DOI:
10.1039/C8NJ05451G
(Paper)
New J. Chem., 2019,
43, 103-110
Liquid crystalline polyurethane composites based on supramolecular structure with reversible bidirectional shape memory and multi-shape memory effects†
Received
26th October 2018
, Accepted 13th November 2018
First published on 14th November 2018
Abstract
In this study, a novel series of supramolecular liquid crystalline (LC) polyurethane composites, named SMPU–#HOBA (# represents the molar ratio of HOBA/BINA), were successfully prepared by incorporating hexadecyloxybenzoic acid (HOBA) into pyridine-containing polyurethane (PU). The effect of HOBA on the structure, morphology, and properties has been carefully investigated. The results demonstrate that the obtained SMPU–#HOBA composites not only maintained the LC property of the HOBA mesogen, but also enhanced the intrinsic crystallization of the soft segment phase. Thus, the composites showed an excellent multi-shape memory property with two crystalline temperatures. Moreover, self-gripping was demonstrated revealing reversible bidirectional shape memory by virtue of molecular flipping and movement of the hard segment domain. These composites display a rare combination of LC properties, multi-shape memory properties, and reversible bidirectional shape memory properties, which makes them ideal for many potential applications in smart temperature sensitive devices.
Introduction
As intelligent stimuli-responsive materials, shape memory polymers (SMPs) have become increasingly important in many applications, such as electromechanical systems, textiles, smart optical systems and coatings.1–4 In particular, thermally responsive SMPs (TSMPs) have attracted more interest because they can be firstly deformed at a high temperature, and then fixed with a temporary shape through cooling. In contrast to conventional shape memory behavior, recent advances in TSMPs have developed tunable multi-shape memory effects, which can remember more than one temporary shape, recover the temporary shapes one by one and finally back to the original shape.5–7 In order to achieve multi-shape effects, the critical factor is integrating two reversible phases into a polymer matrix. The typical strategy of multi-shape memory polymers relies on a single broad thermal transition which could be considered to be composed of countless microscale transitions.8,9 Chen et al. successfully synthesized liquid crystalline (LC) polyurethane composites through mixing 4-n-octyldecyloxybenzoic acid (OOBA) with shape memory polyurethane, exhibiting both multi-shape memory effects and liquid-crystalline properties.10 However, most multi-SMPs only allow the temporary shape to recover once, indicated as one-way shape memory. After recovery, the next temporary shape needs an external force to be reprogrammed.
Unlike conventional one-way TSMPs, two-way TSMPs experience a reversible bidirectional shape memory effect (rbSME) upon heating and cooling with or without the need of external force for reprogramming.11–13 In 2013, Lendlein14 reported a multi-phase polyurethane matrix, which contained two domains in the crystalline phase, that exhibited an interesting stress-free rbSME. In this multiphase matrix, one crystalline segment with a high melting point served as the shifting-geometry domain while the other low-melting phase provided the actuating functionality. This is the first report about a true two-way rbSME without any external programming force. After that, substantial research has concentrated on stress-free reversible bidirectional shape memory polymers (rbSMPs).15–19 However, up to now, there are few reports about TSMPs with both multi-shape memory and reversible bidirectional effects. In addition, supramolecular polymers have been widely utilized to design unique TSMPs due to distinct stimuli-responsive supramolecular switches, such as π–π stacking,20 CD inclusion,21,22 and hydrogen bonding,23,24 opening up new territory to generate novel TSMPs. Recent advances in supramolecular polymers have resulted in triple shape memory polymers.25–27 Since Kato first reported hydrogen bonding between carboxylic acids and pyridine, carboxylic acid and pyridine complexes have been widely investigated. For instance, Ware et al. synthesized an acrylic supramolecular SMP endowed with triple shape memory functionality by virtue of self-complementary hydrogen bonds and a glass transition.28 Importantly, many liquid crystal supramolecular polymers have been utilized to design reversible bidirectional shape memory polymer.29 Wang et al. synthesized a liquid crystalline elastomer by using two-stage crosslinking, exhibiting a reversible shape memory by tuning the geometric structure of the liquid crystalline phase under external stimuli.30
According to these analyses, in this study, we develop a novel LC supramolecular polymer that represents both stress-free reversible bidirectional and multi-shape memory effects through grafting 4-hexadecyloxybenzoic acid (HOBA) chains onto shape memory polyurethane (SMPU) with pyridine as active points. Unlike a traditional simple mixture of a polymer matrix and mesogenic units,31 the grafted rigid segment reinforces the mechanical properties of SMPU. Upon repetitively heating and cooling, this thermoplastic SMPU actuates between two different shapes under stress-free conditions. The TSMP system also presents supramolecular LC properties, endowed with both polymer elasticity induced by entropy and LC ordering. This novel supramolecular LC-SMPU successfully demonstrates reversible bidirectional and multi-shape memory properties.
Experimental
Materials
N,N-Bis(2-hydroxyethyl)isonicotinamide (BINA) was received from Guangxi Sisal Company and dried beforehand at 100 °C for 3 h. HOBA was obtained from the Jiaxing Carry Biochemical Technology Co. Ltd. 1,6-Hexamethylene diisocyanate (HDI), polycaprolactone diol (PCL) (Mn = 2000), dimethylformamide (DMF) and dibutyl tin dilaurate (DBTDL) were purchased from Aladdin Chemical Reagent Co. Ltd and used without further purification.
Synthesis of SMPU
The synthesis routine is shown in Fig. 1a. SMPU was prepared by PCL, HDI, and a chain extender (BINA) via a bulk polymerization method, keeping a 1
:
1.05 molar ratio of [–OH]
:
[–NCO] and the hard segment content (HSC) 30%. For synthesizing 10 g SMPU as an example, firstly we dissolve 7 g PCL and 1.69 g HDI in a three-neck flask. The reaction was performed at 80 °C for 1 h under a nitrogen atmosphere and the flask was equipped with a mechanical stirrer, a thermometer, and a condenser. After 1 h, 1.32 g BINA and 0.02 wt% dibutyltin dilaurate (DBTDL) were added to the reaction as a chain extender and catalyst, respectively. To control the viscosity of the solution, 100 mL of DMF was added occasionally to the reaction during the whole process. The reaction was maintained for 4 h to obtain a 10 wt% SMPU/DMF solution.
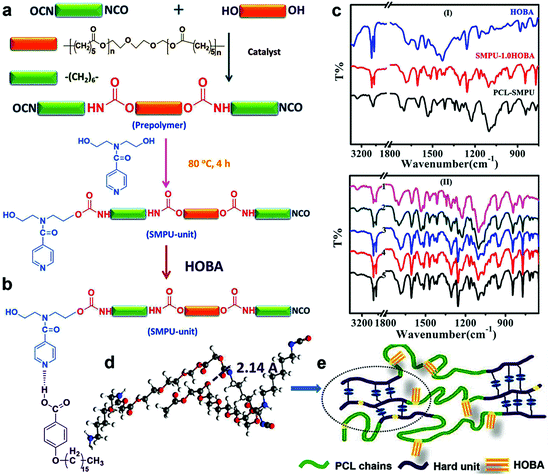 |
| Fig. 1 (a) Schematic representation of the synthesis routine of polyurethane. (b) Supramolecular structure of the SMPU–HOBA composites. (c) FTIR spectra of (I) pure SMPU, SMPU–1.0OOBA and HOBA. (II) SMPU with various HOBA (1. SMPU–0.2HOBA; 2. SMPU–0.4HOBA; 3. SMPU–0.6HOBA; 4. SMPU–0.8HOBA; 5. SMPU–1.0HOBA). (d) Simulation of the energy-minimized configuration of hydrogen bonding in SMPU considering one polyol, one diisocyanate, and one chain extender unit calculated in Chem3D Ultra 8.0. (e) Schematic representation of the hard segment region of SMPU. | |
Preparation of SMPU–#HOBA composites
According to the composition, as seen in Table S1 (ESI†), for the SMPU content of approximately 10.0 g, a certain quantity of HOBA (e.g., 1.56 g) was added to the SMPU/DMF solution and mixed for 8 h under rapid mechanical stirring to obtain a homogenous solution–phase mixture. After that, the as-synthesized SMPU/HOBA composite was obtained by casting the mixture onto a Teflon mold, which was dried at 80 °C under a vacuum of 0.1 kPa for 24 h. The reaction scheme of SMPU–HOBA composites is given in Fig. 1b. These samples were marked as SMPU–#HOBA (# represents the molar ratio of HOBA/BINA), such as SMPU–0.4HOBA.
Instruments and measurements
The FT-IR spectra were scanned from 0.2 mm thin solid films utilizing a Nicolet 6700 FT-IR spectrometer via the FT-IR attenuated total reflection (ATR) method. Ten scans were averaged at a resolution of 4 cm−1 and stored for further analysis.
Wide-angle X-ray diffraction (WAXD) experiments were performed utilizing a D8 Advance (Bruker, Germany) diffractometer with the scanning rate of 12° min−1 under an 0.154 nm X-ray wavelength. Specimens were cut to 0.5 mm thick for measurement.
DSC curves were recorded using a TA Q200 instrument with nitrogen as a purge gas. Samples were firstly heated from −50 to 150 °C at a heating rate of 10 °C min−1 and maintained at 150 °C for about 1 min. After cooling back to −50 °C at a cooling rate of 10 °C min−1, the samples were reheated to 150 °C and the cooling and reheating curves were recorded.
Mesophases were identified by utilizing a Zeiss-Axio Scope polarized optical microscope (POM) at a scan rate of 2 °C min−1. The samples were heated from 25 °C to 170 °C and cooled from 170 °C to 25 °C through a variable-temperature stage (LinkamTHMS-600).
DMA curves were determined utilizing a DMA Q800 system (TA, USA) at a heating rate of 2 °C min−1 under 1 Hz. The measured samples were cut into rectangle shapes with a thickness of 0.5 mm.
The morphology of the specimens was examined by scanning electron microscopy (SEM, Hitachi, Japan) at an accelerating voltage of 30 kV. Prior to SEM scanning, the fracture surfaces of samples were coated with sputtered gold.
Results and discussion
Structure analysis
FT-IR was employed to investigate the molecular structure of SMPU–#HOBA composites. Fig. 1c(I) shows the FT-IR spectra of HOBA, pure SMPU, and SMPU–1.0HOBA. The peak at approximately 3320 cm−1 in the spectra of the pure SMPU and SMPU–1.0HOBA corresponds to the N–H stretching vibration, exhibiting the formation of urethane groups (–NHCOO–). The O–H stretching vibration is detected at about 3450 cm−1 in the spectrum of HOBA. In addition, on comparing with the spectrum of the pure SMPU, new frequencies at approximately 1263 cm−1, 1160 cm−1, and 1055 cm−1 are detected in the spectrum of the SMPU–1.0HOBA composite, which also occur in that of HOBA. This indicates that the HOBA units have been successfully incorporated into the SMPU matrix to form SMPU–#HOBA composites. Fig. 1c(II) illustrates the spectra of all the SMPU–#HOBA composites at varying HOBA contents. However, no significant differences can be observed in the C
O vibration frequency (at 1669 cm−1) or the N–H vibration frequency (at approximately 3396 cm−1). This result confirms that the HOBA doped in the SMPU matrix does not influence the original hydrogen-bonding interactions between the C
O group and the N–H group of the urethane groups. It is well known that due to its dimerization structure through hydrogen bonding, HOBA contains a long lath-like structure with a three-ring core, displaying LC properties. In these SMPU–#HOBA composites, LC mesogens are attached to the pendant pyridine rings as side chains instead of a simple physical mixture. As a result, HOBA tends to keep the intrinsic LC properties in the SMPU matrix.
In Fig. 1d, simulation of the energy-minimized configuration reveals that the distance between the N–H moiety of the urethane linkage and ester carbonyl (C
O) of the neighboring molecule is 2.07 Å for a similar bond pair, showing the good interaction of hydrogen bonds in the hard segment region of PCL–SMPU (Fig. 1e). As shown in Fig. S1 (ESI†), the WAXD test result further confirms the supramolecular structure of the SMPU–#HOBA composites. In the case of the pure SMPU, only two broad, weak peaks are detected at 18.8° and 24.2°, assigned to (120) and (132) crystallographic planes of PCL, indicating that the pure SMPU matrix contains not only an amorphous hard phase but also a semi-crystalline soft phase of PCL. In addition, in the range of 10°–35°, the pure HOBA shows several sharp peaks, exhibiting a complete crystalline structure of HOBA.32 For the WAXD of SMPU–#HOBA composites, nearly all crystalline peaks of HOBA can be detected and the intensity increases as the HOBA content increases. Moreover, the crystalline peaks assigned to the PCL soft phase are also intensified with the increasing content of HOBA. This result reveals that HOBA improves the crystallinity of the soft phase of SMPU. Based on the above analysis, we hypothesize the supramolecular structure of the SMPU–#HOBA composites. The polymer backbone of the main matrix is composed of a HDI–BINA segment and the pedant pyridine rings of BINA units are used as H-acceptors. Strong hydrogen bonds can be formed between the carboxyl groups of HOBA and the pyridine rings in such a SMPU–#HOBA system. For increasing the content of HOBA, the intensity of the crystalline peak of SMPU–#HOBA composites increases as a result of the dimerization of higher HOBA contents, suggesting the formation of supramolecular LC structure.
Morphology analysis
Fig. 2a–d displays the SEM images of the surface of SMPU–#HOBA films containing various HOBA contents. The pure SMPU has a smooth surface without any holes (Fig. 2a), whereas after being doped with HOBA into the matrix, the surface of the composites becomes rough and more cracks can be observed with increasing HOBA content (Fig. 2b–d). The liquid crystalline property in the SMPU–#HOBA composites tends to improve with higher liquid crystal content. In Fig. 2c and d, a moderate quantity of HOBA crystals is clearly detected, demonstrating a two-phase separated structure which is composed of the amorphous SMPU matrix and the crystalline HOBA phase in the SMPU–#HOBA composites. AFM images further verify the micro-phase separated structure. The height (Fig. 2e) and phase image (Fig. 2f) of SMPU–0.6HOBA show that shaded and light cross-distribution areas exist in the micro PU matrix. In the AFM phase image (Fig. 2f), outshoots appear throughout the surface, representing the hard phase of polyurethane. In contrast, the concave parts (describing continuous phases) represent the soft phase of the PU matrix.33 These continuous repeating peaks in the phase image indicate that the PU forms and maintains a clear micro-phase separation structure, giving a morphological foundation to explain the shape memory properties.
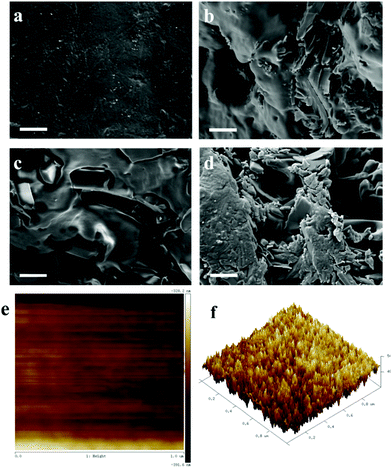 |
| Fig. 2 SEM images of (a) pure SMPU, (b) SMPU–0.2HOBA, (c) SMPU–0.6HOBA and (d) SMPU–1.0HOBA, scale bar: 20 μm. AFM images of SMPU–0.6HOBA: (e) height image; (f) phase image. | |
Thermal properties
The thermal properties of the SMPU–HOBA composites were investigated through TGA and DSC. The TG curves of SMPU–HOBA composites indicate that decomposition contains two stages, the urethane moiety at approximately 250 °C and the soft segments at 350 °C, respectively (as seen in Fig. S2a, ESI†). The decomposition temperature of the SMPU–HOBA composites appears to be identical to the pure SMPU with the retention weight increasing with the content of HOBA. This result suggests that the thermal stability is well-maintained in the composites. In addition, the DTG curves indicate that the maximum decomposition temperatures of SMPU–HOBA composites are higher than that of pure SMPU at the first stage (as seen in Fig. S2b, ESI†). A possible explanation for this result may be that the doped HOBA inhibits the decomposition degree of the polymeric-based composites, thereby improving the thermal stability. Therefore, these results confirm the excellent thermal stability of the SMPU–HOBA composites.
Fig. 3a presents the comparison of second DSC heating curves of the pure SMPU, HOBA, and SMPU–0.2HOBA. The pure SMPU shows a distinct endothermic peak at 50 °C, consistent with Tm of the PCL soft phase of SMPU. In addition, the pure HOBA crystalline exhibits two types of crystal melting peaks at approximately 20 and 105 °C with a heat-induced Tc at 65 °C. The second peak at 105 °C likely indicates a nematic–isotropic phase transition. When the HOBA is connected to the pyridine ring of the SMPU backbone to form SMPU–#HOBA composites, e.g., SMPU–0.2HOBA, the intensity of the Tm peak of the PU matrix slightly increases and cold crystallization is obviously detected at approximately 0 °C. A possible hypothesis to explain this result is that the hydrogen bonding is easily destroyed during crystallization. After cooling from high temperature, hydrogen bonding is formed gradually at lower temperature, but due to the inhibition of the polymer matrix, the incorporated low content of the HOBA mesogen needs a long crystallization time to form crystals. Therefore, only a Tm of the PCL soft phase is detected in the second heating curves in sample SMPU–0.2HOBA and no other Tm from the HOBA mesogen can be observed, whereas a thermally-induced recrystallization peak occurs at approximately 0 °C (Fig. 3a).
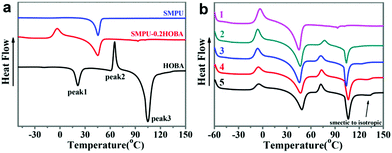 |
| Fig. 3 DSC curves of the SMPU–#HOBA composites (a) the second heating curves of HOBA, SMPU, and SMPU–0.2HOBA, (b) the second heating curves of SMPU–#HOBA composites. | |
The effects of various HOBA contents on the thermal property are presented in Fig. 3b. With the increasing HOBA content, a new phase transition occurs at 105 °C in the SMPU–#HOBA composites with over 0.4HOBA contents. This may be attributed to the smectic–nematic phase transition,34 suggesting that the smectic liquid crystal line exits in the high HOBA content samples in this temperature region. In the SMPU–1.0HOBA composites, the weak endothermic peak emerging at approximately 125 °C demonstrates the transition from the smectic to the isotropic phase of the HOBA mesogen, namely the isotropic temperature. Furthermore, from the cooling curves (Fig. S3, ESI†), the crystallization peak occurs at 65–80 °C and the crystallization peak significantly grows as the HOBA content increases. In the composites with higher HOBA contents over 0.4HOBA, apart from the second crystallization peak at about 80 °C, a weak exothermic peak is able to be observed from 100 °C to 120 °C, consistent with the phase transition from the nematic to the smectic phase. These results reveal that the liquid crystalline properties of HOBA are maintained in the SMPU–#HOBA composites. These phase transitions can be further verified by the following investigations of POM images.
Liquid crystalline properties
POM pictures intuitively show the phase transition behaviors and liquid crystalline properties of SMPU–0.8HOBA at different temperatures. In order to maintain consistency among DSC results (the second heating curve), all samples were measured at the same temperature consistent with DSC tests and then cooled back to room temperature slowly for further measurements. At 40 °C, SMPU–0.8HOBA exhibits a colorful bright crystalline texture owing to the overlapping crystallization (Fig. 4a).35,36 Upon heating to 80 °C, thermal-induced crystallization occurs in the whole area of image, showing a smectic LC phase of HOBA crystals (Fig. 4b). This result is consistent with DSC curves of pure HOBA. Moreover, when the temperature reaches 115 °C, a clear two-phase separation structure is observed. The isolated phase exhibits clear liquid crystalline texture, suggesting the good liquidity of the nematic phase of SMPU–0.8HOBA (Fig. 4c). Upon continuously heating above 115 °C, the liquid crystalline texture begins to disappear gradually, indicating that the nematic phase transfers to the isotropic state.37–39 In addition, the POM images also indicate that other crystals do not disappear although the temperature has reached 165 °C (Fig. 4d). A possible hypothesis is that these are spherical crystals of the BINA–BDO hard segment of SMPU. The existence of the crystalline hard phase can be confirmed by means of DSC tests.
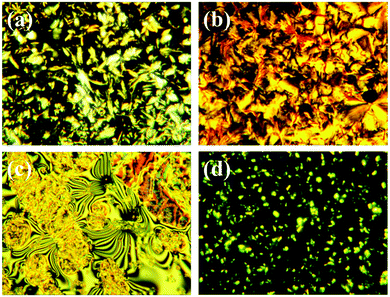 |
| Fig. 4 POM images of SMPU–0.8HOBA at different temperatures: (a) 40 °C, (b) 80 °C, (c) 115 °C, and (d) 165 °C (×400). | |
Dynamic mechanical properties
DMA measurements were performed to investigate the temperature-dependent visco-elastic properties of the synthesized composites. Fig. 5 shows the DMA curves of the pure SMPU and SMPU–#HOBA composites with various HOBA contents. As the temperature increases, all samples exhibit a high storage modulus during the glassy state, whereas a significant decrease occurs at 50 °C owing to the crystal melting of the PCL soft phase (Fig. 5a). A similar result is shown in the loss modulus text (Fig. S4, ESI†). In contrast to the pure SMPU, two rubbery state platforms emerge in the modulus curves of SMPU–#HOBA composites. It is evident that the second decrease of the storage modulus ranges from 100 to 125 °C and this decrease can be attributed to the crystal melting of the HOBA mesogen, consistent with the DSC curves. These results suggest that the HOBA mesogen provides another reversible crystal phase that acts as a reinforcement filler of the storage modulus in the rubbery state. Additionally, as shown in Fig. 5b, the tan delta curves further verify that the pure SMPU displays two peaks (a Tg and a Tm peak) of the soft phase, but the SMPU–#HOBA composites exhibit an additional transition peak at about 110 °C. Moreover, the peak intensity of Tm increases while the peak intensity of the Tg transition decreases with increasing the HOBA content. A possible explanation is that during the Tg transition the HOBA molecules incorporated onto the pyridine ring lubricate the polyurethane chains acting as a lubricant agent. On the other side, during the Tm transition the HOBA crystals serve as crystallization seeds to improve the storage loss. It is well known that multi-shape memory functionality requires multi-phase structure; the significant multi-step modulus change in DMA curves demonstrates that SMPU–#HOBA composites exemplify multiple phase transitions, indicating a potential multi-shape memory effect.
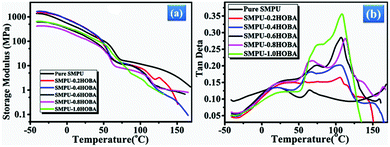 |
| Fig. 5 DMA curves of SMPU–HOBA composites: (a) storage modulus, (b) tan delta. | |
Multi-shape memory properties
The multi-shape memory effects of SMPU–#HOBA composites could be qualitatively investigated with thermo-mechanical analysis according to the test procedure reported in the literature, using a TA Instruments 800 in controlled force mode with tension clamps.8,40Fig. 6a shows the dual-shape memory property of SMPU–0.2HOBA. When the sample was deformed at 70 °C, fixed at −5 °C and recovered at 70 °C sequentially, 95.5% shape fixity and 94% shape recovery could be achieved, suggesting the good dual shape memory property. Fig. 6b illustrates the recovery process of a folded specimen in an 80 °C water bath within 2 min. In addition, the multi-shape memory property of the SMPU–0.8HOBA composite is presented in a sequential multi-stage recovery process, as shown in Fig. 6c. After fixing a deforming strain at 0 °C, the fixed strain can recover to the original shape in stages sequentially with increasing temperature from 70 °C to 80 °C, 95 °C, 110 °C, and 120 °C. Fig. 6d visually demonstrates the sequential recovery process of the SMPU–0.8HOBA composite from a one-step programmed temporary shape. Furthermore, Fig. 6e–g presents the triple-shape memory properties of SMPU–0.2HOBA, SMPU–0.4HOBA and SMPU–0.6HOBA composites, respectively. These synthesized composites were measured through two-step shape recovery cycle tests.41 Based on the DSC and DMA results, the shape memory properties at relatively low temperature should be consistent with the crystal melting of the PCL soft phase, while at a higher temperature derive from the melting of the HOBA mesogen. SMPU–0.2HOBA could be deformed with an approximately 100% strain at 80 °C, and more than 90% shape recovery occurs during the reheating process. As the HOBA content increases, the shape fixity rates in both the first and the second stages increase due to the enhanced crystallization capacity, as discussed in the DSC results. However, the strain recovery rate in the second stage is significantly lower than that in the first stage. A possible reason is that the doped HOBA mesogen interrupts the hydrogen bonding serving as a lubricating agent that results in permanent deformation and the increase of phase mixing. However, all SMPU–HOBA composites exhibit an excellent triple-shape memory effect. Accordingly, the SMPU–HOBA composites can potentially be implemented in smart temperature sensitive devices.
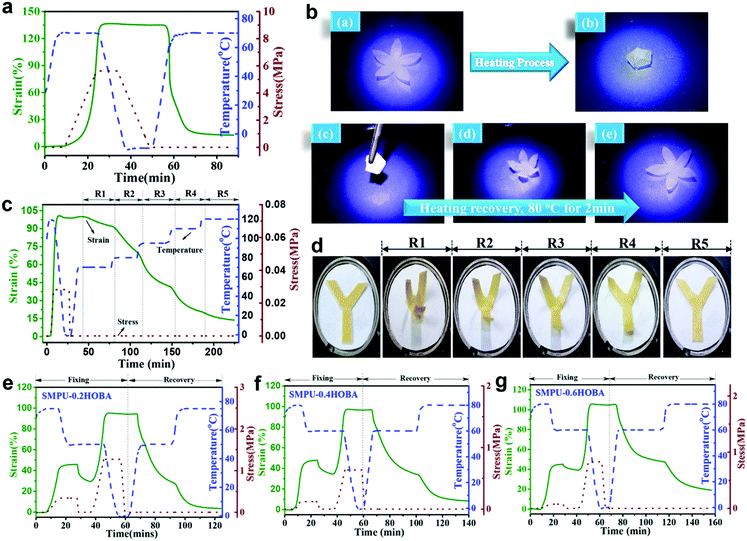 |
| Fig. 6 (a) Dual-shape memory effect of SMPU–0.2HOBA. (b) Sequential shape recovery of SMPU–0.2HOBA. (c) Multi-stage sequential shape recovery of SMPU–0.8HOBA. (d) Schematic illustration of multi-stage sequential shape recovery of SMPU–0.8HOBA. Triple shape memory effect of (e) SMPU–0.2HOBA, (f) SMPU–0.4HOBA, and (g) SMPU–0.6HOBA. | |
Reversible bidirectional shape memory properties
Fig. 7a demonstrates the rbSME for SMPU–#HOBA composites. The test was conducted at the shape giving (Tform) temperature of 90 °C and the shape fixing (Tfix) temperature of 5 °C, respectively. These strips of composites was deformed into a ring like shape at higher temperature (Tform) and fixed at lower temperature (=<Tm of the soft segment) to act as an actuator. In Fig. 7a, upon heating to a reversible temperature (Trev = 50 °C), an rbSME is detected where the ring opens up to some extent, and then goes back to the closed ring like shape after cooling to 5 °C. The gap between the two ends of an opening strip nearly reaches 0.9 cm and cyclic heating and cooling between Tform and Tfix enables the strip to reversibly switch between these two shapes (opening ↔ closing). Moreover, Fig. 7b proves its reproducibility of repeating cycles. As shown in Fig. 7c, we assembled a gripper by arranging two long strips of SMPU–0.4HOBA into a cross pattern. The gripper presented a complete closed loop at Tfix of 5 °C, then opened up at Trev of 50 °C and exhibiting its total reversibility at 5 °C again. At Trev of 50 °C, the open gripper could be above a coin and pick it up when cooled to Tfix spontaneously. The gripper released the coin upon heating to Trev of 50 °C again, showing the important capacity of a mini robot through the rbSME. The rbSME of SMPU–#HOBA composites was further quantified through cyclic, thermomechanical tensile tests. Elongation ε plotted against temperature is presented in Fig. 7d for the first reversibility cycle. Shape A is characterized by εA at high temperature, which is stabilized by the crystalline phase. When cooling to 0 °C, the polymer strip transforms to shape B, whereby the increasing elongation to εB (17%) could be ascribed to the crystallization of the oriented PCL domains. Upon heating to 50 °C, the strip restores to shape A again. Insets of Fig. 7d present the movement of the hard segment of SMPU–HOBA composites through cyclic heating and cooling. A possible hypothesis for the rbSME is that upon cooling, the hard segment of SMPU distributes toward the neighboring soft segment phase and induces the crystallization of the PCL soft phase. Hence, the cluster size decreases with increasing temperature to consolidate the hard segment, while after cooling the cluster size increases again due to the redistribution of the hard segments. Therefore, during the heating–cooling cycle, molecular flipping occurs in the hard segment accompanied by the formation of a stable pseudohexagonal phase, endowing the stability of shape A and reversible transformation of shape B, namely a reversible bidirectional shape memory effect.
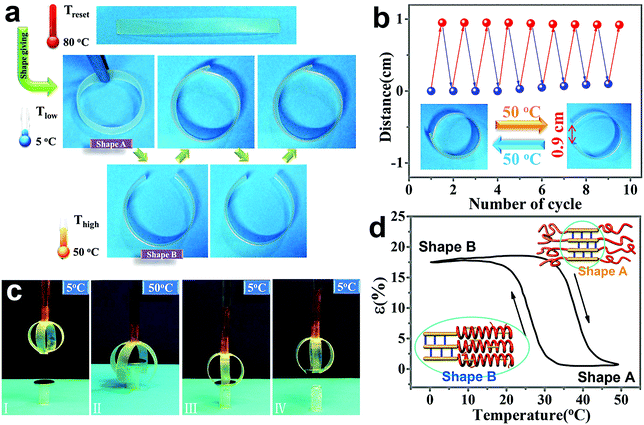 |
| Fig. 7 (a) Photograph series showing the rbSME of SMPU–HOBA composites. (b) Repeating cycles of rbSME. (c) A gripper device made from SMPU–0.4HOBA reversibly catches and releases a coin. (d) rbSME of SMPU–HOBA composites quantified in cyclic, thermomechanical tensile measurements. | |
Conclusions
In this paper, a set of supramolecular liquid crystalline polyurethane composites were successfully synthesized by incorporating the HOBA mesogen into PCL based SMPUs. Molecular structure analyses showed that HOBA was connected to the pyridine ring of the SMPU matrix via strong hydrogen bonds. The synthesized SMPU–#HOBA composites not only maintained the liquid-crystalline property of HOBA but also enhanced the intrinsic crystallization of the PCL soft phase. Shape memory investigations utilizing dynamic mechanical analysis revealed that the composites showed an excellent multi-shape memory property. In the triple-shape memory effect of SMPU–#HOBA composites, the increasing HOBA content improved the strain fixity due to the enhanced crystallinity but the strain recovery reduced as a result of the lubrication of HOBA chains. Moreover, a reversible bidirectional shape memory effect occurred for the SMPU–#HOBA composites by virtue of the hard segments not only acting as a geometry skeleton at the reversible temperature (Trev) but also enhancing crystallization of the actuator domains on cooling.
Conflicts of interest
There are no conflicts to declare.
Acknowledgements
This research was financially supported by the National Nature Science Foundation of China (51503044 and 51763009), Guangdong Province Rubber/Plastic Materials Preparation & Processing Engineering Technology Development Centre (2015B090903083), and Scientific Research Fund of Guangdong University of Petrochemical Technology (518045).
Notes and references
- J. H. Lee, R. Hinchet, S. K. Kim, S. Kim and S. W. Kim, Energy Environ. Sci., 2015, 8, 3605–3613 RSC.
- Q. Zhao, H. J. Qi and T. Xie, Prog. Polym. Sci., 2015, 49, 79–120 CrossRef.
- M. D. Hager, S. Bode, C. Weber and U. S. Schubert, Prog. Polym. Sci., 2015, 49, 3–33 CrossRef.
- Z. Yu, Q. Zhang, L. Li, Q. Chen, X. Niu, J. Liu and Q. Pei, Adv. Mater., 2011, 23, 664–668 CrossRef CAS.
- W. Li, Y. Liu and J. Leng, J. Mater. Chem. A, 2015, 3, 24532–24539 RSC.
- J. Wu, C. Yuan, Z. Ding, M. Isakov, Y. Mao, T. Wang, M. L. Dunn and H. J. Qi, Sci. Rep., 2016, 6, 24224 CrossRef.
- S. Chen, F. Mo, F. J. Stadler, S. Chen, Z. Ge and H. Zhuo, J. Mater. Chem. B, 2015, 3, 6645–6655 RSC.
- T. Xie, Nature, 2010, 464, 267 CrossRef CAS.
- K. Yu, T. Xie, J. Leng, Y. Ding and H. J. Qi, Soft Matter, 2012, 8, 5687–5695 RSC.
- S. Chen, F. Mo, Y. Yang, F. J. Stadler, S. Chen, H. Yang and Z. Ge, J. Mater. Chem. A, 2015, 3, 2924–2933 RSC.
- J. M. Raquez, S. Vanderstappen, F. Meyer, P. Verge, M. Alexandre, J. M. Thomassin, C. Jérôme and P. Dubois, Chem. – Eur. J., 2011, 17, 10135–10143 CrossRef CAS.
- S. Pandini, S. Passera, M. Messori, K. Paderni, M. Toselli, A. Gianoncelli, E. Bontempi and T. Riccò, Polymer, 2012, 53, 1915–1924 CrossRef CAS.
- K. K. Westbrook, P. T. Mather, V. Parakh, M. L. Dunn, Q. Ge, B. M. Lee and H. J. Qi, Smart Mater. Struct., 2011, 20, 065010 CrossRef.
- M. Behl, K. Kratz, J. Zotzmann, U. Nöchel and A. Lendlein, Adv. Mater., 2013, 25, 4466–4469 CrossRef CAS.
- M. Saatchi, M. Behl, U. Nöchel and A. Lendlein, Macromol. Rapid Commun., 2015, 36, 880–884 CrossRef CAS.
- Q. Zhao, W. Zou, Y. Luo and T. Xie, Sci. Adv., 2016, 2, e1501297 CrossRef.
- K. Wang, Y. G. Jia and X. Zhu, Macromolecules, 2017, 50, 8570–8579 CrossRef CAS.
- L. Lu, J. Cao and G. Li, J. Appl. Polym. Sci., 2017, 134, 45225 CrossRef.
- O. Dolynchuk, I. Kolesov, R. Androsch and H. J. Radusch, Polymer, 2015, 79, 146–158 CrossRef CAS.
- Z. Shen, Y. Jiang, T. Wang and M. Liu, J. Am. Chem. Soc., 2015, 137, 16109–16115 CrossRef CAS.
- H. Luo, Y. Liu, Z. Yu, S. Zhang and B. Li, Biomacromolecules, 2008, 9, 2573–2577 CrossRef CAS.
- M. M. Fan, Z. J. Yu, H. Y. Luo, S. Zhang and B. J. Li., Macromol. Rapid Commun., 2009, 30, 897–903 CrossRef CAS.
- M. Wei, M. Zhan, D. Yu, H. Xie, M. He, K. Yang and Y. Wang, ACS Appl. Mater. Interfaces, 2015, 7, 2585–2596 CrossRef CAS.
- M. Guo, L. M. Pitet, H. M. Wyss, M. Vos, P. Y. Dankers and E. Meijer, J. Am. Chem. Soc., 2014, 136, 6969–6977 CrossRef CAS.
- X. Le, W. Lu, J. Zheng, D. Tong, N. Zhao, C. Ma, H. Xiao, J. Zhang, Y. Huang and T. Chen, Chem. Sci., 2016, 7, 6715–6720 RSC.
- Y. Wu, J. Hu, C. Zhang, J. Han, Y. Wang and B. Kumar, J. Mater. Chem. A, 2015, 3, 97–100 RSC.
- N. Zheng, J. Hou, Y. Xu, Z. Fang, W. Zou, Q. Zhao and T. Xie, ACS Macro Lett., 2017, 6, 326–330 CrossRef CAS.
- T. Ware, K. Hearon, A. Lonnecker, K. L. Wooley, D. J. Maitland and W. Voit, Macromolecules, 2012, 45, 1062–1069 CrossRef CAS.
- S. Chen, H. Yuan, S. Chen, H. Yang, Z. Ge, H. Zhuo and J. Liu, J. Mater. Chem. A, 2014, 2, 10169–10181 RSC.
- Y. Wang, X. Huang, J. Zhang, M. Bi, J. Zhang, H. Niu, C. Li, H. Yu, B. Wang and H. Jiang, Mol. Cryst. Liq. Cryst., 2017, 650, 13–22 CrossRef CAS.
- H. Chen, Y. Liu, T. Gong, L. Wang, K. Zhao and S. Zhou, RSC Adv., 2013, 3, 7048–7056 RSC.
- J. Ban, L. Zhu, S. Chen and Y. Wang, J. Mater. Sci., 2017, 52, 2628–2641 CrossRef CAS.
- C. Tao, Z. Luo, J. Bao, Q. Cheng, Y. Huang and G. Xu, J. Mater. Sci., 2018, 53, 8639–8652 CAS.
- A. V. Emelyanenko and A. R. Khokhlov, J. Chem. Phys., 2015, 142, 204905 CrossRef CAS.
- Z. Y. Kuang, Y. J. Fan, L. Tao, M. L. Li, N. Zhao, P. Wang, E. Q. Chen, F. Fan and H. L. Xie, ACS Appl. Mater. Interfaces, 2018, 10, 27269–27277 CrossRef CAS.
- L. Tao, H. Chen, Z. Y. Kuang, C. Weng, P. Wang, N. Zhao and H. L. Xie, ACS Appl. Mater. Interfaces, 2018, 1, 4122–4129 CAS.
- J. Ban, L. Mu, J. Yang, S. Chen and H. Zhuo, J. Mater. Chem. A, 2017, 5, 14514–14518 RSC.
- Y. Guo, D. Shi, Z. W. Luo, J. R. Xu, M. L. Li, L. H. Yang, Z. Q. Yu, E. Q. Chen and H. L. Xie, Macromolecules, 2017, 50, 9607–9616 CrossRef CAS.
- J. Tang, L. Tao, H. L. Xie, Y. Guo, B. Yi, S. G. Yang, H. L. Zhang and E. Q. Chen, Polymer, 2018, 143, 137–144 CrossRef CAS.
- N. Zheng, J. Hou, Y. Xu, Z. Fang, W. Zou, Q. Zhao and T. Xie, ACS Macro Lett., 2017, 6, 326–330 CrossRef CAS.
- J. Li and T. Xie, Macromolecules, 2010, 44, 175–180 CrossRef.
Footnote |
† Electronic supplementary information (ESI) available. See DOI: 10.1039/c8nj05451g |
|
This journal is © The Royal Society of Chemistry and the Centre National de la Recherche Scientifique 2019 |