DOI:
10.1039/C8NJ04127J
(Paper)
New J. Chem., 2019,
43, 295-303
In situ formation of AuNPs using fatty N-acylamino hydrazide organogelators as templates†
Received
13th August 2018
, Accepted 15th November 2018
First published on 19th November 2018
Abstract
This work reports, for the first time, the synthesis of new fatty N-acylamino hydrazides and demonstrates the activity of these compounds as low-molecular-weight organic gelators and templates for preparation of gold nanoparticles (AuNPs). Initially, we evaluated the gelation properties of fatty N-acylamino hydrazides in various nonpolar and polar solvents (n-hexane, toluene, benzene, cyclohexane, and ethanol). Fatty N-acylamino hydrazide derived of the glycine and stearic acid (C18:0) did not form gels in any of the tested solvents. All other hydrazides did form gels in at least two of the organic solvents tested. The morphology of each gel was observed via scanning electron microscopy. The organogels derived from alanine, valine, and phenylalanine had translucid properties, while the serine organogels were opaque. Afterwards, the synthesis of AuNPs in the presence of the organogelator using microwave irradiation was realized. Organogelator agents reduced HAuCl4 showing plasmon band peaks between 530 and 543 nm. In addition, the method does not require a reducing agent, which is typically a potential source of contamination and toxicity. Therefore, this work confirms the importance of the hydrazide group of the new fatty N-acylamino hydrazides in gel formation and as organogelator agents for preparation of AuNPs.
Introduction
Fatty N-acylamino acid, ester, and amine derivatives are noted for their ability to gelatinize organic solvents1–5 and their role in important biological activities (Fig. 1).6,7 Moreover, these gelators selectively form gels in water8 and they are biodegradable, making them compatible with biological systems.9
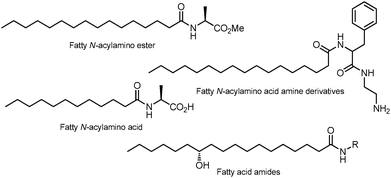 |
| Fig. 1 Fatty organogel agents. | |
These compounds belong to a class of materials called low-molecular-weight organic gelators (LMOGs), which include derivatives of long-chain hydrocarbons, salts of organic compounds, steroids, metallic complexes, and derivatives of fatty ureas and amino acids.8N-Acylamino acids and their fatty derivatives harden organic solvents at the supramolecular level, resulting in a network of three-dimensional structures. The role of these compounds in the gelation of organic solvents originates from intermolecular interactions between gelator molecules such as dipole–dipole and van der Waals forces, π-stacking, and hydrogen bond interactions.9 However, it is unclear how and why an organogelator gelatinizes one particular solvent over another.
LMOGs have attracted interest from technicians as well as academics because they are present in many commercial products (e.g., cosmetics, foods, and medicines) and are used in tissue engineering and effluent treatment.8,9N-Acylamino acid derivatives and fatty derivatives are also used in the pharmacological field as carriers of drugs.10–13 A subcutaneous implant made from the organogelator N-stearyl alanine methyl ester showed advantages over a known polymeric matrix for delivering drugs, as it was easier to prepare and nontoxic.11
In the literature, obtained nanoparticles have different applications in many fields, such as electrochemistry, electronic magnetic storage, sensors, catalysis, biotechnology and optical devices.14–16 In addition, LMOGs are also employed as templates to obtain nanoparticles.4
In this work, we describe the synthesis of new fatty N-acylamino hydrazides from fatty acids with saturated chains and commercial amino acids. The gelation properties of the new compounds were evaluated using non-polar and polar organic solvents, namely, n-hexane, toluene, benzene, cyclohexane, and ethanol. In addition, the obtained LMOGs were tested to develop a new method to obtain gold nanoparticles, using microwave irradiation.
Results and discussion
The structural design of the new fatty N-acylamino hydrazides was based on the natural availability of amino acids and fatty acids, considering their biodegradability and ability to solidify organic solvents based on previous work.5 A large number of derivatives were considered, to better clarify the mechanism of gelation and to determine the structural motifs required by these systems to exhibit efficient gelation behavior. Previous results have indicated that unsaturated chains are not favorable for the formation of gels. Thus, in this study, we synthesized new fatty N-acylamino hydrazide derivatives from saturated lipophilic chains.
Organic synthesis
Initially, the fatty N-acylamino esters 11–15a, b were synthesized from a reaction between palmitic (C16:0) and stearic (C18:0) acids with amino esters, according to previous work.5 Next, the new fatty N-acylamino hydrazides were synthesized by reacting fatty N-acylamino esters with an excess of hydrazine in acetonitrile (as the solvent) under reflux for 4 h (Scheme 1). The solids formed were washed with water to afford the compounds 16b and 17–20a, b without the need for chromatographic columns. All compounds were analyzed by 1H and 13C nuclear magnetic resonance (NMR) and infrared (IR) spectroscopic techniques and data were consistent with the expected structures.
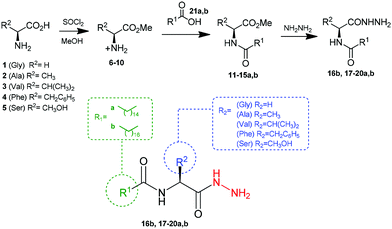 |
| Scheme 1 Synthesis of fatty N-acylamino hydrazides 16b and 17–20a, b from amino acids 1–5 and palmitic (21a, C16:0) and stearic (21b, C18:0) acids. | |
Formation of physical gels
Compounds 16b and 17–20a, b were tested for their ability to gelatinize different organic solvents. A total of 10 mg of each compound was heated in 1 mL solvent (n-hexane, toluene, benzene, cyclohexane, or ethanol) until total dissolution of the compound. Then the vial containing the mixture was cooled to 25 °C. After a few minutes, the vial was inverted to allow for the observation of the gelation effect.3,10Table 1 show the gelation abilities of the new fatty N-acylamino hydrazides 16b and 17–20a, b in organic solvents at 25 °C.
Table 1 Gelation ability of new fatty N-acylamino hydrazides 16b and 17–20a, b in different organic solvents
Entry |
Fatty N-acylamino hydrazide |
Physical characteristics |
Toluene |
Benzene |
n-Hexane |
Cyclohexane |
Ethanol |
1 |
|
Insoluble |
Insoluble |
Insoluble |
Insoluble |
Soluble |
2 |
|
Soluble |
Soluble |
Gel |
Gel |
Insoluble |
3 |
|
Gel |
Gel |
Insoluble |
Immediate precipitation |
Gel |
4 |
|
Gel |
Immediate precipitation |
Gel |
Gel |
Soluble |
5 |
|
Gel |
Gel |
Insoluble |
Insoluble |
Insoluble |
6 |
|
Gel |
Gel |
Insoluble |
Insoluble |
Gel |
7 |
|
Gel |
Gel |
Immediate precipitation |
Immediate precipitation |
Gel |
8 |
|
Gel |
Gel |
Gel |
Immediate precipitation |
Gel |
9 |
|
Gel |
Gel |
Insoluble |
Insoluble |
Insoluble |
Structure–activity relationship
The gelation ability was highly influenced by structural changes in the compounds, specifically the number of carbons present in the lipophilic chains and the nature of the substituents present in the amino acid moieties of the tested hydrazides. The glycine derivative 16b, with a C18:0 chain, was insoluble in the major solvents tested (n-hexane, toluene, benzene and cyclohexane) and did not form a gel in ethanol (Table 1, entry 1). All the other compounds synthesized (17–20a, b) in this work formed gels in some tested solvents, and all of those compounds had stereogenic centers. This finding is already described in the literature and confirms that the presence of a stereogenic center is essential for the gelation ability and molecular organization.5,18
The number of carbons in lipophilic chains showed a relationship with the gelation properties. The fatty N-acylamino hydrazide 17a, derived from valine and C16:0, formed gels in n-hexane and cyclohexane (Table 1, entry 2). The homologue 17b, from C18:0, formed gels in toluene, n-hexane, and cyclohexane (Table 1, entry 6). The same behavior was observed for the compound 19a, a derivative of phenylalanine and C16:0; it also formed a partial gel in benzene (Table 1, entry 4). Compound 19b formed gels in toluene, benzene, n-hexane, and ethyl alcohol and a partial gel in cyclohexane (Table 1, entry 8). These results suggest the influence of the carbon atom number on gelation.
The experiments also demonstrated the influence of the polarity and planarity of each solvent on the compounds’ gelation ability. In all cases involving apolar solvents was observed a similar behavior between planar solvents (benzene and toluene) and between nonplanar solvents (n-hexane and cyclohexane). Previous studies that used fatty N-acylamino esters and fatty N-acylamino acids derived from alanine, valine, phenylalanine, and serine demonstrated gelation properties only for palmitic or stearic N-acylamino acids from alanine in toluene and phenylalanine or from serine in hexane.5
In this work, replacing the ester and acid groups with a hydrazide group led to superior gelation of different solvents. The results showed that all the new fatty N-acylamino hydrazides synthesized which were derived from palmitic (C16:0) and stearic (C18:0) acids, with the exception of glycine derivatives, were able to form gels in at least two of the organic solvents tested. An explanation for this might be the effective intermolecular hydrogen bond interactions made possible with hydrazides. These interactions are more efficient than the effects of bulkier substituents attached to amino acid portions, such as the isopropyl group in derivatives of valine. Such bulky substituents prevent gel formation with fatty ester or fatty acid valine derivatives, in contrast to that observed using hydrazide valine derivatives 17a and 17b (Table 1, entries 2 and 6, respectively).
In the previous study using serine derivatives, the presence of a polar substituent in the stereogenic center is crucial for the gelation of hydrocarbons.5 This polar substituent establishes strong intermolecular interactions during the self-assembly of gelators in the derivative segment of this amino acid. The fatty N-acylamino esters used in earlier studies derived from serine were able to form gels in hexane. In the present study, fatty N-acylamino hydrazides 20a and 20b, derivatives of serine, did not form gels with hexane, cyclohexane, or ethanol but did form gels with toluene and benzene. According to the literature, L-serine amphiphiles have also been used to produce gels in CHCl3, water, and in hexane.17 Therefore, the balance between gelator structure, intermolecular interactions, and gelation properties is very important for good formation of gels.
IR spectroscopy was carried out to understand the role of the hydrazine residue and fatty chains in gelation of organic solvents. Samples of gel produced in toluene were dried under a vacuum, and the resulting solids were examined by IR spectroscopy as KBr pellets. The same compounds had previously been dissolved in 1 mL chloroform (in conditions in which they were not able to form a gel) to observe the changes in absorption bands related to intermolecular interactions (Fig. 2). IR spectra of the gel derived from fatty N-acylamino hydrazide 18a showed a strong interaction between the groups able to form hydrogen bonds, namely strong stretches of hydrazine N–H at 3284 cm−1 and carbonyl groups C
O at 1627 and 1541 cm−1. Moreover, the analysis of the IR spectra of compound 18a in CHCl3 revealed that the profile underwent great changes, showing the weakening of the intermolecular hydrogen bonds.
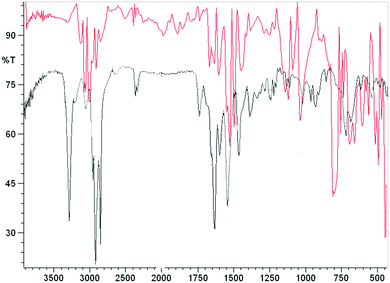 |
| Fig. 2 Infrared spectra: compound 18a in CHCl3 (red), and compound 18a in gel form (black). | |
Fig. 3 shows the importance of intermolecular interactions of hydrogen bonds in the hydrazide and amide groups for the auto-organization of three-dimensional structures of the fatty N-acylamino hydrazide organogelators.5
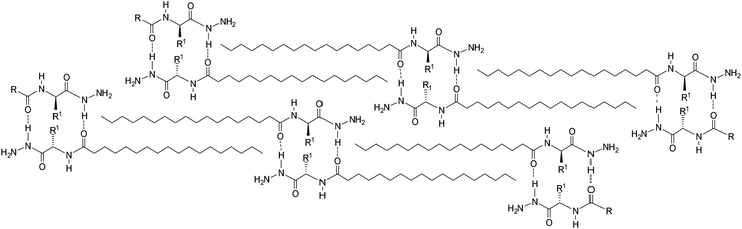 |
| Fig. 3 Physical gelation of organic solvents by fatty N-acylamino hydrazides. | |
Fig. 4 shows gels obtained from toluene with fatty organogel agents 17a, 18b and 20b. According to experimental results the organogels 17–19a, b from alanine, valine, and phenylalanine had translucid properties, while the serine organogels 20a and 20b were opaque.
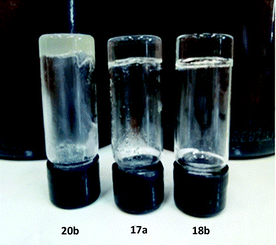 |
| Fig. 4 Organogels from compounds 20b, 17a, and 18b in toluene. | |
SEM observation of xerogels
To investigate the morphologies of the organogels, SEM was performed on the corresponding xerogels (air-dried gels). Gel samples were slowly evaporated to dryness, and then the resultant xerogels were examined. As shown in Fig. 5, the ‘preferable’ morphology adopted by the gels was tapes for compounds 17b and 18b derived from alanine and valine, respectively (Fig. 5a and b), and a more fibrous morphology for compounds 19b and 20b derived from phenylalanine and serine, respectively (Fig. 5c and d).
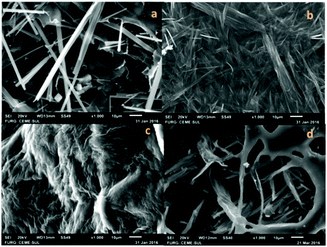 |
| Fig. 5 SEM images of air-dried organogels in toluene obtained from 17b (a), 18b (b), 19b (c), and 20b (d). | |
These observations indicate that the different morphologies of compounds 17–20b, derived from stearic acid (C18:0), depended of the structural changes in the amino acid moiety, because all were derived from the same fatty acid. In addition, compounds derived from different fatty alkyl chains and the same amino acid portion resulted in a similar morphology of the xerogel. This is confirmed by the SEM image of compound 18a derived from valine and palmitic acid (C16:0), that showed a morphology similar to 18b (Fig. 5b). In the morphological analyses conducted via SEM differences in the 3D arrangement of the materials with opaque and translucid properties were also observed (Fig. 6).
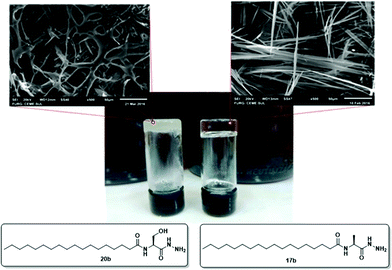 |
| Fig. 6 Different morphologies observed in opaque and translucid gels. | |
In situ preparation of AuNP containing new organogels
The new organogel fatty N-acylamino hydrazides were employed as templates for the in situ preparation of gold nanoparticles (AuNPs) using a microwave-assisted method and the resulting nanoparticles were studied using UV-visible absorption and TEM.4 The synthesized fatty compounds were employed as stabilizing agents for the nanoparticle syntheses due to the presence of long chains, derived from the fatty acids, and of the free terminal hydrazine (–NH–NH2) group that could act as a ligand towards gold, capping the AuNPs.
According to the literature, gold nanoparticles can be prepared in a simple one-pot process employing fatty alkylamine oleyl amine (OLA), where the amine group acts as a reducing agent and then subsequently stabilizes the gold particles.19 The authors suggest that the gold–amine complex is formed in the reaction procedure and its reactivity strongly depends on the nature of the amine indicating that the gold nanoparticles are produced via the Au–amine complex.
Monosubstituted fatty urea has been used for in situ preparation of gold nanoparticles. Vemula and John developed fatty urea-based aryl derivatives as gelators, which showed excellent gelation ability in a broad range of solvents from water to cyclohexane.20 Afterward, these gelators were successfully used in the synthesis of AuNPs as reducing and capping agents, showing the essential nature of the free NH2 group for the reduction and further capping process. In these experiments fatty urea derivatives were added to the HAuCl4 solution; after heating the yellow coloured solution became colourless [Au(III) to Au(I)] and then turned a pink hue, indicating the reduced form of gold. After, at room temperature a pink coloured gel with embedded AuNPs formed.
In another study, in situ preparation of gold and silver nanoparticles into a gel network structure using tyrosine containing oligopeptide based organogelators was investigated.21 The tyrosine residue of peptides were successfully utilized to reduce Au(III)/Ag(I) into colloid Au(0)/Ag(0) nanoparticles. In addition, after the reduction the gelator peptides keep their gelation properties intact.
Thus, after the formation of the gel in the presence of the organogelators 18–20a, b in toluene the metal precursor HAuCl4 was added. The mixture was placed in a microwave and after 15 min with a power of 150 W the diffusion of the salt complex in the gel matrix occurred, with a change in the coloration of the mixture to pink, indicating the formation of gold metallic nanoparticles. In all cases, a small amount of HAuCl4 salt in the organogel matrix afforded the AuNPs after exposure to microwave irradiation and the method does not require a reducing agent, which is typically a potential source of contamination and toxicity. Fig. 7 shows the preparation of the gold nanoparticles carried out with organogelator 19b.
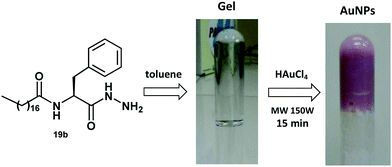 |
| Fig. 7
In situ preparation of gold nanoparticles in the presence of the organogelator 19b catalyzed by microwave irradiation. | |
In addition, an experiment to form AuNPs was also carried out, using the same protocol, in toluene using fatty N-acylamino esters and N-acylamino acids as organogelators derived from palmitic (C16:0) and stearic (C18:0) acids and alanine. However, in this case the formation of gold nanoparticles was not observed. Thus, this result shows that the free terminal hydrazine group (RC(O)–NH–NH2) of the organogelator could act as a ligand toward gold, capping the metal, and this derivative can be employed as stabilizing agents of the synthesized nanoparticles due to the presence of long chains. The –NH–NH2 group could initially capture the metal, for example, to form gold hydrazide complexes of type [RC(O)NH–NH2]/[Au(III)], followed by electron loss, involving the transfer of electrons between the –NH–NH2 group and gold ions in each step, and finally Au(0), under microwave irradiation.
UV-vis spectra of AuNPs
The reduction of transition metals in the presence of a stabilizer for the formation of metallic nanoparticles can be monitored through the spectrum in the UV-vis region. According to the literature, UV-vis absorption spectroscopy is the most widely used method for characterizing the optical properties and electronic structure of nanoparticles as the absorption bands are related to the diameter and aspect ratio of metal nanoparticles. The nanometer dimensions can oscillate on the particle surface and absorb electromagnetic radiation at a particular energy. This resonance, known as surface plasmon resonance (SPR) or plasmon absorbance, of nanoparticles is a consequence of their small size. It is well reported that the size and shape of metal nanoparticles determine the spectral position of the plasmon band, as well as its width.22,23
The UV-vis spectra of AuNPs employing as templates the organogel agents fatty N-acylamino hydrazides are shown in Fig. 8–10. According to the results the UV-vis spectra of AuNPs using organogelators 18b, 18b and 20b in toluene have plasmon bands at 532, 530 and 543 nm, respectively. The plasmon band width of agents 19b and 20b is larger than that of agent 18b indicating a higher aggregation state of nanoparticles in the presence of organogelators 19b and 20b. The characteristic SPR absorption peak indicates a larger size particle in the organogel agent 20b compared to 19b and 18b.
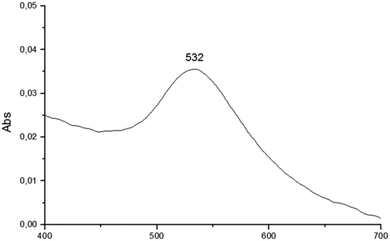 |
| Fig. 8 The plasmon absorbance of AuNPs in the presence of the organogelator 18b. | |
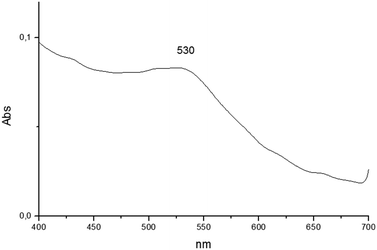 |
| Fig. 9 The plasmon absorbance of AuNPs in the presence of the organogelator 19b. | |
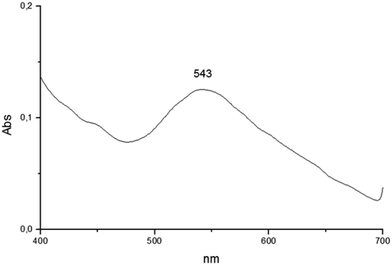 |
| Fig. 10 The plasmon absorbance of AuNPs in the presence of the organogelator 20b. | |
SEM observation of xerogels with AuNPs
SEM analysis was performed to verify the morphology of the obtained gels in the presence of gold nanoparticles. Fig. 11 shows that the morphology was not altered.
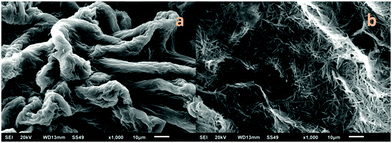 |
| Fig. 11 SEM images of air-dried organogels in toluene with nanoparticles of gold from: (a) 19b and (b) 18b. | |
TEM observation of xerogels with AuNPs
Fig. 12 shows the electron micrographs of gold nanoparticles obtained in the new organogelator fatty N-acylamino hydrazide 18b and toluene. The obtained gold nanoparticles were spheres and the average particle size was equal to 28.5 nm. Fig. 13 displays the corresponding particle size distribution of formed AuNPs obtained from the toluene gel of 18b.
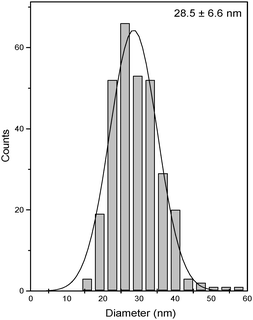 |
| Fig. 12 TEM micrographs showing the Au(0) nanoparticles obtained in toluene organogels of 18b observed at 100 kV. | |
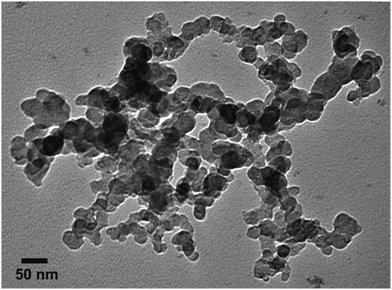 |
| Fig. 13 Histogram illustrating the particle size distribution of AuNPs obtained in the toluene gel of 18b. | |
Thus, we found that, in principle, AuNPs with a narrow particle size distribution can be prepared in the organogels, due to the presence of long chains and of the free terminal hydrazine, after exposure to microwave irradiation in the absence of a reducing agent.
Conclusions
This study reports the synthesis of new fatty N-acylamino hydrazides from renewable fatty acids with saturated chains and amino acids. The gelation properties of compounds were evaluated using five different organic solvents. Our results demonstrate the importance of including groups that form hydrogen bonds, such as hydrazides, to obtain new compounds with gelling properties. In addition, our findings further emphasize the importance of a stereogenic center to gelling, because even with the insertion of a hydrazide group, derivatives of glycine did not form gels. SEM analyses showed that the morphologies of the new compounds depended on the chemical structure, where those derived from lower fatty chains showed more compact and regular morphologies. These findings are very important for a better understanding of the gelation mechanism. In addition, the microwave-assisted synthesis of gold nanoparticles (AuNPs) using organogelator agents as templates was demonstrated. Organogelator agents reduced HAuCl4 in the absence of reducing agents, resulting in nanoparticles which showed the plasmon absorbance peak. The average size of particles was about 29 nm. Therefore, this work confirms the importance of the hydrazide group of the new fatty N-acylamino hydrazides in gel formation and as organogelator agents for preparation of AuNPs. This methodology could also be applicable for producing other metal nanoparticles.
Finally, our results show that these LMOGs can be synthesized very easily from naturally occurring sources (e.g., fatty acids and common amino acid substrates in the chemical industry), which makes them excellent materials for use in various applications.
Experimental section
Materials and methods
All solvents and reagents were obtained from commercial suppliers (Synth, Brazil; Sigma Aldrich, United States). 1H and 13C NMR spectra were recorded on a Varian VNMRS at 400 and 100 MHz, respectively, using CDCl3 and DMSO-d6 as a solvent. The chemical shifts (δ) are reported in parts per million (ppm), and the coupling constants (J) are reported in hertz (Hz). Melting points were measured on a Fisatom 430D and are uncorrected. Infrared (IR) spectra were obtained on a Shimadzu-IR PRESTIGE-21. A sample of each gel produced was dried in a vacuum and the resulting solid was examined as a KBr pellet. The morphology of the materials was analyzed by FE-SEM using a JEOL model JSM 6610 at 20 kV. Reactions were monitored by TLC with Merck silica gel 60 GF254r plates and n-hexane/EtOAc (7
:
3 v/v) eluents. The products were purified using column chromatography with ACROS silica gel 60A, 0.035–0.070 mm, and gradients of n-hexane/EtOAc eluents.
Synthetic procedure
Fatty N-acylamino hydrazides: the N-acylamino acid methyl ester (1 mmol) previously synthesized was added to a flask with 10 mL CH3CN. After, the hydrazine monohydrate (5 mmol) was added into the mixture of N-acylamino acid methyl ester and was refluxed for 4 hours. The product was purified by filtration and washed with water.
N-(Acyl-stearyl)glicinyl hydrazide (16b).
Yield 89%. White solid. M.p.: 74–75 °C. IR (KBr) cm−1: 3296, 3194, 2916, 2848, 1647, 1554, 981. 1H NMR (400 MHz, DMSO-d6): δ 0.87 (t, 3H, J = 6.8 Hz), 1.25 (m, 28H), 1.49 (m, 2H), 2.11 (t, 2H, J = 7.4 Hz), 4.32 (d, 2H, J = 5.5 Hz), 5.8 (m, 1H), 8.16 (m, 1H). 13C NMR (100 MHz, DMSO-d6): δ 9.9, 14.3, 22.4, 25.5, 29.0, 29.1, 29.2, 29.3, 31.7, 33.2, 35.5, 152.9, 173.1.
(S)-N-(Acyl-palmitoyl)alaninyl hydrazide (17a).
Yield 82%. White solid. M.p.: 117–124 °C. IR (KBr): 3307, 2918, 2848, 1737, 1639, 1573 cm−1. 1H NMR (400 MHz, CDCl3): δ 0.89 (t, 3H, J = 6.8 Hz), 1.27 (m, 28H), 1.38 (m, 3H), 1.63 (m, 2H), 2.20 (t, 2H, J = 7.6 Hz), 3.91 (m, 2H), 4.51 (m, 1H), 6.17 (d, 1H, J = 6.8 Hz), 7.79 (m, 1H). 13C NMR (100 MHz, CDCl3): δ 10.3, 14.1, 22.6, 25.6, 29.2, 29.3, 29.4, 29.5, 31.9, 49.1, 155.6, 156.8.
(S)-N-(Acyl-stearyl)alaninyl hydrazide (17b).
Yield 96%. White solid. M.p.: 139–142 °C. IR (KBr): 3307, 2918, 2848, 1737, 1639, 1573 cm−1. 1H NMR (400 MHz, CDCl3): δ 0.89 (t, 3H, J = 7.6 Hz), 1.29 (m, 28H), 1.38 (d, 3H, J = 6.9 Hz), 1.63 (m, 2H), 2.20 (t, 2H, J = 7.6 Hz), 3.89 (m, 2H), 4.51 (m, 1H), 6.17 (d, 1H, J = 6.8 Hz), 7.94 (m, 1H). 13C NMR (100 MHz, CDCl3): δ 14.0, 17.9, 22.6, 25.5, 29.2, 29.3, 29.4, 29.6, 29.6, 31.9, 36.5, 47.2, 173.0, 173.3.
(S)-N-(Acyl-palmitoyl)valinyl hydrazide (18a).
Yield 80%. White solid. M.p.: 124–131 °C. IR (KBr): 3284, 2918, 2848, 1629, 1541, 1465 cm−1. 1H NMR (400 MHz, CDCl3): δ 0.89 (t, 3H, J = 6.3 Hz), 0.96 (d, 6H, J = 5.2 Hz), 1.29 (m, 24H), 1.64 (m, 2H), 2.06 (m, 1H), 2.23 (t, 2H, J = 7.2 Hz), 3.91 (m, 2H), 4.25 (t, 1H, J = 7.9 Hz), 6.20 (d, 1H, J = 7.2 Hz), 7.92 (m, 1H). 13C NMR (100 MHz, CDCl3): δ 14.0, 18.4, 19.2, 22.6, 25.6, 29.3, 29.4, 29.6, 30.7, 31.9, 36.6, 57.0, 172.0, 173.3.
(S)-N-(Acyl-stearyl)valinyl hydrazide (18b).
Yield 88%. White solid. M.p.: 164–169 °C. IR (KBr): 3386, 2916, 2848, 1633, 1544, 1462 cm−1. 1H NMR: (400 MHz, CDCl3): δ 0.89 (t, 3H; J = 6.6 Hz), 0.96 (d, 6H, J = 5.3 Hz), 1.27 (m, 28H), 1.67 (m, 2H), 2.07 (m, 1H), 2.23 (t, 2H, J = 6.9 Hz), 3.95 (m, 2H), 4.24 (t, 1H, J = 7.8 Hz), 6.15 (d, 1H, J = 7.4 Hz), 7.78 (m, 1H). 13C NMR: (100 MHz, DMSO-d6): δ 14.3, 18.8, 19.5, 22.4, 25.7, 29.0, 29.20, 29.28, 29.3, 30.8, 31.7, 35.6, 56.9, 170.0, 172.5.
(S)-N-(Acyl-palmitoyl)phenylalanyl hydrazide (19a).
Yield 95%. White solid. M.p.: 156–161 °C. IR (KBr): 3294, 3059, 3030, 2916, 2848, 1641, 1604, 1537 cm−1. 1H NMR: (400 MHz, CDCl3): δ 0.89 (t, 3H; J = 6.8 Hz), 1.26 (m, 24H), 1.54 (m, 2H), 2.15 (t, 2H, J = 7.3 Hz), 3.06 (m, 2H), 3.84 (m, 2H), 4.71 (q, 1H, J = 7.5 Hz), 6.33 (d, 1H, J = 8.0 Hz), 7.22 (m, 5H), 7.84 (m, 1H). 13C NMR: (100 MHz, CDCl3): δ 14.1, 22.6, 25.5, 29.1, 29.35, 29.36, 29.4, 29.64, 29.66, 31.9, 36.5, 38.3, 52.9, 127.0, 128.6, 129.1, 136.4, 171.7, 173.3.
(S)-N-(Acyl-stearyl)phenylalanyl hydrazide (19b).
Yield 97%. White solid M.p.: 160–163 °C. IR (KBr): 3317, 3277, 3080, 3030, 2918, 2848, 1645, 1589, 1529 cm−1. 1H NMR: (400 MHz, CDCl3): δ 0.89 (t, 3H; J = 6.7 Hz), 1.26 (m, 28H), 1.54 (m, 2H), 2.15 (t, 2H, J = 7.4 Hz), 3.06 (m, 2H), 3.85 (m, 2H), 4.72 (q, 1H, J = 7.5 Hz), 6.37 (d, 1H, J = 8.0 Hz), 7.24 (m, 5H), 7.90 (m, 1H). 13C NMR (100 MHz, CDCl3): δ 14.1, 22.6, 25.5, 29.1, 29.34, 29.36, 29.4, 29.64, 29.67, 31.9, 36.5, 38.3, 52.9, 127.0, 128.6, 129.1, 136.3, 171.7, 173.3.
(S)-N-(Acyl-palmitoyl)serinyl hydrazide (20a).
Yield 89%. White solid. M.p.: 161–163 °C. IR (KBr): 3298, 3271, 2916, 2848, 1643, 1624, 1462 cm−1. 1H NMR: (400 MHz, DMSO-d6): δ 0.87 (t, 3H, J = 6.8 Hz), 1.27 (m, 24H), 1.50 (m, 2H), 2.14 (t, 2H, J = 7.4 Hz), 3.52 (m, 2H), 4.27 (m, 1H), 7.43 (d, 1H, J = 7.8 Hz), 8.79 (m, 1H). 13C NMR (100 MHz, DMSO-d6): δ 14.2, 22.4, 25.5, 29.0, 29.1, 29.2, 29.34, 29.37, 31.6, 35.8, 39.7, 39.9, 40.7, 40.9, 54.3, 62.3, 169.9, 172.6.
(S)-N-(Acyl-stearyl)serinyl hydrazide (20b).
Yield 92%. White solid. M.p.: 164–166 °C. IR (KBr): 3298, 3273, 2916, 2848, 1645, 1544, 1462 cm−1. 1H NMR (400 MHz, DMSO-d6): δ 0.82 (m, 3H), 1.18 (m, 28H), 1.47 (m, 2H), 2.13 (m, 2H), 3.44–3.51 (m, 2H), 4.28 (m, 1H), 7.55 (m, 1H), 8.89 (m, 1H). 13C NMR (100 MHz, DMSO-d6): δ 14.3, 22.4, 24.8, 25.5, 25.8, 29.0, 29.1, 29.2, 31.7, 33.7, 35.7, 48.0, 54.2, 62.2, 169.9, 172.6.
Preparation of the gel
Compounds 16b, and 17–20a, b were tested for their ability to gelatinize different organic solvents. A total of 10 mg of each compound was heated in 1 mL solvent (n-hexane, toluene, benzene, cyclohexane, or ethanol) until total dissolution of the compound or reflux of the solvent. Then the vial containing the mixture was cooled to 25 °C. After a few minutes, the vial was inverted to observe the gelation effect.
Preparation of the gold nanoparticles
HAuCl4 (0.05 mmol) was added to the gel formed employing organogelators (0.5 mmol) and fresh toluene (5 mL), in an appropriate 10 mL tube in a Discovery CEL Discovery & Explorer SP microwave. After complete dispersion of salt in the gel matrix, the preparation of the gold nanoparticles was conducted for 15 min under microwave irradiation in a power mode at 150 W. The resulting AuNPs were heated in toluene until total dissolution for UV-vis spectroscopy studies.
Microscopic study
SEM observation.
The morphology of the materials was analyzed by SEM using a JEOL model JSM 6610 LV operated at an accelerating voltage of 20 kV. The gel was placed on a cover slip and dried for 48 h under vacuum before imaging.
TEM observation of gold nanoparticles.
The morphology of the reported organogels and gold nanoparticles (AuNPs) was observed using TEM. Samples were prepared by depositing a drop of the gel phase gelators or AuNPs dispersed in MeOH on a TEM copper grid (200 mesh) coated with a carbon film. The grid was then dried under vacuum at room temperature overnight. Images were obtained using a JEOL JEM-1400 electron microscope operating at 100 kV. The histograms of the nanoparticle size distribution were obtained from measurements of around 300 diameters and reproduced in different regions of the Cu grid assuming spherical shapes.
Conflicts of interest
The authors declare no conflict of interest.
Acknowledgements
The authors are thankful for financial support from Fundação de Amparo à Pesquisa do Estado do Rio Grande do Sul (FAPERGS/PRONEM 11/2069-0 and PRONEX 16/2551-0000481-1), and Conselho Nacional de Desenvolvimento Científico e Tecnológico (CNPq). Fellowships from CNPq and Coordenação de Aperfeiçoamento de Pessoal de Nivel Superior (CAPES) are also acknowledged.
References
- K. J. Skilling, F. Citossi, T. D. Bradshaw, M. Ashford, B. Kellam and M. Marlow, Soft Matter, 2014, 10, 237 RSC.
- J. F. T. Vazquez, J. M. Rueda, V. A. Mallia and R. G. Weiss, Food Biophys., 2010, 5, 193 CrossRef.
- S. Bhattacharya and A. Pal, J. Phys. Chem. B, 2008, 112, 4918 CrossRef CAS PubMed.
- F. Delbecq, K. Tsujimoto, Y. Ogue, H. Endo and T. Kawai, J. Colloid Interface Sci., 2013, 390, 17 CrossRef CAS PubMed.
- R. C. Duarte, R. F. Ongaratto, L. A. Piovesan, V. R. Lima, V. Soldi, A. A. Merlo and M. G. M. D’Oca, Tetrahedron Lett., 2012, 53, 2454 CrossRef CAS.
- C. R. M. D’Oca, T. Coelho, T. G. Marinho, C. R. L. Hack, R. C. Duarte, P. A. Silva and M. G. M. D’Oca, Bioorg. Med. Chem. Lett., 2010, 20, 5255 CrossRef PubMed.
- S. Burstein and R. Salmonsen, Bioorg. Med. Chem., 2008, 16, 9644 CrossRef CAS PubMed.
- S. Bhattacharya and P. Y. K. Ghosh, Chem. Commun., 2001, 185 RSC.
- F. Plourde, A. Hoarau, A. C. C. Hoarau, D. Hoarau, H. Ong and J. C. Leroux, J. Controlled Release, 2005, 108, 433 CrossRef CAS PubMed.
- S. Bhattacharya and P. Y. K. Ghosh, Tetrahedron, 2007, 63, 7334 CrossRef.
- N. M. Sangeetha and U. Maitra, Chem. Soc. Rev., 2005, 34, 821 RSC.
- A. Vintiloiu and J. C. Leroux, J. Controlled Release, 2008, 125, 179 CrossRef CAS PubMed.
- A. Vintiloiu, M. Lafleur, G. Bastiat and J. C. Leroux, Pharm. Res., 2008, 25, 845 CrossRef CAS PubMed.
- L. Liu and A. Corna, Chem. Rev., 2018, 118, 4981 CrossRef CAS PubMed.
- V. M. Rotello, A. Verma and C. C. You, Soft Matter, 2006, 2, 190 RSC.
- A. C. C. Hoarau, A. Motulsky, P. Delmas and J. C. Leroux, Pharm. Res., 2004, 21, 454 CrossRef PubMed.
- L. Y. G. Lim, Y. Su, F. Braet and P. Thordarson, Aust. J. Chem., 2009, 62, 653 CrossRef CAS.
- B. Weiss, J. Org. Chem., 1959, 24, 1367 CrossRef CAS.
- M. Aslam, L. Fu, M. Su, K. Vijayamohananb and V. P. Dravid, J. Mater. Chem., 2004, 14, 1795 RSC.
- P. K. Vemula and G. John, Chem. Commun., 2006, 2218 RSC.
- S. Ray, A. K. Das and A. Banerjee, Chem. Commun., 2006, 2816 RSC.
- D. Philip, Spectrochim. Acta, Part A, 2008, 71, 80 CrossRef PubMed.
- S. Behzadi, F. Ghasemi, M. Ghalkhani, A. A. Ashkarran, S. M. Akbari, S. Pakpour, M. R. Hormozi-Nezhad, Z. Jamshidi, S. Mirsadeghi and R. Dinarvand, Nanoscale, 2015, 7, 5134 RSC.
Footnote |
† Electronic supplementary information (ESI) available: NMR 1H and 13C spectra and TEM and SEM micrographs. See DOI: 10.1039/c8nj04127j |
|
This journal is © The Royal Society of Chemistry and the Centre National de la Recherche Scientifique 2019 |
Click here to see how this site uses Cookies. View our privacy policy here.