μM) in cancer cells are the prerequisite to induce limited cancer cell death,13 which need to efficiently supply a large amount of the NO donor in the tumour site. At low concentrations, nitric oxide (NO) can not only accelerate tumour angiogenesis,14 but it can also overcome the multidrug resistance of chemotherapy by suppressing the expression of P-glycoprotein.15 Thus, NO is usually combined with chemotherapeutic drugs (DOX, etc.) to elevate the anticancer efficiency.16–18 However, inevitable nonspecific systemic toxicity could be caused by the early release of chemotherapy drugs, which severely limits its clinical applications.
Peroxynitrite (ONOO−), as a derivative of NO, is also an endogenous signalling molecule and plays a key role in various physiological and pathological processes.19–22 As a highly reactive nitrogen species, ONOO− shows significant oxidization or nitration capabilities, which can considerably cause cell death by destroying proteins, lipids and DNA. In addition, its cytotoxicity is higher than most free radicals, such as NO and hydroxyl radical (˙OH).23,24 Thus, we assume that the introduction of ONOO− can enhance the cancer therapeutic efficiency.
In living systems, ONOO− can be produced by a direct coupling reaction between NO and superoxide anion radical (O2˙−).25 One feasible way to produce O2˙− can be realised by the type I photodynamic therapy (PDT).26 Due to the minimal invasiveness, spatiotemporal controllability and excellent anticancer effect, PDT has attracted a great deal of interest in cancer therapy.27–36 Porphyrins and their derivatives, as typical photosensitizers (PSs), have been widely used during PDT processes due to their high molar absorption coefficients, high singlet oxygen quantum yields and excellent biocompatibility.37–42 Based on the different photochemical reaction processes, PDT can be divided into two types: type I PDT and type II PDT. In particular, PSs (in the excited triplet state) convert triplet oxygen (3O2) into singlet oxygen (1O2) via direct energy transfer (type II). Simultaneously, the excited PS can also directly react with 3O2 to produce O2˙− (type I).26
Herein, the intelligent photo-responsive HA-TPP@NORM nanoparticles based on the co-assembly of tetraphenylporphyrin (TPP) modified hyaluronic acid (HA) and NO donor (NORM) have been successfully constructed for generating ONOO−via synergistic interactions between PDT and NO therapy in order to elevate the anticancer efficiency (Scheme 1). With a 650 nm light irradiation, the excited TPP can react with surrounding 3O2 to produce 1O2 and O2˙−. Simultaneously, the released NO from NORM irradiated with 365 nm light can immediately react with the produced O2˙− to generate the highly biocidal molecule ONOO−. It is noteworthy to mention that a lot of NO donors,43 such as bis-N-nitroso compounds (BNNs),44,45 Roussin's black salt (RBS),46,47S-nitrosothiols,48,49 and N-diazeniumdiolates,50,51 have been widely used for curing cancer. In this study, N-nitrosated napthalimide (NORM)52 was used as an NO donor. Under UV light irradiation, NORM can rapidly release NO. Moreover, the quenched fluorescence can be recovered, which can be used to monitor the released NO. First, we have checked the as-prepared HA-TPP@NORM nanoparticles, and subsequently evaluated for the capability of generating NO, 1O2 and ONOO−. Finally, the MTT assay was utilized to evaluate the dark- and photo-cytotoxicity of HA-TPP@NORM nanoparticles.
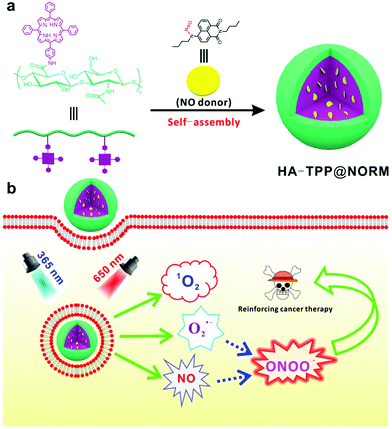 |
| Scheme 1 Schematic of peroxynitrite (ONOO−) generation from HA-TPP@NORM nanoparticles. (a) The structure and preparation of HA-TPP@NORM nanoparticles. (b) Illustration of the mechanism for intracellular generating ONOO− from HA-TPP@NORM nanoparticles. | |
Materials and methods
Materials
Benzaldehyde, propionic acid, pyrrole, trichloroacetic acid (CCl3COOH), trifluoroacetic acid (CF3COOH), acetic acid (CH3COOH), sodium nitrite (NaNO2), hydrochloric acid, tin(II) dichloride dehydrate, ammonium hydroxide, 4′,6-diamidino-2-phenylindole (DAPI), 3-(4,5-dimethylthiazol-2-yl)-2,5-diphenyl- tetrazolium bromide (MTT), 1-(3-dimethylaminopropyl)-3-ethylcarbodiimide hydrochloride (EDC), N-hydroxysuccinimide (NHS), 1,3-diphenylisobenzofuran (DPBF), 4-bromo-1,8-naphthalic anhydride, n-butylamine, ethanol and sodium bicarbonate were purchased from Aladdin and used as received. A nitric oxide assay kit (Griess assay) was obtained from Beyotime Institute of Biotechnology. Sodium hyaluronate (7500 Dalton) was purchased from BLOOMAGE FREDA BIOPHARM CO., LTD. All other reagents were of analytical grade and used directly.
Characterisation
An AV 400 Spectrophotometer (400 MHz) was used to record the 1H NMR spectra. Tetramethylsilane (TMS) was used as an internal reference, and CDCl3 or DMSO-d6 were used as the solvent. A UV-2450 UV-visible spectrophotometer and F-4500 fluorescence spectrophotometer were used to obtain the UV-vis spectra and fluorescence spectra, respectively. Dynamic light scattering (DLS) measurements were performed using a BECKMAN COULTER Delasa Nano C particle analyzer at room temperature. A transmission electron microscopy (TEM) image was recorded on a JEOL JEM1400 electron microscope at an accelerating voltage of 100 kV. Confocal laser scanning microscopy (CLSM) was performed on a Nikon A1R, and flow cytometry was performed using a BD FACS Calibur flow cytometer. The MTT assay was conducted using a spectrophotometric microplate reader (Thermo Multiskan MK3 spectrometer) at a wavelength of 560 nm.
Synthesis of TPP
TPP was synthesized according to the previous literature.53 Benzaldehyde (8.0 g, 75 mmol) and propionic acid (220 mL) were added into a 500 mL three-necked round-bottom flask fitted with a constant pressure drop funnel and a reflux condenser. Under nitrogen protection, the mixture was heated to 140 °C, then pyrrole (5.2 mL) was added dropwise into the mixture. After reacting for 4 h, the reaction mixture was slowly cooled to room temperature. Propionic acid was removed with reduced pressure distillation. 400 mL methanol was subsequently added into the mixture, and it was placed in −20 °C for 24 h. The resulting precipitate was obtained by filtration and washed with cold methanol several times. Finally, the crude product was further purified with column chromatography to obtain 2.1 g TPP with 18.4% yield.
Synthesis of TPP-NH2
TPP (2.0 g, 3.25 mmol), trichloroacetic acid (26.6 g, 163 mmol), acetic acid (9.3 mL, 163 mmol) and trifluoroacetic acid (7.2 mL, 98 mmol) were added into a 250 mL round-bottom flask. After the mixture was stirred at 0 °C for 30 min, NaNO2 (561.2 mg, 8.13 mmol) was added into the reaction mixture and stirred for another 24 h. 100 mL of H2O was added into the reaction mixture to quench the reaction, and then CH2Cl2 (3 × 100 mL) was used to extract the crude product. The organic layer was dried with Na2SO4, filtered and rotary-evaporated to obtain the crude product of nitration (TPP-NO2).
The crude product was added to 200 mL concentrated hydrochloric acid, and then tin(II) dichloride dehydrate (2.7 g, 12.13 mmol) was added into the mixture. After the mixture was heated to 80 °C for 2 h, 100 mL of H2O was added into the mixture to terminate the reaction and kept at room temperature for another 12 h. The green precipitate was obtained by filtration and subsequently dissolved in 150 mL of CH2Cl2. Afterwards, 100 mL of H2O was added into the solution, and then ammonium hydroxide was used to neutralize the mixture until the color changed to violet. The organic layer was collected and dried with Na2SO4, subsequently filtered and rotary-evaporated to obtain the crude product, which was further purified with a silica gel column using CH2Cl2 and PE as the eluents to afford 0.9 g TPP-NH2 in 43.6% yield.
Synthesis of the TPP-modified hyaluronic acid (HA-TPP)
Sodium hyaluronate was transformed into hyaluronic acid (HA), according to the procedure mentioned in the previous work.54 Sodium hyaluronate (3.75 g, 0.5 mmol) was dialyzed against 0.01 N HCl, followed by dialysis against deionized water several times. After the solution was lyophilized, the acidic form of HA (3.5 g, 0.47 mmol) was obtained. HA (1 g, 0.13 mmol) and TPP-NH2 (500 mg, 0.79 mmol) were dissolved in the co-solvent of water and DMF, then NHS (115 mg, 1 mmol) was added into the reaction mixture. HCl was used to adjust the pH of the solution to 4.5. After stirring for 30 min, EDC (191.7 mg, 1 mmol) was added into the reaction mixture. The reaction was set to proceed for 48 h, and then the reaction mixture was dialyzed using a dialysis tube (MWCO = 3500) using the co-solvent of DMF and deionized water, followed by dialysis against deionized water to remove DMF. Finally, the solution was lyophilized to get HA-TPP (1.2 g).
Synthesis of M2
M2 was synthesized according to the previous literature.52M1 (20 g, 72.18 mmol) and n-butylamine (6.34 g, 86.616 mmol) were added into 500 mL of ethanol. Under reflux conditions, the mixture was stirred for 8 h, and then filtered and washed by ethanol to afford a crude product. The crude product was purified by recrystallization in the co-solvent of ethanol and water to obtain M2 (20.6 g) in 86% yield.
Synthesis of M3
M3 was synthesized also according to same previous literature.52 M2 (10 g, 30.29 mmol), n-butylamine (2.66 g, 36.35 mmol) and NaHCO3 (3.05 g, 36.35 mmol) were added into 300 mL of DMSO. The mixture was stirred and heated to 110 °C for 12 h and then cooled to room temperature. Then, DMSO was evaporated under a reduced pressure. The resulting residue was transferred into 300 mL of H2O, and then extracted by CH2Cl2 three times. The organic layer was dried with Na2SO4, filtered and rotary-evaporated to obtain the crude product, which was further purified with a silica gel column with CH2Cl2 and PE as the eluent to afford the M3 (7.96 g) in 81% yield.
Synthesis of NORM
M3 (1 g, 3.08 mmol) was dissolved in a co-solvent of AcOH, HCl, CH2Cl2 (10
:
0.5
:
7, v/v) (40 mL) and cooled to 0 °C. NaNO2 (478.17 mg, 4.62 mmol) was added into the mixture, stirred for 30 min. Then, the ice bath was removed and the reaction mixture was stirred at room temperature for another 2 h. A saturated NaHCO3 solution was added to the flask until the solution pH was adjusted to neutral. The crude product was extracted with CH2Cl2 three times. Then, the organic layer was dried with Na2SO4, filtered and rotary-evaporated to obtain the crude product. The crude product was further purified using a silica gel column using the co-solvent of CH2Cl2 and CH3OH as eluent to afford the NORM (674 mg, 62% yield).
Preparation of the HA-TPP@NORM nanoparticles
10 mg HA-TPP and 5 mg NORM were dissolved in 1 mL of DMSO, and then the solution was dropwise added into 4 mL of H2O at room temperature. The obtained solution was dialyzed against deionized water using a dialysis membrane (MWCO = 3500) for 24 h in order to remove DMSO. In order to measure the NORM content in the nanoparticles, the solution was lyophilized and then dissolved in DMSO and irradiated with UV light for 20 min to make the encapsulated NORM totally decomposed to M3. The content of NORM was then determined by UV-vis absorption spectrum at 445 nm. The NORM loading and entrapment efficiency of the HA-TPP@NORM nanoparticles were separately calculated using the following equations:
NORM loading (%) = NORM amount in the NPs/mass of NPs × 100% |
Entrapment efficiency (%) = NORM amount in NPs/amount of NORM used × 100% |
Detection of 1O2 with DPBF
1,3-Diphenylisobenzofuran (DPBF) was used to detect the generated singlet oxygen (1O2) in an aqueous solution with 1% DMSO to improve the solubility of DPBF. The HA-TPP@NORM nanoparticles were irradiated with a 650 nm laser with 1 W cm−2, then the decay of DPBF at 420 nm was monitored every 5 s using a UV-vis spectrophotometer.
Quantitative detection of the NO release from the HA-TPP@NORM nanoparticles by the Griess assay
A typical Griess assay was used to quantitatively detect the NO release from the HA-TPP@NORM nanoparticles. The detection principle of the method is that the released NO molecules, after contact with water, could be converted into nitrate or nitrite then react with a Griess agent and finally converted into an azo dye, which could be quantitatively determined using a microplate reader or via UV-vis absorption spectroscopy (λ = 540 nm). Before testing, a standard curve was established by the NaNO2 standard samples (0–100 μM) for the calculation of NO concentrations (Fig. S12, ESI†). Detailed detection steps are described below. 250 μL of the sample solution was added into 250 μL of Griess reagent I and shaken for 1 min. Then, 250 μL of Griess reagent II was added into the mixture solution and shaken for another 2 min in dark. At last, 1.25 mL of water was added into the mixture before the mixture was measured using a UV-visible spectrophotometer.
Determination of ONOO−
L-Tyrosine was used as a probe molecule in the presence of CO2 in a weak alkaline environment. Dityr was generated by the oxidation of ONOO−. In a typical experiment, 10 mL of an aqueous solution containing PBS (0.10 M, pH = 8.2), NaHCO3 (0.015 M), and L-tyrosine (5.0 × 10−4 M) was prepared at room temperature. Then, 1 mg of HA-TPP@NORM nanoparticles was added into the solution and illuminated with 365 nm (130 mW cm−2) and 650 nm (1 W cm−2) lights for 1 min. Subsequently, a fluorescence spectrophotometer was used to detect the ONOO− at an excitation wavelength at 313 nm.
Cell culture
HeLa cells kindly provided by the Institute of Biochemistry and Cell Biology, SIBS, Chinese Academy of Sciences were cultured in Dulbecco's modified Eagle medium (DMEM), containing 10% fetal bovine serum (FBS) and antibiotics (50 units per mL streptomycin and 50 units per mL penicillin) under a humidified atmosphere with 5% CO2 at 37 °C.
Intracellular ROS detection
Intracellular ROS production was measured via 2′,7′-dichlorodihydrofluorescein diacetate (DCFH-DA) via confocal laser scanning microscopy (CLSM). After taken up by the cancer cells, DCFH-DA will be deacetylated by the intracellular esterases to form dichlorodihydrofluorescein (DCFH). DCFH can transform to fluorescent dichlorofluorescein (DCF) in the presence of ROS. HeLa cells were plated onto glass-bottom Petri dishes with 1 × 105 cells per well. After 24 h of incubation, the cells were treated with the HA-TPP@NORM nanoparticles. After washing with PBS to remove the residual nanomaterials, the cells were incubated with DCFH-DA (10 μM) for 30 min. Then, the cells were thoroughly washed with PBS then irradiated with 650 nm laser (1 W cm−2, 10 min) or not. Finally, the fluorescence of DCF was observed by CLSM for each group.
Detection of the intracellular NO release from HA-TPP@NORM nanoparticles by self-calibration with CLSM and flow cytometry
The behaviour of the NO release in HeLa cells was detected by self-calibration with CLSM. HeLa cells were seeded onto glass-bottom Petri dishes with 1 × 105 cells per well and then cultured for 24 h. After the addition of HA-TPP@NORM nanoparticles and incubation for another 24 h, the cells were washed with PBS 3 times to remove residual nanoparticles, and then stained with DAPI and fixed with 4% paraformaldehyde solution for 30 min. Then, the cells were irradiated with UV light for 0 s, 15 s or 45 s. Finally, confocal luminescent imaging experiments were performed on a confocal laser scanning microscope (Nikon AIR). The excitation wavelength was 488 nm, and the emission wavelength was 525 nm.
The behaviour of the NO release in HeLa cells was also detected via flow cytometry. HeLa cells were seeded into a 6-well plate at 2 × 105 per well and then cultured at 37 °C for 24 h. After the addition of HA-TPP@NORM nanoparticles and incubation for another 24 h, the cells were washed with PBS 3 times to remove residual nanoparticles. Then, the cells were irradiated with UV light for 0 s, 15 s or 45 s, respectively. Subsequently, the cells were harvested and washed with PBS three times and re-suspended in PBS. Finally, the fluorescence intensity was detected using a BD FACS Calibur flow cytometer.
Intracellular detection of the generated ONOO−
To detect intracellular ONOO−, a Cell Meter Assay Kit (AAT Bioquest) for peroxynitrite assay was used. The cells with concentration of 5 × 103 cells per well were treated with a medium containing 100 μL of HA-TPP or HA-TPP@NORM nanoparticles for 24 h. Then, DAX-J2 PON Green working solution (10%) was added into the cells and incubated for another 30 min. After washing with PBS, the cells were irradiated with 365 nm, 650 nm or 365 nm and 650 nm light for 10 min and incubated for another 30 min. The absorbance of each well was monitored at Ex/Em = 490/530 nm.
In vitro cytotoxicity assay
Dark toxicity.
HeLa cells were seeded into a 24-well plate at 5000 per well and then cultured at 37 °C for 24 h. HA-TPP or HA-TPP@NORM nanoparticles with different concentrations dispersed in a culture medium were added into the wells and incubated for another 24 h. Subsequently, the cells were washed using sterilized PBS three times, and the culture medium was replaced with 200 μL of fresh DMEM included 20 μL of MTT (5 mg mL−1). The cells were incubated for another 4 h. After that, 150 μL of DMSO was added into the wells with gentle shaking to extract the formazan products. Then, the absorbance was measured at 560 nm using a microplate reader. Cell viability (%) was calculated according to the equation: cell viability (%) = (ODtest − ODbackground)/(ODcontrol − ODbackground) × 100, where ODtest is the absorbance in the presence of sample solutions, and ODcontrol is the absorbance of the control group.
Photo-toxicity.
HeLa cells were seeded into a 24-well plate at 5000 per well and then cultured at 37 °C for 24 h. HA-TPP or HA-TPP@NORM nanoparticles with different concentrations dispersed in a culture medium were added into the wells and incubated for another 24 h. Subsequently, the cells were washed with sterilized PBS 3 times to remove residual nanoparticles, and fresh DMEM was added into the plate. Then, the cells were irradiated using a 365 nm light (130 mW cm−2) or 650 nm light (1 W cm−2) or 365 nm plus 650 nm light for 10 min, and put back into an incubator for another 24 h. Finally, cell viability was evaluated using the MTT assay.
Statistical analysis
All experiments were performed at least three times under the same experimental conditions. The results are expressed as means ± standard errors. A statistical analysis was performed by one way (ANOVA), followed by the Bonferroni test using the SPSS 19.0 software, the p-value < 0.05 was considered to be statistically significant in all cases.
Results and discussion
HA-TPP was synthesized via amidation reaction between TPP-NH2 and hyaluronic acid (HA), catalysed with EDC and NHS. A detailed synthetic route has been shown in Scheme S1 (ESI†). According to the 1H NMR spectrum (Fig. 1a), the TPP was successfully grafted onto HA due to the appearance of hydrogen proton signals (δ = 9–7 ppm and −3 ppm), which all belong to TPP-NH2. The successful conjugation of TPP was further confirmed via Fourier transform infrared spectroscopy (FTIR) (Fig. S3, ESI†). NORM, as a self-calibrating NO donor was selected, and the detailed synthetic route has been shown in supporting information (Scheme S2, ESI†). Under UV light irradiation, the characteristic absorption peak at 345 nm has disappeared rapidly (Fig. S7, ESI†), while the characteristic absorption peak at 450 nm has increased. Accompanying the NO releasing processes, the fluorescence at 555 nm was recovered steadily (Fig. S8, ESI†). This behaviour has also been found in previous literatures,52–55 which can be used to conveniently detect intracellular NO release.
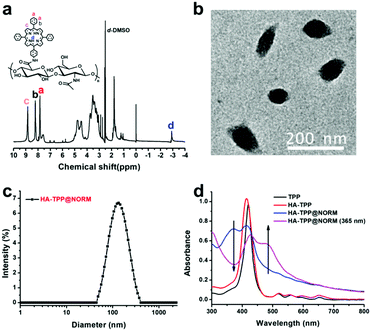 |
| Fig. 1 Preparation and characterization of the HA-TPP@NORM nanoparticles. (a) 1H NMR spectrum of HA-TPP. (b) TEM image of HA-TPP@NORM nanoparticles. (c) DLS of HA-TPP@NORM nanoparticles. (d) UV-vis absorption spectra of HA-TPP@NORM nanoparticles in aqueous solution. | |
Finally, the HA-TPP@NORM nanoparticles were prepared by the co-assembly of HA-TPP and NORM in an aqueous solution via a nanoprecipitation method. TEM and dynamic light scattering (DLS) were utilized to characterize the obtained HA-TPP@NORM nanoparticles. According to the TEM result (Fig. 1b), the diameter of the nanoparticles is about 95.3 nm. As shown in Fig. 1c, the diameter detected by DLS is about 108.6 nm, and polydispersity index (PDI) is 0.187. The size calculated by DLS is bigger than that determined by TEM, which may be due to the extending state of a hydrophilic segment in an aqueous solution. In addition, the HA-TPP@NORM nanoparticles have favourable stability because the diameter of the nanoparticles remained almost unchanged (when stored at room temperature in the dark for two weeks), which is beneficial for biomedical applications (Fig. S9, ESI†).
The UV-vis absorption spectra (Fig. 1d) indicated that NORM was successfully loaded into HA-TPP, according to the simultaneous appearance of the characteristic absorption peaks of TPP (about 420 nm) and NORM (about 360 nm). After the HA-TPP@NORM nanoparticles were irradiated with UV light, the absorption peak at 360 nm decreased but the absorption peak at 485 nm increased. This phenomenon is similar to that of NORM under an UV light irradiation (Fig. S7, ESI†). The encapsulation and loading efficiencies of NORM were determined to be 50.2% and 26.7%, respectively, which were quantified by the standard curve of the UV-vis absorption spectrometry of M3, which is the degradation product of NORM (Fig. S10, ESI†).
1,3-Diphenylisobenzofuran (DPBF) was widely used to determine the produced 1O2. The structure of DPBF can be destroyed by 1O2, resulting in a decrease in the absorption peak at 420 nm. As shown in Fig. 2a, the absorption of DPBF sharply reduced after the HA-TPP@NORM nanoparticles were irradiated with a 650 nm light (1 W cm−2) to excite TPP for only several seconds. This result indicated that TPP in the nanoparticles can efficiently produce 1O2 during the PDT process.
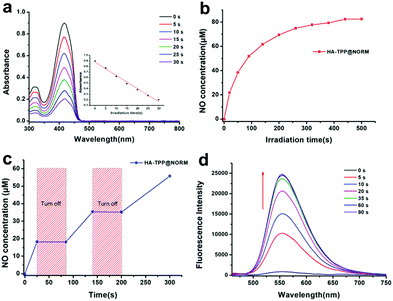 |
| Fig. 2 The abilities of the HA-TPP@NORM nanoparticles for generating of 1O2 and NO. (a) Singlet oxygen (1O2) generation as determined by the decay of DPBF. (b) NO release from HA-TPP@NORM nanoparticles under irradiation with 365 nm light. (c) On demand NO release from HA-TPP@NORM under UV light irradiation. (d) The fluorescence spectra of HA-TPP@NORM nanoparticles excited with 445 nm under irradiation with 365 nm light. | |
The behaviour of the NO release was quantitatively detected using a typical Griess assay. As shown in Fig. 2b, a large amount of NO was released (>40 μM) from the HA-TPP-NORM nanoparticles under the excitation of UV light within 100 seconds, which is beneficial for cancer therapy due to only high concentrations of NO with tumoricidal effect. Moreover, the ability of the controllable NO release from the HA-TPP@NORM nanoparticles was also studied. Under UV light irradiation, a NO burst was released, but the enhancement in the NO level immediately stopped after turning off the UV light (Fig. 2c). It can be concluded that NO can be quickly and controllably released from the HA-TPP@NORM nanoparticles. With the NO release, the fluorescence signal at 558 nm can also be recovered (Fig. 2d), exhibiting a similar change in small NORM molecules (Fig. S8, ESI†). This ability of self-calibration for the NO release is convenient and advantageous for investigating the intracellular NO release; thus, external NO detection reagents are not needed during the intracellular detection process.
Peroxynitrite (ONOO−) can be detected by L-tyrosine (L-try) in the presence of CO2 in a weak alkaline environment.56 After being oxidized by ONOO−, the dimerization of L-tyrosine (Dityr) will be generated. The fluorescence spectrophotometer was used to detect Dityr. The excitation wavelength (λex) is located at 313 nm, and the corresponding emission spectrum is around 400 nm. As revealed in Fig. 3, without light irradiation, no fluorescence signal was detected in the following groups, such as L-try, L-try + HA-TPP, HA-TPP@NORM and L-try + HA-TPP@NORM, which mean that none ONOO− was generated in these groups. After HA-TPP was added into L-try and then irradiated with 365 nm and 650 nm lights, still no fluorescence signal appeared in this system, meaning that still no ONOO− was produced. Without synergistic interaction, the groups (HA-TPP@NORM (365 nm), HA-TPP@NORM (650 nm), L-try + HA-TPP@NORM (365 nm) and L-try + HA-TPP@NORM (650 nm)) also could not produce ONOO−. It was thrilling to find that the fluorescence intensity at 400 nm of Dityr was very strong after HA-TPP@NORM nanoparticles and L-try were mixed together and then irradiated with 365 and 650 nm light. According to these results, it can be concluded that the generated O2˙− from type I PDT can efficiently react with the released NO to produce ONOO−.
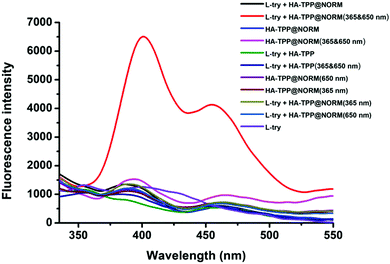 |
| Fig. 3 Detection of the produced ONOO− with L-tyrosine by a fluorescence spectrophotometer (λex = 313 nm). | |
The cellular uptake of the HA-TPP@NORM nanoparticles is shown in Fig. S11 (ESI†). Without HA-TPP@NORM nanoparticles, no red fluorescence of TPP has appeared in the HeLa cells. However, incubated with the HA-TPP@NORM nanoparticles for 8 h, red fluorescence appeared. By extending incubation time to 24 h, the red fluorescence was significantly enhanced. These results indicated that the HeLa cells can uptake the HA-TPP@NORM nanoparticles in a time-dependent manner.
Intracellular 1O2 production was evaluated using 2′,7′-dichloro-dihydrofluorescein diacetate (DCFH-DA), which exhibited green fluorescence after having reacted with 1O2. As shown in Fig. S12 (ESI†), the HeLa cells incubated with the HA-TPP@NORM nanoparticles and irradiated with 650 nm light exhibited strong green fluorescence, but negligible green fluorescence was found in the HeLa cells without 650 nm laser irradiation, indicating that a large amount of 1O2 was generated and an efficient PDT process was conducted in cancer cells.
The intracellular NO release was investigated via CLSM and flow cytometry. After HA-TPP@NORM nanoparticles were incubated with HeLa cells for 24 h, these cells were irradiated with 365 nm light for different durations. As depicted in Fig. 4a, green fluorescence was not present in the group without irradiation. After the group was irradiated for 15 s, green fluorescence immediately appeared. However, by extending illumination time to 45 s, enhanced green fluorescence was found. According to these results, it can be concluded that the intracellular controllable NO release could be realized by manipulating irradiation durations. The data from the flow cytometry (Fig. S13, ESI†) also exhibited a similar outcome for the group irradiated for 45 s exhibited the strongest fluorescence.
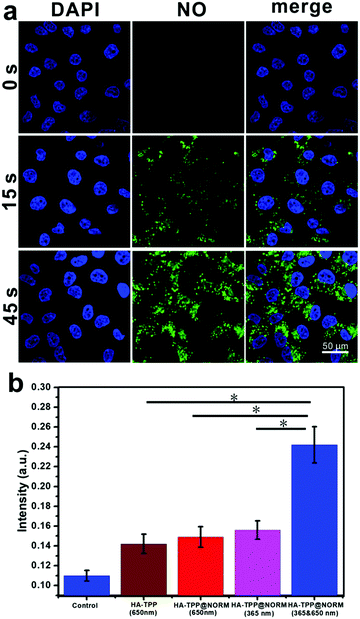 |
| Fig. 4 (a) Intracellular NO release from the HA-TPP@NORM nanoparticles detected with CLSM. The blue signal is assigned to the nucleus of HeLa cells stained with DAPI; the green signal is assigned to NO. Scale bar: 50 μm. (b) Intracellular ONOO− quantitative detection with DAX-J2 PON Green reagent. | |
As a highly selective reagent, DAX-J2 PON Green57 was utilized to determine intracellular ONOO−. As shown in Fig. 4b, the HeLa cells treated with HA-TPP or HA-TPP@NORM nanoparticles and irradiated with 650 nm laser (1 W cm−2) slightly enhanced the level of ONOO−, which may have resulted from the reaction between O2˙− from type I PDT and endogenous intracellular NO. The cells treated with HA-TPP@NORM but irradiated only with the 365 nm laser also slightly increased the ONOO− level, which may be ascribed to the generated NO from the nanoparticles reacted with endogenous intracellular O2˙−. It should be noted that, compared with treatments of HA-TPP (650 nm), HA-TPP@NORM (650 nm) and HA-TPP@NORM (365 nm), the intracellular ONOO− level has significantly increased in the HeLa cells treated with HA-TPP@NORM nanoparticles and irradiated with the 365 nm and 650 nm lights, which further verified that the synergistic interactions of PDT and NO can generate ONOO− in the cancer cells.
Encouraged by the controllable NO release and an efficient generation of 1O2 and ONOO−, the cancer therapeutic effects of the HA-TPP@NORM nanoparticles were subsequently evaluated by the virtue of the 3-(4,5-dimethylthiazol-2-yl)-2,5-diphenyl-tetrazolium bromide (MTT) assay. As shown in Fig. 5a, no obvious dark toxicity against cancer cells was observed in HA-TPP and HA-TPP@NORM groups because the cell viability was still high above 95% with the highest TPP concentration (20 μg mL−1). It can be concluded that HA-TPP and HA-TPP@NORM have favourable biocompatibility. The photo-toxicity of the HA-TPP@NORM nanoparticles was evaluated. As depicted in Fig. 5b, the cells treated with HA-TPP@NORM nanoparticles and irradiated only with the 365 nm light exhibited slightly cytotoxicity, probably due to a small number of NO released from the nanoparticles, indicating that NO therapy alone has a limited anticancer effect. In addition, the PDT treatment of the HA-TPP (650 nm) group also showed weak cytotoxicity with cell viability exceeded 65%, which may be ascribed to the low therapeutic TPP concentration (2 μg mL−1), resulting in limited ROS production in the HeLa cells. It was surprising to find that the HeLa cells incubated with HA-TPP@NORM nanoparticles and irradiated with 365 and 650 nm light exhibited the lowest cell viability compared with the other two groups, suggesting that the synergistic interaction of PDT and NO therapy can obviously elevate the anticancer efficiency by generating the highly biocidal ONOO− molecules.
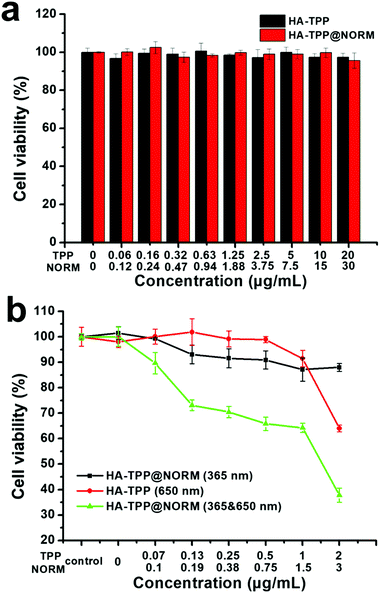 |
| Fig. 5 (a) Dark- and (b) photo-toxicity of HA-TPP@NORM nanoparticles against HeLa cells. | |
Conclusions
In conclusion, we have successfully constructed multifunctional HA-TPP@NORM nanoparticles, which can efficiently produce 1O2 and NO. In addition, a highly biocidal molecule of ONOO− can also be generated in cancer cells by synergistic interaction of the PDT and the NO therapy. According to MTT results, without light irradiation, the HA-TPP@NORM nanoparticles have favourable biocompatibility. However, the nanoparticles exhibited a remarkable effect of killing HeLa cells compared with individual PDT or NO therapy when simultaneously irradiated with 365 and 650 nm light. As a result, the proposed ONOO− molecule generating the HA-TPP@NORM nanoparticles may have profound therapeutic implications for cancer therapies, and it may provide new insights with regard to NO based therapy.
Conflicts of interest
There are no conflicts to declare.
Acknowledgements
This work was supported by the Shanghai Natural Science Foundation (18ZR1408300).
Notes and references
- S. Moncada and E. A. Higgs, Br. J. Pharmacol., 2006, 147, S193–S201 CrossRef CAS.
- L. J. Ignarro, G. Cirino, A. Casini and C. Napoli, J. Cardiovasc. Pharm., 1999, 34, 879–886 CrossRef CAS.
- D. Fukumura, S. Kashiwagi and R. K. Jain, Nat. Rev. Cancer, 2006, 6, 521–534 CrossRef CAS.
- P. Pacher, J. S. Beckman and L. Liaudet, Physiol. Rev., 2007, 87, 315–424 CrossRef CAS.
- E. Nisoli, E. Clementi, C. Paolucci, V. Cozzi, C. Tonello, C. Sciorati, R. Bracale, A. Valerio, M. Francolini, S. Moncada and M. O. Carruba, Science, 2003, 299, 896–899 CrossRef CAS PubMed.
- Y. Y. Deng, F. Jia, S. Y. Chen, Z. D. Shen, Q. Jin, G. S. Fu and J. Ji, Biomaterials, 2018, 187, 55–65 CrossRef CAS PubMed.
- W. M. Xu, L. Z. Liu, M. Loizidou, M. Ahmed and I. G. Charles, Cell Res., 2002, 12, 311–320 CrossRef PubMed.
- A. W. Carpenter and M. H. Schoenfisch, Chem. Soc. Rev., 2012, 41, 3742–3752 RSC.
- S. Mocellin, V. Bronte and D. Nitti, Med. Res. Rev., 2007, 27, 317–352 CrossRef CAS PubMed.
- H. J. Lee, D. E. Kim, D. J. Park, G. H. Choi, D. N. Yang, J. S. Heo and S. C. Lee, Colloids Surf., B, 2016, 146, 1–8 CrossRef CAS.
- T. W. Liu, Z. Y. Qiao, J. L. Wang, P. Zhang, Z. D. Zhang, D. S. Guo and X. L. Yang, Colloids Surf., B, 2019, 173, 356–365 CrossRef CAS PubMed.
- I. Di Bari, G. Granata, G. M. L. Consoli and S. Sortino, New J. Chem., 2018, 42, 18096–18101 RSC.
- Z. Huang, J. Fu and Y. Zhang, J. Med. Chem., 2017, 60, 7617–7635 CrossRef CAS PubMed.
- J. A. McCleverty, Chem. Rev., 2004, 104, 403–418 CrossRef CAS PubMed.
- X. Zhang, G. Tian, W. Yin, L. Wang, X. Zheng, L. Yan, J. Li, H. Su, C. Chen, Z. Gu and Y. Zhao, Adv. Funct. Mater., 2015, 25, 3049–3056 CrossRef CAS.
- J. Fan, Q. J. He, Y. Liu, F. W. Zhang, X. Y. Yang, Z. Wang, N. Lu, W. P. Fan, L. S. Lin, G. Niu, N. Y. He, J. B. Song and X. Y. Chen, ACS Appl. Mater. Interfaces, 2016, 8, 13804–13811 CrossRef CAS.
- D. E. Kim, C. W. Kim, H. J. Lee, K. H. Min, K. H. Kwack, H. W. Lee, J. Bang, K. Chang and S. C. Lee, ACS Appl. Mater. Interfaces, 2018, 10, 26870–26881 CrossRef CAS PubMed.
- C. Hu, X. L. Cun, S. B. Ruan, R. Liu, W. Xiao, X. T. Yang, Y. Y. Yang, C. Y. Yang and H. L. Gao, Biomaterials, 2018, 168, 64–75 CrossRef CAS.
- G. Ferrer-Sueta and R. Radi, ACS Chem. Biol., 2009, 4, 161–177 CrossRef CAS.
- F. Torreilles, S. Salman-Tabcheh, M. C. Guerin and J. Torreilles, Brain Res. Rev., 1999, 30, 153–163 CrossRef CAS.
- R. Radi, A. Cassina, R. Hodara, C. Quijano and L. Castro, Free Radical Biol. Med., 2002, 33, 1451–1464 CrossRef CAS.
- C. Szabo, H. Ischiropoulos and R. Radi, Nat. Rev. Drug Discovery, 2007, 6, 662–680 CrossRef CAS.
- R. Radi, J. S. Beckman, K. M. Bush and B. A. Freeman, Arch. Biochem. Biophys., 1991, 288, 481–487 CrossRef CAS.
- Z. Du, X. Zhang, Z. Guo, J. Xie, X. Dong, S. Zhu, J. Du, Z. Gu and Y. Zhao, Adv. Mater., 2018, 30, 1804046 CrossRef.
- G. Ferrer-Sueta, N. Campolo, M. Trujillo, S. Bartesaghi, S. Carballa, N. Romero, B. Alvarez and R. Radi, Chem. Rev., 2018, 118, 462–1408 CrossRef.
- W. Fan, P. Huang and X. Chen, Chem. Soc. Rev., 2016, 45, 6488–6519 RSC.
- J. P. Celli, B. Q. Spring, I. Rizvi, C. L. Evans, K. S. Samkoe, S. Verma, B. W. Pogue and T. Hasan, Chem. Rev., 2010, 110, 2795–2838 CrossRef CAS.
- S. S. Lucky, K. C. Soo and Y. Zhang, Chem. Rev., 2015, 115, 1990–2042 CrossRef CAS.
- M. Ethirajan, Y. Chen, P. Joshi and R. K. Pandey, Chem. Soc. Rev., 2011, 40, 340–362 RSC.
- M. R. Detty, S. L. Gibson and S. J. Wagner, J. Med. Chem., 2004, 47, 3897–3915 CrossRef CAS PubMed.
- D. Dolmans, D. Fukumura and R. K. Jain, Nat. Rev. Cancer, 2003, 3, 380–387 CrossRef CAS PubMed.
- H. Jin, T. Zhu, X. G. Huang, M. Sun, H. G. Li, X. Y. Zhu, M. L. Liu, Y. B. Xie, W. Huang and D. Y. Yan, Biomaterials, 2019, 211, 68–80 CrossRef CAS.
- K. Shanmugapriya, H. Kim and H. W. Kang, Colloids Surf., B, 2019, 181, 778–788 CrossRef PubMed.
- M. D. Sun, Y. Zhang, Y. He, M. H. Xiong, H. Y. Huang, S. C. Pei, J. F. Liao, Y. S. Wang and D. Shao, Colloids Surf., B, 2019, 180, 313–318 CrossRef CAS PubMed.
- N. An, Y. Wang, M. Li, H. Lin and F. Qu, New J. Chem., 2018, 42, 18318–18327 RSC.
- J. Shen, Z. Ke, J. Chen, Z. Zou, L. Sun and D. Zou, New J. Chem., 2019, 43, 13670–13674 RSC.
- Y. Zhang, F. Wang, C. Liu, Z. Wang, L. Kang, Y. Huang, K. Dong, J. Ren and X. Qu, ACS Nano, 2018, 12, 651–661 CrossRef CAS.
- W.-H. Chen, G.-F. Luo, W.-X. Qiu, Q. Lei, L.-H. Liu, S.-B. Wang and X.-Z. Zhang, Biomaterials, 2017, 117, 54–65 CrossRef CAS.
- D. Guo, S. Xu, N. Wang, H. Jiang, Y. Huang, X. Jin, B. Xue, C. Zhang and X. Zhu, Biomaterials, 2017, 144, 188–198 CrossRef CAS.
- H. Wang, Y. Chao, J. Liu, W. Zhu, G. Wang, L. Xu and Z. Liu, Biomaterials, 2018, 181, 310–317 CrossRef CAS.
- C. Tu, L. Zhu, P. Li, Y. Chen, Y. Su, D. Yan, X. Zhu and G. Zhou, Chem. Commun., 2011, 47, 6063–6065 RSC.
- K. Lu, C. He, N. Guo, C. Chan, K. Ni, R. R. Weichselbaum and W. Lin, J. Am. Chem. Soc., 2016, 138, 12502–12510 CrossRef CAS.
- P. G. Wang, M. Xian, X. P. Tang, X. J. Wu, Z. Wen, T. W. Cai and A. J. Janczuk, Chem. Rev., 2002, 102, 1091–1134 CrossRef CAS.
- J. Fan, N. He, Q. He, Y. Liu, Y. Ma, X. Fu, Y. Liu, P. Huang and X. Chen, Nanoscale, 2015, 7, 20055–20062 RSC.
- Z. Jin, Y. Wen, Y. Hu, W. Chen, X. Zheng, W. Guo, T. Wang, Z. Qian, B.-L. Su and Q. He, Nanoscale, 2017, 9, 3637–3645 RSC.
- L. Tan, R. Huang, X. Li, S. Liu and Y.-M. Shen, Acta Biomater., 2017, 57, 498–510 CrossRef CAS PubMed.
- A. Janczyk, A. Wolnicka-Glubisz, A. Chmura, M. Elas, Z. Matuszak, G. Stochel and K. Urbanska, Nitric Oxide-Biol. Ch., 2004, 10, 42–50 CrossRef CAS PubMed.
- W. Fan, W. Bu, Z. Zhang, B. Shen, H. Zhang, Q. He, D. Ni, Z. Cui, K. Zhao, J. Bu, J. Du, J. Liu and J. Shi, Angew. Chem., Int. Ed., 2015, 54, 14026–14030 CrossRef CAS PubMed.
- Y. Zhou, J. Y. Tan, Y. P. Dai, Y. M. Yu, Q. Zhang and M. E. Meyerhoff, Chem. Commun., 2019, 55, 401–404 RSC.
- X. Jia, Y. Zhang, Y. Zou, Y. Wang, D. Niu, Q. He, Z. Huang, W. Zhu, H. Tian, J. Shi and Y. Li, Adv. Mater., 2018, 30, 1704490 CrossRef PubMed.
- M.-F. Chung, H.-Y. Liu, K.-J. Lin, W.-T. Chia and H.-W. Sung, Angew. Chem., Int. Ed., 2015, 54, 9890–9893 CrossRef CAS PubMed.
- Z. Zhang, J. Wu, Z. Shang, C. Wang, J. Cheng, X. Qian, Y. Xiao, Z. Xu and Y. Yang, Anal. Chem., 2016, 88, 7274–7280 CrossRef CAS PubMed.
- S. Meng, Z. P. Xu, G. Hong, L. H. Zhao, Z. J. Zhao, J. H. Guo, H. Y. Ji and T. J. Liu, Eur. J. Med. Chem., 2015, 92, 35–48 CrossRef CAS PubMed.
- P. V. Almeida, M. A. Shahbazi, E. Makila, M. Kaasalainen, J. Salonen, J. Hirvonen and H. A. Santos, Nanoscale, 2014, 6, 10377–10387 RSC.
- X. Xie, J. Fan, M. Liang, Y. Li, X. Jiao, X. Wang and B. Tang, Chem. Commun., 2017, 53, 11941–11944 RSC.
- D. Wang, L. Niu, Z.-Y. Qiao, D.-B. Cheng, J. Wang, Y. Zhong, F. Bai, H. Wang and H. Fan, ACS Nano, 2018, 12, 3796–3803 CrossRef CAS PubMed.
- D. E. Kim, C. W. Kim, H. J. Lee, K. H. Min, K. H. Kwack, H.-W. Lee, J. Bang, K. Chang and S. C. Lee, ACS Appl. Mater. Interfaces, 2018, 10, 26870–26881 CrossRef CAS.
Footnote |
† Electronic supplementary information (ESI) available. See DOI: 10.1039/c9nj04763h |
|
This journal is © The Royal Society of Chemistry and the Centre National de la Recherche Scientifique 2020 |