DOI:
10.1039/C8NJ04697B
(Paper)
New J. Chem., 2019,
43, 407-412
Supramolecular self-assemblies of inverted cucurbit[7]uril with biogenic amines†
Received
14th September 2018
, Accepted 14th November 2018
First published on 16th November 2018
Abstract
The interactions between inverted cucurbit[7]uril (iQ[7]) and six biogenic amines, namely tyramine (TA), 2-phenylethylamine (PEA), histamine (HI), tryptamine (TR), spermine (SPM), and spermidine (SPD), were studied through 1H nuclear magnetic resonance (NMR) spectroscopy, UV/vis absorption spectroscopy, fluorescence spectroscopy, isothermal titration calorimetry (ITC), and matrix-assisted laser desorption ionization time-of-flight mass spectrometry (MALDI-TOF MS). The results showed that iQ[7] exhibits binding affinity towards five of these biogenic amines, with the exception of HI, and that the host–guest binding sites differ depending on the structure of the biogenic amine. For the aromatic amines (TA and PEA), the phenyl groups are completely encapsulated within iQ[7]; for the heterocyclic aromatic amine TR, there is deep inclusion of its indole group in the cavity of iQ[7]; for the fatty amines (SPM and SPD), the central alkyl chain is bound within the central cavity of iQ[7].
Introduction
Biogenic amines are basic nitrogenous compounds with at least one primary amine group, and they can be formed during the storage and processing of food by thermal or enzymatic decarboxylation of amino acids.1,2 In terms of biological activity, biogenic amines play major roles in brain activity, regulation of body temperature, immune response, cell growth, gastric acid secretion, stomach pH, and differentiation.3 High levels of biogenic amines in food can cause disorders such as headaches, changes in blood pressure, and nausea.3 Primary alkyl amines are also widely used in industry, despite their toxic effect on aquatic organisms and the environment.4–9 There are potential public concerns due to their physiological and toxicological effects, which have been widely studied in the past few decades. A variety of analytical methods for the separation and quantification of biogenic amines have been developed, including chromatographic (gas chromatography, liquid chromatography, high-performance liquid chromatography, and ion-exchange chromatography) and electrophoretic techniques, and UV/vis and fluorescence spectroscopies.10–14
Owing to directional, tunable, and reversible molecular recognition motifs, supramolecular self-assembly through noncovalent interactions between a receptor macrocycle and a guest has been widely used to recognize biogenic amines in recent years. On the basis of this strategy, a variety of macrocyclic hosts, including crown ethers, cyclodextrins, calix[n]arenes, and pillar[n]arenes, have been studied with regard to their interactions with biogenic amine guests.15–20
As a new class of supramolecular macrocyclic hosts, cucurbit[n]urils (Q[n]s, n = 5–8, 10, 13–15), synthesized from glycoluril and formaldehyde, consist of a highly polarizable carbonyl-rich portal and a hydrophobic interior cavity. They have attracted increasing interest in recent years because they can encapsulate small organic molecules, bio-macromolecules, metal ions, and even nanoparticles.21–31 Because of their excellent ability to bind a number of guests, several supramolecular assemblies between Q[n]s and certain biogenic amines have also been developed. Among them, only spermine (SPM), spermidine (SPD), putrescine, and other fatty amines have been widely researched with Q[n]s, whereas aromatic amines and heterocyclic aromatic amines involved in Q[n] supramolecular assemblies still remain extremely rare.32–36
Examples of a new branch of the Q[n] family, inverted cucurbit[n]urils (iQ[n]s, n = 6, 7) were synthesized and isolated by Isaacs and Kim in 2005.37 We have been greatly interested in these iQ[n]s with a reverse glycoluril unit and different properties from those of common cucurbit[n]urils. The host–guest interactions between iQ[n]s and various guests, such as p-phenylenediaminium, α,ω-alkyldiammonium, viologen derivatives, and amino acids, have been studied.38–44 In continuation of our related research, we report herein the interactions between iQ[7] and six biogenic amine guests, namely tyramine (TA), phenylethylamine (PEA), histamine (HI), tryptamine (TR), spermine (SPM), and spermidine (SPD), whose structures are shown in Fig. 1. These interactions have been probed by 1H nuclear magnetic resonance (1H NMR) spectroscopy, UV/vis absorption spectroscopy, fluorescence spectroscopy, isothermal titration calorimetry (ITC), and matrix-assisted laser desorption ionization time-of-flight mass spectrometry (MALDI-TOF MS).
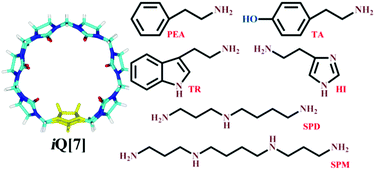 |
| Fig. 1 Structures of the host iQ[7] and the studied biogenic amine guests. | |
Results and discussion
NMR spectroscopy
The six biogenic amines selected for complexation studies with iQ[7] were divided into three groups: aromatic amines (TA and PEA), heterocyclic aromatic amines (HI and TR), and fatty amines (SPM and SPD). The aromatic amines were first examined by 1H NMR spectroscopic analysis of host–guest mixtures. Fig. 2 shows the 1H NMR spectra of PEA in D2O recorded in the absence or the presence of approximately 1.0 equiv. of the host. Fig. 2 shows that, in the presence of iQ[7], the peaks for all of the aromatic ring protons of PEA display substantial upfield shifts compared with those of the free guest. Simultaneously, the signals corresponding to the methylene protons Hd and He show downfield shifts (Δδ = 0.07 and 0.32 ppm, respectively). This evidence suggests the guest being located just outside the portal of the host.
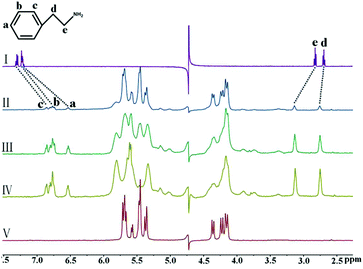 |
| Fig. 2
1H NMR spectra (400 MHz, D2O) of iQ[7] in the absence (V) and presence of 0.18 (II), 0.65 (III), and 1.09 (IV) equiv. of PEA, and of free guest PEA (I) at 20 °C. | |
The binding behavior of iQ[7] to another aromatic amine, TA, was distinct from that to PEA. As can be seen in Fig. 3, with the addition of 1.12 equiv. of iQ[7], all of the signals of the TA protons showed obvious upfield shifts compared with those of the free guest. This observation implies that the entire TA guest is located inside the cavity of iQ[7], forming an inclusion complex.
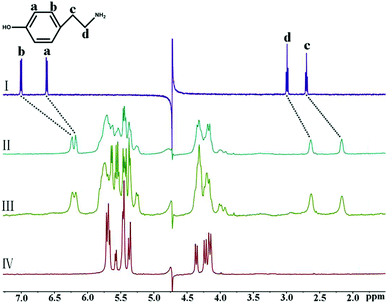 |
| Fig. 3
1H NMR spectra (400 MHz, D2O) of iQ[7] in the absence (IV) and presence of 0.56 (II) and 0.98 (III) equiv. of TA, and of free guest TA (I) at 20 °C. | |
Next, we investigated the binding interactions of the heterocyclic aromatic amines HI and TR with iQ[7]. The interaction of TR with iQ[7] was studied first, and, as clearly shown in Fig. 4, the signals of the protons Ha–He attributed to the indole ring underwent significant upfield shifts. Furthermore, the signal of the methylene protons Hg showed an obvious downfield shift from 2.88 ppm to 3.13 ppm, whereas that of the methylene protons Hf underwent a slight upfield shift from 2.88 ppm to 2.75 ppm, thus suggesting deep inclusion of the indole group and partial inclusion of the methylene moiety of the guest TR molecule in the cavity of iQ[7]. In contrast, no obvious shift was observed after mixing of the host iQ[7] with HI (Fig. S1, ESI†).
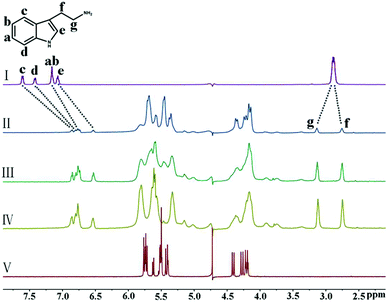 |
| Fig. 4
1H NMR spectra (400 MHz, D2O) of iQ[7] in the absence (V) and presence of 0.17 (II), 0.62 (III), and 1.08 (IV) equiv. of TR, and of free guest TR (I) at 20 °C. | |
Furthermore, the binding interactions of the fatty amines (SPM and SPD) with iQ[7] were also studied. Fig. 5 shows the 1H NMR spectra recorded for SPM, iQ[7], and the mixtures thereof. With the addition of iQ[7], the signals of the protons Ha and Hb underwent significant upfield shifts, and at the same time, those of the protons Hd and He underwent substantial downfield shifts, thus suggesting that the central diaminobutane linker of SPM was bound within the central cavity of iQ[7]. Although this was not the case for the iQ[7]-SPD system, the signals of the five methylene protons Hb–Hf of SPD underwent a substantial upfield shift in the presence of iQ[7], and that of the proton Ha underwent a significant downfield shift, and that of the proton Hg showed no obvious change. These results suggest that the central linker (CH2)2NH(CH2)3 of SPD is bound within the cavity of iQ[7], as illustrated in Fig. S2 (ESI†).
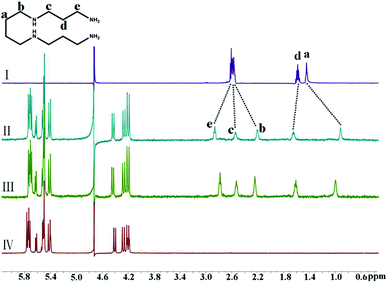 |
| Fig. 5
1H NMR spectra (400 MHz, D2O) of iQ[7] in the absence (IV) and presence of 0.66 (II) and 0.97 (III) equiv. of SPM, and of free guest SPM (I) at 20 °C. | |
UV/vis absorption and fluorescence emission spectra
To further research the supramolecular interactions of the host–guest inclusion complex, in a follow-up experiment, we examined the bonding behavior of iQ[7] and TR through spectroscopic methods (absorption and fluorescence spectroscopies). According to the UV absorption spectroscopic results, the free guest TR exhibited a maximum UV absorption at 219 nm in aqueous medium, but the host (iQ[7]) showed no absorbance ≥210 nm, as shown in Fig. 6A. After the gradual addition of iQ[7] to a solution of TR, the absorption spectra of TR progressively decreased in intensity. This phenomenon implied the bonding behavior between the host and the guest. Further support for this possibility was provided by the fluorescence spectroscopic results, as illustrated in Fig. 6B. When increasing amounts of the host iQ[7] were added to TR at a fixed concentration of 2 × 10−5 M, a decrease in the emission intensity at the maximum emission wavelength (359 nm) was observed, in agreement with the results from the UV experiments. Furthermore, the stoichiometry was confirmed by Job's plots (Fig. 6C and D). The UV and fluorescence data for the molar ratio of the host iQ[7] to the guest (NiQ[7]/NTR) fitted to a 1
:
1 binding model.
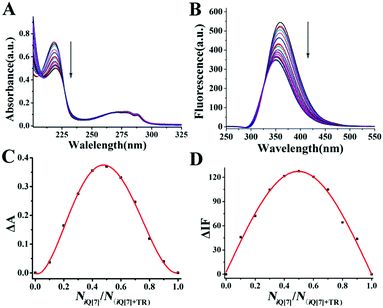 |
| Fig. 6 (A) UV absorption and (B) fluorescence spectra of TR (2 × 10−5 mol L−1) upon addition of increasing amounts (0, 0.1, 0.2⋯1.9, 2.0 equiv.) of iQ[7] and the corresponding (C) ΔA vs. NiQ[7]/NiQ[7] + NTR and (D) ΔF vs. NiQ[7]/NiQ[7] + NTR curves. | |
Isothermal titration calorimetry
To study the corresponding thermodynamic parameters of the complexes between iQ[7] and the biogenic amine guests in aqueous solution, we conducted ITC experiments at 25 °C in pure water. The titration graphs and the detailed thermodynamic parameter data are shown in Fig. S5 (ESI†) and Table 1, respectively. The experimental results revealed Ka values ranging from approximately 104 to 106 M−1 and negative ΔG° values ranging from −30.02 kJ mol−1 to −32.96 kJ mol−1. Thus, these biogenic amines effectively bound to the iQ[7] host. Among them, SPM was bound by iQ[7] with the highest binding affinity, (2.60 ± 0.12) × 106, a result consistent with the binding behavior of Q[7] to SPM.12 As can be observed from the ΔH° and TΔS° values given in Table 1, the intermolecular complexation interactions between the iQ[7] host and the biogenic amine guests SPM, SPD, and TR were evidently driven both by favorable enthalpy changes and favorable entropy changes, whereas the interactions between iQ[7] and TA and PEA appeared to be driven by favorable enthalpy changes accompanied by small negative (unfavorable) entropy changes. The ITC experiment showed no effective interaction of iQ[7] with the guest HI, and according to the NMR data, iQ[7] showed no interaction with HI. Hence, these observations are consistent.
Table 1 Thermodynamic parameters obtained by ITC for the complexation of SPM, SPD, TA, PEA, and TR by iQ[7] in aqueous media at 25 °C
Host–guest |
K
a (L mol−1) |
ΔH (kJ mol−1) |
TΔS (kJ mol−1) |
ΔG (kJ mol−1) |
iQ[7] -SPM |
(2.60 ± 0.12) × 106 |
−6.16 ± 0.34 |
26.80 |
−32.96 |
iQ[7]-SPD |
(3.33 ± 0.31) × 105 |
−9.96 ± 0.02 |
21.55 |
−31.51 |
iQ[7]-TA |
(5.51 ± 0.80) × 105 |
−33.05 ± 0.78 |
−0.28 |
−32.77 |
iQ[7]-PEA |
(1.06 ± 0.22) × 106 |
−34.09 ± 0.43 |
−1.70 |
−32.39 |
iQ[7]-TR |
(1.82 ± 0.24) × 105 |
−29.42 ± 0.89 |
0.60 |
−30.02 |
MALDI-TOF mass spectrometry
In the field of supramolecular chemistry, MALDI-TOF MS is a common and useful method for monitoring the host–guest interaction mode. As shown in Fig. 7, major obvious m/z signals were obtained for the formation of the inclusion complexes of iQ[7] and these six biogenic amine guests at m/z 1364.800, 1308.385, 1299.383, 1283.815, 1323.197, and 1273.423, which correspond to the calculated data (m/z 1365.300, 1308.210, 1300.140, 1384.140, 1323.180, and 1274.130, respectively). These intense signals provide direct support for the formation of 1
:
1 stoichiometric host–guest inclusion complexes between the iQ[7] host and these six biogenic amine guests.
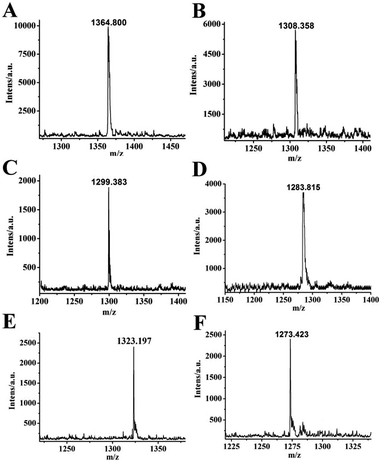 |
| Fig. 7 MALDI-TOF mass spectra of the complexes iQ[7]-SPM (A), iQ[7]-SPD (B), iQ[7]-TA (C), iQ[7]-PEA (D), iQ[7]-TR (E) and iQ[7]-HI (F). | |
Conclusions
In summary, the binding interactions between six biogenic amine guests and the iQ[7] host were investigated through a wide range of methods, including NMR spectroscopy, fluorescence spectroscopy, MALDI-TOF mass spectrometry, and ITC. The experimental results have revealed that iQ[7] shows strong binding affinity towards five of the studied biogenic amines, but not HI, and that the binding sites are different depending on the structure of the biogenic amine. For the aromatic amines (TA and PEA), the phenyl groups are completely encapsulated within iQ[7]; for the heterocyclic aromatic amine TR, there is deep inclusion of its indole group in the cavity of iQ[7]; for the fatty amines (SPM and SPD), the central alkyl chain is bound within the central cavity of iQ[7]. Overall, this study not only enhances the knowledge of the molecular recognition of biogenic amines but also may be valuable for the design and synthesis of new macrocyclic compounds for biological identification and simulation.
Methods
Materials and methods
Six biogenic amines were purchased from Aladdin and used as supplied without further purification. iQ[7] was prepared and purified according to a literature method.38 All other reagents were of analytical grade and were used as received. Double-distilled water was used for all experiments.
Isothermal titration calorimetry (ITC) experiments
All measurements were carried out on an ITC instrument (TA, U. S. A.) at 25 °C. All solutions were prepared in purified water and degassed prior to titration experiments. A typical ITC titration was carried out by titrating a biogenic amine solution against a iQ[7] solution. An aqueous solution (0.1 mM) of iQ[7] was placed in a sample cell (1.3 mL). The solution of the guest biogenic amine (1.0 mM) was added in a series of 25 injections, whereas, the solution of the guest SPM was added in a series of 20 injections (10 μL). The solutions of the guests SPD, TR, PEA, and TA were added in a series of 20–30 injections (6 μL). Computer simulations (curve fitting) were performed using the ITC software in an independent model to analyze and obtain the thermodynamic parameters of the host–guest complexes.
UV spectra and fluorescence measurements
All UV spectra were recorded using an Agilent 8453 spectrophotometer (Hewlett Packard, U. S. A) at room temperature. All fluorescence spectra were recorded using a VARIAN Cary Eclipse (Varian Inc, U. S. A) at room temperature. The solutions of iQ[7] (1.0 × 10−4 mol L−1) were prepared by directly dissolving the host in double-distilled water. The solutions of the biogenic amines (1.0 × 10−3 mol L−1) were prepared for both absorption spectral and fluorescence spectral determination. Samples of these solutions were combined to give guest
:
host ratios of 0, 0.1
:
1, 0.2
:
1, 0.3
:
1,⋯ 2
:
1 respectively. The data were analyzed using ORIGIN 8.0 and photoshop software.
1H NMR measurements
To analyze the host–guest complexation of iQ[7] and the biogenic amines, all 1H NMR spectra, including those for the titration experiments, were recorded at 20 °C on a VARIAN INOVA-400 NMR spectrometer (Agilent Technologies, U. S. A). D2O was used as a field-frequency lock and the observed chemical shifts are reported in parts per million (ppm) relative to that for the internal standard (TMS at 0.0 ppm). The ratio of the biogenic amines to iQ[7] was calculated using the ratio of their integral areas for special peaks. The concentrations of the biogenic amines are 1.0 × 10−4 mol L−1 in the 1H NMR experiments.
MALDI-TOF mass spectrometer
MALDI-TOF mass spectra were recorded on a Bruker BIFLEX III ultra-high resolution Fourier transform ion cyclotron resonance (FT-ICR) mass spectrometer with α-cyano-4-hydroxycinnamic acid as the matrix. The solution concentration is 1.0 × 10−4 mol L−1 (biogenic amines
:
iQ[7] = 1
:
1).
Author contributions
All authors had full access to all the data in the study and take responsibility for the integrity of the data and the accuracy of the data analysis. Pei-Hui Shan and Zhi-Rui Zhang have contributed equally as first authors.
Conflicts of interest
There are no conflicts to declare.
Acknowledgements
We thank the Major Program of the National Natural Science Foundation of China (NSFC, No. 21561007 and 21861011), the Creative Research Groups of Guizhou Provincial Education Department (2017-028), the Innovation Program for High-level Talents of Guizhou Province (No. 2016-5657) and the Science Technology Fund of Guizhou Province (No. 2016-1030) for financial support.
Notes and references
- M. H. S. Santos and MH Silla Santos, Int. J. Food Microbiol., 1996, 29, 213–231 CrossRef CAS.
- A. R. Shalaby, Food Res. Int., 1996, 29, 675–690 CrossRef CAS.
- B. B. Ten, C. Damink, H. M. Joosten and J. H. Huisin't Veld, Int. J. Food Microbiol., 1990, 11, 73–84 CrossRef.
- L. M. Games and R. A. Hites, Anal. Chem., 1977, 49, 1433–1440 CrossRef CAS.
- J. W. Grate, Chem. Rev., 2008, 108, 726–745 CrossRef CAS PubMed.
- F. Röck and N. Barsan, Chem. Rev., 2008, 108, 705–725 CrossRef PubMed.
- A. O. Christie and D. J. Crisp, Comp. Biochem. Physiol., 1966, 18, 59–69 CAS.
- A. O. Christie and D. J. Crisp, J. Appl. Chem., 1967, 17, 11–14 CrossRef CAS.
- H. Greim, D. Bury, H. J. Klimisch, M. Oeben-Negele and K. Ziegler-Skylakakis, Chemosphere, 1998, 36, 271–295 CrossRef CAS PubMed.
- A. Zotou and M. Notou, Anal. Bioanal. Chem., 2012, 403, 1039–1048 CrossRef CAS PubMed.
- G. I. Mohammed, A. S. Bashammakh, A. A. Alsibaai, H. Alwael and M. S. EI-Shahawi, TrAC, Trends Anal. Chem., 2016, 78, 84–94 CrossRef CAS.
- Y. Y. Chen, Z. H. Huang, H. Y. Zhao, J. F. Xu, Z. W. Sun and X. Zhang, ACS Appl. Mater. Interfaces, 2017, 9, 8602–8608 CrossRef CAS PubMed.
- M. Y. Khuhawar and G. A. Qureshi, J. Chromatogr. B: Biomed. Sci. Appl., 2001, 764, 385–407 CrossRef CAS.
- L. F. Sun, Y. Y. Ding, Y. L. Jiang and Q. Y. Liu, Sens. Actuators, B, 2017, 239, 848–856 CrossRef CAS.
- A. D’Urso, G. Brancatelli, N. Hickey, E. Farnetti, R. D. Zorzi, C. Bonaccorso, R. Purrello and S. Geremia, Supramol. Chem., 2016, 28, 499–505 CrossRef.
- J. Zhou, G. C. Yu and F. H. Huang, Chem. Soc. Rev., 2017, 46, 7021–7053 RSC.
- G. Gattuso, A. Notti, M. F. Parisi, I. Pisagatti, P. M. Marcos, J. R. Ascenso, G. Brancatelli and S. Geremia, New J. Chem., 2015, 39, 817–821 RSC.
- G. C. Yu, R. Zhao, D. Wu, F. W. Zhang, L. Shao, J. Zhou, J. Yang, G. P. Tang, X. Y. Chen and F. H. Huang, Polym. Chem., 2016, 7, 6178–6188 RSC.
- F. P. Ballistrer, A. Notti, S. Pappalardo, M. F. Parisi and I. Pisagatti, Org. Lett., 2003, 5, 1071–1074 CrossRef PubMed.
- H. Y. Zhou, Q. Y. Liu, W. M. Liu, J. C. Ge, M. H. Lan, C. Wang, J. X. Geng and P. F. Wang, Chem. – Asian J., 2014, 9, 811–818 CrossRef CAS PubMed.
- J. Kim, I. S. Jung, S. Y. Kim, E. Lee, J. K. Kang, S. Sakamoto, K. Yamaguchi and K. Kim, J. Am. Chem. Soc., 2000, 122, 540–541 CrossRef CAS.
- A. I. Day, R. J. Blanch, A. P. Arnold, S. Lorenzo, G. R. Lewis and L. Dance, Angew. Chem., 2002, 114, 285–287 CrossRef.
- H. Liu, Y. Ding, B. Yang, Z. Liu, Q. Liu and X. Zhang, Sens. Actuators, B, 2018, 271, 336–345 CrossRef CAS.
- X. J. Cheng, L. L. Liang, K. Chen, N. N. Ji, X. Xiao, J. X. Zhang, Y. Q. Zhang, S. F. Xue, Q. J. Zhu, X. L. Ni and Z. Tao, Angew. Chem., Int. Ed., 2013, 52, 7252–7255 CrossRef CAS PubMed.
- Q. Li, S. C. Qiu, J. Zhang, K. Chen, Y. Huang, X. Xiao, Y. Zhang, F. Li, Y. Q. Zhang, S. F. Xue, Q. J. Zhu, Z. Tao, F. Lindoy and G. Wei, Org. Lett., 2016, 18, 4020–4023 CrossRef CAS PubMed.
- X. Zhu, W. Chen, K. Wu, H. Li, M. Fu, Q. Liu and X. Zhang, New J. Chem., 2018, 42, 1501–1509 RSC.
- R. H. Gao, L. X. Chen, K. Chen, Z. Tao and X. Xiao, Coord. Chem. Rev., 2017, 348, 1–24 CrossRef CAS.
- Q. Liu, P. Chen, Z. Xu, M. Chen, Y. Ding, K. Yue and J. Xu, Sens. Actuators, B, 2017, 251, 339–348 CrossRef CAS.
- S. J. Barrow, S. Kasera, M. J. Rowland, J. D. Barrio and O. A. Schrman, Chem. Rev., 2015, 115, 12320–12406 CrossRef CAS PubMed.
- K. I. Assaf and W. M. Nau, Chem. Soc. Rev., 2015, 44, 394–418 RSC.
- A. E. Kaifer, Acc. Chem. Res., 2014, 47, 2160–2167 CrossRef CAS PubMed.
- Y. Y. Chen, Z. H. Huang, H. Y. Zhao, J. F. Xu, Z. W. Sun and X. Zhang, ACS Appl. Mater. Interfaces, 2017, 9, 8602–8608 CrossRef CAS PubMed.
- E. MAsson, X. Lu, X. Ling and D. L. Patchell, Org. Lett., 2009, 11, 3798–3801 CrossRef CAS PubMed.
- Y. Kim, H. Kim, Y. H. Ko, N. Selvapalam, M. V. Rekharsky, Y. Inoue and K. Kim, Chem. – Eur. J., 2009, 15, 6143–6151 CrossRef CAS PubMed.
- X. Y. He, G. Li and H. L. Chen, Inorg. Chem. Commun., 2002, 5, 633–636 CrossRef CAS.
- J. Kim, Y. Kim, K. Baek, Y. H. Ko, D. Kim and K. Kim, Tetrahedron, 2008, 64, 8389–8393 CrossRef CAS.
- L. Isaacs, S. K. Park, S. M. Liu, Y. H. Ko, N. Selvapalam, Y. Kim, H. Kim, Y. Zavalij, G. H. Kim, H. S. Lee and K. Kim, J. Am. Chem. Soc., 2005, 127, 18000–18001 CrossRef CAS PubMed.
- Z. Z. Gao, L. G. Yang, D. Bai, L. X. Chen, Z. Tao and X. Xiao, ACS Omega, 2017, 2, 5633–5640 CrossRef CAS.
- Z. Z. Gao, D. Bai, L. X. Chen, Z. Tao, X. Xiao, T. J. Prior and C. Redshaw, RSC Adv., 2017, 7, 461–467 RSC.
- Z. Z. Gao, L. G. Yang, D. Bai, L. X. Chen, Z. Tao and X. Xiao, Chin. J. Org. Chem., 2017, 38, 212–216 CAS.
- Q. Li, S. C. Qiu, Y. Q. Zhang, S. F. Xue, Z. Tao, T. J. Prior, C. Redshaw, Q. J. Zhu and X. Xiao, RSC Adv., 2016, 6, 83011 RSC.
- Q. Li, S. C. Qiu, J. H. Lu, S. F. Xue, X. Xiao, Z. Tao and Q. J. Zhu, RSC Adv., 2015, 5, 68914–68918 RSC.
- Q. Li, Y. Q. Zhang, Q. J. Zhu, S. F. Xue, Z. Tao and X. Xiao, Chem. – Asian J., 2015, 10, 1159–1164 CrossRef CAS PubMed.
- X. X. Wang, K. Chen, F. F. Shen, Z. Y. Hua, S. C. Qiu, Y. Q. Zhang, H. Cong, Q. Y. Liu, Z. Tao and X. Xiao, Chem. – Eur. J., 2017, 23, 16953–16956 CrossRef CAS PubMed.
Footnote |
† Electronic supplementary information (ESI) available: 1H NMR, 2D COSY, and ITC results. See DOI: 10.1039/c8nj04697b |
|
This journal is © The Royal Society of Chemistry and the Centre National de la Recherche Scientifique 2019 |
Click here to see how this site uses Cookies. View our privacy policy here.