DOI:
10.1039/C8NJ03776K
(Paper)
New J. Chem., 2019,
43, 146-153
Immobilization of enzymes on an organic–inorganic hybrid network consisting of Dawson-type polyoxotungstate and a zinc(II)-biimidazole complex moiety†
Received
29th July 2018
, Accepted 12th November 2018
First published on 13th November 2018
Abstract
A new polyoxometalate (POM)-based organic–inorganic hybrid compound, {[(Zn(H2biim)2)3(P2W18O62)]·6H2O}n (1) (H2biim = 2,2′-biimidazole), has been hydrothermally synthesized and characterized by physico-chemical and spectroscopic methods. The structure of compound 1 exhibits a two-dimensional (2-D) network character, in which each Dawson-type polyoxoanion [P2W18O62]6− ({P2W18}) is connected with six [Zn(H2biim)2]2+ ({Zn(II)-H2biim}) complex fragments, while each {Zn(II)-H2biim} unit bridges two {P2W18} clusters, forming a 2-D network with a (6,3)-net topology. Compound 1 shows high enzyme-immobilizing capability for horseradish peroxidase (HRP). The maximum HRP load is 90.1 mg g−1 under optimal conditions. Furthermore, the immobilized enzyme (HRP/1) exhibits high catalytic activity and good stability, which can be applied to detect trace amounts of H2O2 in solution with a low detection limit of 3.11 × 10−3 mmol L−1.
Introduction
Enzymes are significant biological catalysts and have many advantages in catalytic reactions such as high activity, good specificity and mild reaction conditions. However, the development of enzyme catalysts has always been limited due to their high cost and the realistic difficulties of separation and recycling in catalytic reactions.1 Therefore, immobilizing enzymes on various supports is an important strategy to improve the recovery and reuse efficiency of enzymes.2,3 Polyoxometalates (POMs) are a unique type of inorganic multidentate oxo-ligand and can be modified by transition metal (TM) ions and/or metal–organic coordination units, generating versatile structures with high thermal and chemical stabilities.4–6 Most importantly, POM materials have a highly negatively charged oxygen-rich surface that could have strong affinity for soluble proteins or enzymes induced by both electrostatic interactions and hydrogen bonds.7,8 Using POM materials as supports for enzyme immobilization is a new interesting approach. Recently, two new Preyssler-type POM-based organic–inorganic hybrid materials have been employed as supports to immobilize horseradish peroxidase (HRP),7 which reflects the advantages and potential applications of POMs in immobilization of enzymes. Furthermore, organic ligands can also be used as both hydrogen bond donors and acceptors, strengthening the hydrogen bonding interactions between enzyme and hybrid materials. Based on these characteristics, we have an opportunity to explore the potential capability of POM-based organic–inorganic hybrid materials in enzyme immobilization. Dawson-type POMs also possess high affinity for amino acids and proteins,9 which may make them a new candidate for the construction of enzyme-immobilizing hybrid materials. 2,2′-Biimidazole (H2biim) ligand and its metal complex moieties also have good biocompatibility.10,11 The combination of Dawson-type POM polyoxoanion and H2biim or TM-H2biim may yield a new type of enzyme-immobilizing support.
To the best of our knowledge, hybrid materials based on Dawson-type POM and TM-H2biim units have rarely been reported yet. One reason is that the Dawson-type POMs usually exist under strong acidic conditions, where H2biim is easily protonated rather than preferentially coordinated with TM ions, and can further form precipitates. Another reason is that the difference in solubility between Dawson-type POMs and TM-H2biim coordination units in aqueous solution leads to the relatively difficult self-assembly of these two building blocks. In this respect, the current related research is that Zhang et al. have reported a successful example that is based on the {Zn(II)-H2biim} complex fragments and Strandberg-type POM units.12 Considering that Strandberg-type and Dawson-type POMs have good stability within a similar pH range, we have introduced the Dawson-type POM into a similar reaction system and successfully synthesized the first organic–inorganic hybrid material constructed from Dawson-type POM and {Zn(II)-H2biim} units, namely {[(Zn(H2biim)2)3(P2W18O62)]·6H2O}n (1). Compound 1 possesses a 2-D network character and exhibits a high loading ability of HRP. Significantly, the immobilized HRP on compound 1 (HRP/1) showed high catalytic activity and good cyclic stability in the model reaction of H2O2 co-oxidation of phenol and 4-aminoantipyrine (4-AAP), which can be potentially applied in the detection of trace amounts of H2O2.
Experimental
Materials and methods
K6[α-P2W18O62]·14H2O (abbreviated as K-{α-P2W18}) was synthesized according to the literature.13 HRP (horseradish peroxidase, isoenzyme C, product P105528, lot K1506050, E.C. 1.11.1.7, 300 U mg−1, RZ > 3.0, Mw ≈ 40 kDa) and 4-AAP were purchased from Aladdin Biochem Technology Co. Ltd, P.R. China. All other chemicals were purchased commercially and used without further purification. Phosphate buffer solution (PBS) (0.1 mol L−1) with different pH values (3.5–8.5) was prepared by using Na2HPO4, NaH2PO4, or H3PO4. Immobilized enzyme HRP/1 was isolated from the mixed solution of HRP and compound 1 by centrifuging using a TG16-WS centrifuge. Single crystal X-ray diffraction data were collected on a Bruker Smart APEX IIX-diffractometer (Bruker, Germany) equipped with Mo-Kα radiation (λ = 0.71073 Å), and powder X-ray diffraction (PXRD) was measured on a Bruker AXS D8 powder diffractometer (Bruker, Germany) equipped with Cu-Kα radiation (λ = 1.5418 Å). IR spectra were recorded in the range of 4000–400 cm−1 with a Bruker AXS TENSOR-27 FT-IR spectrometer (Bruker, Germany) using KBr pellets. C, N and H elemental analyses were performed on a Vario Elcube elemental analyser (Elementar, Germany), and P, Zn and W were analysed on a Prodigy XP emission spectrometer (LEEMAN, United States). Thermal analysis (TGA-DTA) was performed on a Pyris Diamond TG-DTA thermal analyser at a heating rate of 10 °C min−1 in air. Liquid UV-vis absorption spectra were recorded on a Lambda 35 UV-vis spectrophotometer. Confocal laser scanning microscopy (CLSM) images were recorded on a Zeiss LSM 710 confocal microscope, the detection wavelength was λ = 543 nm for rhodamine B isothiocyanate (RhBTC). The circular dichroism (CD) spectra were obtained on a MOS-500 automatic recording spectro-polarimeter (Bio Logic, France) in the wavelength range from 190 to 250 nm at room temperature, using a 2 mm cell with a scan rate of 1.0 s nm−1. Differential scanning calorimetry (DSC) was performed on a NETZSCH STA 449 F3 thermal analyzer under a nitrogen atmosphere using a rate of temperature increase of 10 °C min−1.
Synthesis of {[(Zn(H2biim)2)3(P2W18O62)]·6H2O}n (1)
A mixture of ZnSO4·7H2O (0.075 g, 0.25 mmol), H2biim (0.070 g, 0.50 mmol) and distilled water (7.5 mL) was stirred for 10 minutes, and pH was adjusted to the range of 6.0–6.5 with 3 mol L−1 HCl under continuous stirring. Then, a solution of K-{α-P2W18} (0.400 g, 0.085 mmol) in distilled water (5.0 mL) was added. The resulting solution was sealed in a 20 mL Teflon-lined autoclave and kept at 180 °C for 3 days. After cooling slowly to room temperature, deep-red block crystals of 1 (yield: ca. 70% based on W) were isolated. Elemental analysis, calcd for C36H48N24O68Zn3P2W18: C, 7.90; H, 0.88; N, 6.14; P, 1.13; Zn, 3.58; W, 60.46%; found: C, 7.78; H, 0.98; N, 6.21; P, 1.19; Zn, 3.61; W, 60.50%. FTIR (KBr pellet), cm−1: 3632(m), 3408(m), 3313(m), 3143(w), 1633(m), 1527(m), 1399(m), 1320(w), 1240(w), 1186(w), 1091(s), 937(s), 804(s), 709(s), 666(s), 539(m) and 480(m).
In the synthetic process of compound 1, if the pH was adjusted to below 6.0, another product, red block crystals of [H6(H2biim)6(P2W18O62)]·8H2O (2) (yield: ca. 50% based on W) were isolated.
X-ray crystallography
The diffraction data of compounds 1 and 2 were collected within the scope of 2.613° ≤ θ ≤ 29.788° and 1.68° ≤ θ ≤ 27.41°, respectively. The structures were solved by direct methods and refined by full-matrix least-squares fitting on F2 using SHELXTL-2018.14 Hydrogen atoms on C and N atoms were added in calculated positions, while hydrogen atoms on H2O molecules were directly added in the final formula. Crystal data and structure refinement parameters of compounds 1 and 2 are listed in Table 1. The selected bond lengths and angles, and hydrogen bonds are given in Tables S1–S4 (ESI†). CCDC reference numbers are 1441744 and 1851431.†
Table 1 Crystal and refinement data for compounds 1 and 2
Compound |
1
|
2
|
R
1 = ∑||F0| − |FC||/∑|F0|; wR2 = ∑ [w(F02 − FC2)2]/∑[w(F02)2]1/2.
|
Formula |
C36H48N24O68Zn3P2W18 |
C36H58N24O70P2W18 |
Formula weight |
5472.33 |
5318.30 |
T/K |
296(2) |
296(2) |
Wavelength/Å |
0.71073 |
0.71073 |
Crystal system |
Triclinic |
Triclinic |
Space group |
P![[1 with combining macron]](https://www.rsc.org/images/entities/char_0031_0304.gif) |
P![[1 with combining macron]](https://www.rsc.org/images/entities/char_0031_0304.gif) |
a/Å |
14.6698(9) |
13.8898(15) |
b/Å |
14.9855(9) |
16.0392(17) |
c/Å |
24.7793(15) |
23.038(2) |
α/° |
98.577(1) |
76.838(2) |
β/° |
97.653(1) |
87.770(2) |
γ/° |
118.761(1) |
68.781(2) |
V/Å3, Z |
4588.1(5), 2 |
4653.4(9), 2 |
D
c/g cm−3, F000 |
3.961, 4856 |
3.796, 4728 |
GOF |
1.009 |
1.029 |
Reflections collected |
23 345 |
23 476 |
Unique data, Rint |
12 418, 0.0286 |
13 158, 0.0481 |
θ Range(°) |
1.60 to 25.00 |
1.68 to 25.00 |
R
1(I > 2σ(I))a |
0.0433 |
0.0610 |
wR2 (all data)a |
0.1191 |
0.1736 |
Immobilization of HRP
According to the reported method,7,15 we selected HRP as a model enzyme and compound 1 as a support to prepare the immobilized enzyme (HRP/1) by a direct adsorption method. The immobilizing process was as follows: 5.0 mg of a powdered sample of compound 1 was added to 500 μL of 0.1 mol L−1 PBS at pH 3.5–8.5 containing different concentrations of HRP, and the resulting mixture was stirred at room temperature for a certain period of time, and then centrifuged. The obtained supernatant was checked by UV-vis spectroscopy at λ = 403 nm, and the residue was washed three times with PBS to ensure complete removal of unloaded HRP, and thus HRP/1 was obtained. Furthermore, the optimal conditions of HRP immobilization on compound 1 including pH, HRP concentration and immobilization time were investigated.
While for compound 2, because it can easily dissolve in the reaction system, it is not suitable to be utilized as a support for immobilizing enzymes, and it will not be discussed here.
Assay of the immobilized HRP activity
To evaluate the catalytic activity of HRP/1, the model color-reaction of phenol with 4-AAP catalyzed by enzyme in the presence of H2O2, which is known as the Worthington method, was used.7,16–18 The colorimetric assay was carried out as follows: using 0.1 mol L−1 PBS (pH 3.5–8.5) as a solvent, 1.5 mL of 2 mmol L−1 H2O2 was added to 1.4 mL of mixed liquor containing 172 mmol L−1 phenol and 2.46 mmol L−1 4-AAP, and 0.5 mg HRP/1 catalyst was added into the solution. Then, the absorbance of the above mixture was tested at λ = 510 nm. The intensity of the red product coloring in the catalytic reaction is proportional to the concentration, which can reflect the catalytic activity of the immobilized HRP, so it can be monitored spectrophotometrically by tracing the formation of the red-colored product. In addition, the turnover frequency (TOF, molproduct molcatalyst−1 s−1) of the catalyst was calculated to determine the activity of immobilized HRP. The catalytic activity of free HRP with the same quantity as the corresponding amount of immobilized HRP was also evaluated for comparison.
Results and discussion
Synthesis
Based on a large number of experiments, it was discovered that the choice of starting reaction materials and the selection of the optimal synthetic conditions including pH, molar ratio, temperature and reaction time are very important for the formation of Dawson-type POM-based hybrid material modified by TM-H2biim units. Furthermore, the crystalline product is extremely difficult to obtain with conventional synthetic methods, in aqueous solution. But, a hydrothermal synthetic method can cause a mixture of powder materials to undergo the process of “dissolution–nucleation–crystallization”, and finally the crystals can be prepared. We selected the Dawson-type POM K-{α-P2W18} as a starting material, and the molar ratio of Zn2+ and H2biim was controlled at 1.0
:
2.0. After the reaction temperature was kept at 180 °C for 3 days, compound 1 was obtained (Scheme 1, route I). Interestingly, compound 1 can only be gained in a very narrow pH range (6.0–6.5). During dropwise addition of 3 mol L−1 HCl solution, it can be observed that H2biim ligand is just dissolved completely at pH = ca. 6.0. If more HCl solution is added to the reaction solution with low pH (<6), another crystalline product, i.e. compound 2, will be obtained as the main product (Scheme 1, route II). Moreover, if selecting Zn(H2biim)2 complex powder as the starting material, no single crystals but fine crystalline powders were isolated from the reaction system. Thus, different structural assembly will happen although using the same inorganic and metal–organic units under the same hydrothermal conditions, which is influenced by a few of the reaction factors; especially, the pH is one of the most important factors.
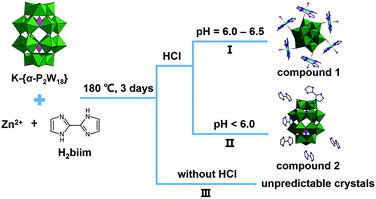 |
| Scheme 1 Schematic representation of the isolation of the hybrid compounds based on Dawson-type POM and {Zn(II)-H2biim} coordination units. | |
Crystal structure
Single crystal X-ray diffraction analysis revealed that compound 1 is composed of {P2W18} polyoxoanions, {Zn(II)-H2biim} coordination fragments, and lattice water molecules (Fig. S1, ESI†); every six {Zn(II)-H2biim} coordination moieties are coordinated with one {P2W18} anion via the Zn–O coordination bonds (Fig. 1a). In the crystal structure of 1, all Zn(II) centers exhibit the ZnN4O2 octahedral coordination environment (Fig. 1a and b). Zn1 coordinates with four N atoms (N1, N2, N3 and N4) of two H2biim molecules and two terminal O atoms (O57, O59) from two {P2W18} anions, respectively (Fig. 1b and Fig. S2, ESI†). The bond lengths of Zn1–O57 and Zn1–O59 are 2.182(9) Å and 2.314(9) Å, respectively, and those of Zn1–N are in the range of 2.067(14)–2.159(15) Å (Table S1, ESI†). Zn2 and Zn3 centers also coordinate with four N atoms from two H2biim ligands and two terminal O atoms (O5/O5A and O38/O38A; O30/O30A and O61/O61A) of two {P2W18} units, respectively. The bond lengths of Zn2–O5 and Zn2–O38 are 2.323(9) and 2.209(10) Å, and those of Zn3–O30 and Zn3–O61 are 2.267(10) and 2.566(10) Å, respectively. The Zn2–N and Zn3–N bond lengths are in the range of 2.096(16)–2.125(13) Å and 2.025(14)–2.162(14) Å, respectively (Table S1, ESI†). Based on the above coordination modes, all {Zn(II)-H2biim} complex fragments are connected with the equatorial sites of the Dawson-type POM units, exposing the polar trimetallic fragments of POM clusters (Fig. S2, ESI†). More interestingly, every {Zn(II)-H2biim} unit bridges two {P2W18} clusters, and each {P2W18} anion links with the other six {P2W18} anions through the {Zn(II)-H2biim} bridges, forming a 2-D network with a (6,3)-net topology (Fig. 1c). To our knowledge, such a Dawson-type POM-based 2-D network with (6,3)-net topology is reported for the first time. These 2-D layers are further stacked into a three-dimensional (3-D) structure (Fig. 1d) by virtue of hydrogen-bonding interactions. The typical intermolecular hydrogen bonds of N–H…O/OW are 2.790(19)–3.21(2) Å (Table S2, ESI†). Lattice water molecules are scattered in the voids of the structure.
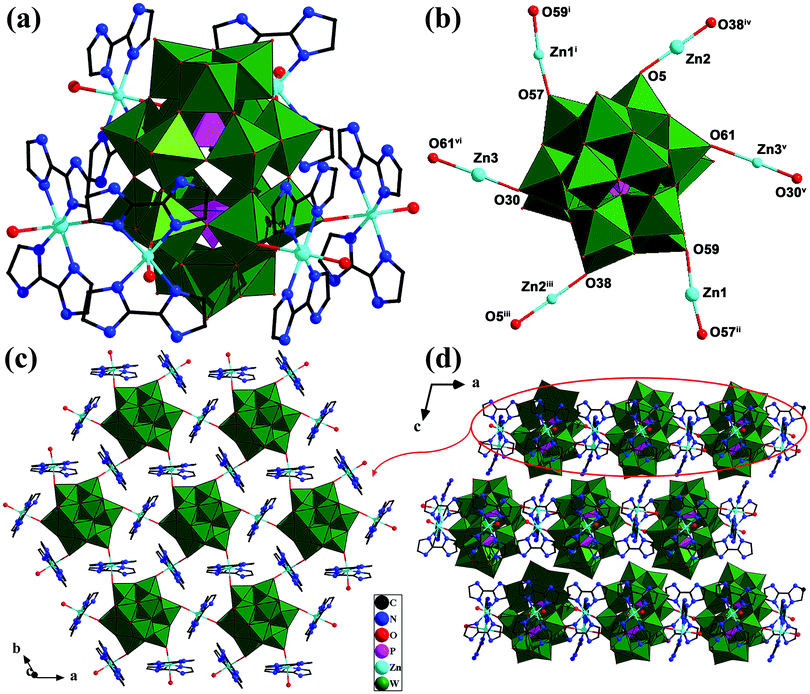 |
| Fig. 1 (a) Polyhedral and ball-and-stick representation of the basic structural unit of compound 1; (b) the connection mode between Zn centers and Dawson-type POM units in compound 1 [symmetry code: (i) x + 1, y + 1, z; (ii) x − 1, y − 1, z; (iii) x, y − 1, z; (iv) x, y + 1, z; (v) x − 1, y, z; (vi) x + 1, y, z]; (c) the 2-D network of compound 1; (d) the 3-D stacking mode of compound 1 (lattice H2O molecules, and hydrogen atoms have been omitted for clarity). | |
Compound 2 is composed of one {P2W18} polyoxoanion, six monoprotonated [H3biim]+ units, and eight lattice H2O molecules (Fig. S3, ESI†). Since this compound is easily dissolved in aqueous solution and cannot be used as a support, further characterization and research were not undertaken.
Physical characterization
FT-IR spectroscopy.
The IR spectrum of compound 1 is shown in Fig. S4a (ESI†). Its characteristic peaks are as follows: a peak at 1091 cm−1 is attributed to v(P–O), and strong peaks at 937, 804, 709, 666, 539 and 480 cm−1 are ascribed to the asymmetric stretching vibrations of the terminal v(W–Ot) and bridging v(W/Zn–Ob,c) bonds (Ot and Ob/Oc are terminal and bridging O atoms, respectively).19,20 A broad band at 3143–3632 cm−1 is associated with the v(O–H) of water molecules.21 Absorption peaks at 1633, 1527 and 1399 cm−1 are associated with the H2biim ligand.10,22
PXRD.
The powder XRD pattern of compound 1 and its simulated XRD pattern are shown in Fig. S5 (ESI†), the diffraction peaks of which correspond well. It was confirmed that the powder sample is of the same structure, i.e. compound 1, as the single crystals.
TGA-DTA.
As shown in Fig. S6 (ESI†), the TG curve of compound 1 shows three continuous weight loss steps from 33 to 800 °C. The first weight loss step (2.5%) from 33 to 200 °C is in accordance with the calculated value (2.0%), which is attributed to the loss of all lattice water molecules. The second and the third weight losses of 12.2% in the range of 330–750 °C are lower than the theoretical value (14.7%), which is caused by the influence of the loss of H2biim molecules. These results indicate that the structure of compound 1 has a good thermal stability up to 300 °C.
Factors affecting enzyme immobilization
For the immobilization of HRP onto compound 1, pH, HRP concentration and immobilization time, etc. can obviously affect the HRP loading. In order to obtain stable HRP/1 with high loading and activity, besides the above factors, the activity of the immobilized enzyme HRP/1 and the support itself on the selected model reaction, i.e., the colour-reaction of phenol with 4-AAP catalyzed by enzyme in the presence of H2O2 (Fig. S7, ESI†), should also be considered. Firstly, compound 1 and HRP/1 were used as catalysts in the model reaction, respectively, then UV-visible absorbance of the obtained chromogenic solution was tested. For comparison, the UV-visible spectrum of free HRP aqueous solution was also measured (Fig. 2a). As shown in Fig. 2a, there is a maximum absorbance peak at 510 nm for free HRP and HRP/1, while no signal was generated for compound 1, which means that compound 1 only acts as the enzyme-loading material without catalytic activity for the given model reaction.
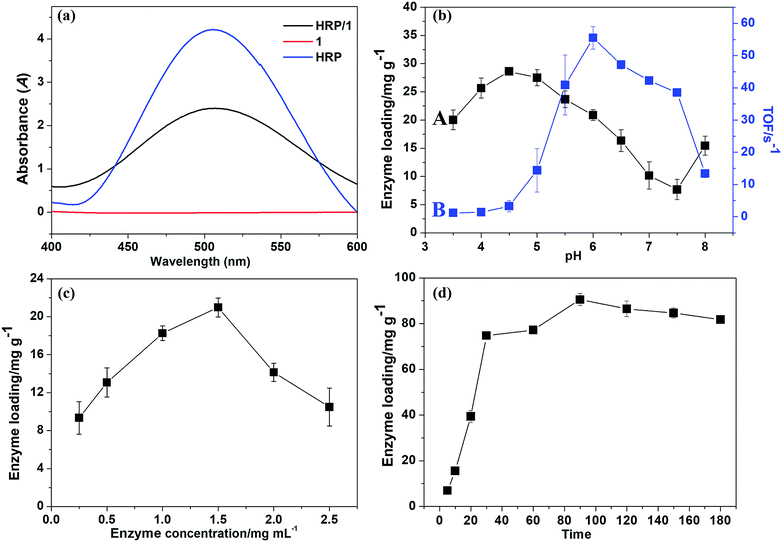 |
| Fig. 2 (a) The UV-vis absorbance spectra of the model reaction solutions with HRP, compound 1 and HRP/1 as catalysts; (b) the effect of pH on HRP loading (curve A) and catalytic activity (curve B) of HRP/1 evaluated by TOF (HRP solution: 0.5 mg mL−1 in PBS, compound 1: 5.0 mg, immobilization time: 30 min; the line is drawn only to guide the eye); (c) the influence of HRP concentration on enzyme loading for compound 1 as support (pH: 6.0, compound 1: 5.0 mg, immobilization time: 10 min); (d) the influence of immobilization time on enzyme loading for compound 1 as support (pH: 6.0, HRP concentration: 1.5 mg mL−1 in PBS, compound 1: 5.0 mg). | |
Because HRP has the maximum absorption at 403 nm, and its concentration has a linear relationship with the absorbance of HRP-containing solution, it forms a standard curve of HRP concentration (Fig. S8, ESI†). As shown in Fig. S8 (ESI†), the HRP concentration in the testing solution can be determined by colorimetry. Based on this, the immobilized amount of HRP (HRP load) can be calculated according to the following equation (eqn (1)):23
| Immobilized amount (mg g−1) = (c0 − c) V/Ws | (1) |
where,
c0 and
c (mg mL
−1) determined from Fig. S8 (ESI
†) are the initial and final HRP concentrations before and after HRP immobilization, respectively;
V (mL) is the volume of HRP solution; and
Ws (compound
1, 5 mg) represents the amount of support.
Through numerous tests, some main factors affecting the enzyme immobilization were found, and all data were averages of multiple measurements. The pH value is one of the most important factors affecting the enzyme immobilization and the activity of free/immobilized enzyme. The effects of pH on enzyme loading and the catalytic activity of immobilized enzyme were evaluated (see Fig. 2b). As shown in Fig. 2b, curve A, with the pH increasing from 3.5 to 4.5, the enzyme loading initially increased, and then it gradually decreased in the pH range of 4.5–7.5. While the activity of (evaluated by TOF values) HRP/1 obviously increased with increasing pH value from 3.5 to 6.0, it then decreased after pH 7.5, and the maximum TOF value was at pH 6.0 (Fig. 2b, curve B). The enzyme loading and the activity of immobilized enzyme should simultaneously be considered in confirmation of the optimal pH. So, it was determined that the optimal pH of HRP immobilization was 6.0. The influence of HRP concentration on HRP loading at pH 6.0 is displayed in Fig. 2c. It was found that the optimal HRP concentration is 1.5 mg mL−1. In addition, when the pH was 6.0 and HRP concentration was 1.5 mg mL−1, the immobilization time was also determined (Fig. 2d). As shown in Fig. 2d, the enzyme loading was maximum at 90 min.
Summarizing the above results, it is suggested that the optimum conditions of making HRP/1 in the lab are as follows: a loading pH of 6.0, HRP concentration of 1.5 mg mL−1, and immobilization time of 90 min. The maximum of HRP load of 90.1 mg g−1 can be calculated from eqn (1). This result indicates that compound 1 has relatively high loading capacity for HRP compared with other reported materials, such as nanoporous copper (8.8 mg g−1), modified chitosan (82.66 mg g−1) and graphene oxide (0.7 mg g−1).24–26
Characterization of the immobilized HRP
HRP can be directly adsorbed on compound 1 by simple physical adsorption, but the amount adsorbed is small, and it is difficult to determine the existence of HRP. In order to detect HRP in the obtained immobilized enzyme, a fluorescence labelling method was adopted.7,27 HRP was labelled with RhBTC before immobilization, then the RhBTC labelled HRP (RhBTC-HRP) was also subjected to the same immobilization procedure as free HRP, the immobilized labelling-enzyme RhBTC-HRP/1 was obtained and tested with confocal laser scanning microscopy (CLSM) (Fig. 3). It can be clearly observed from Fig. 3 that there are more luminescent red particles generated from RhBTC-HRP/1, which confirms that HRP has been successfully loaded on compound 1. To investigate whether the structure of the enzyme is still maintained after immobilization, CD spectroscopy was adopted for aqueous solution samples, and the CD spectra (Fig. S9, ESI†) of free HRP and eluting HRP removed from its support (compound 1) were compared. The results illustrated in Fig. S9 (ESI†) show that the curve profile of desorbed HRP solution coincides well with that of free HRP, indicating that the secondary structure of the enzyme is retained.
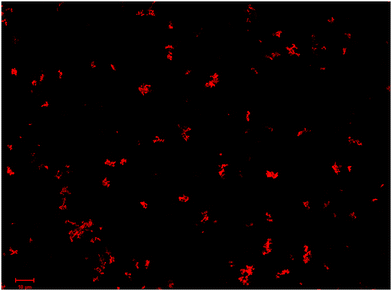 |
| Fig. 3 CLSM image of HRP/1. | |
The interactions between the HRP molecule and the POM support can be analyzed by DSC (S10, ESI†). As shown in Fig. S10a (ESI†), free HRP shows three endothermic peaks at about 72, 230 and 341 °C, respectively. The first endothermic peak and weight loss can be assigned to the dehydration of the enzyme. The endothermic peaks at about 230 and 341 °C can be attributed to the decomposition of the enzyme. As shown in Fig. S10b and c (ESI†), HRP/1 exhibits a weak endothermic peak at about 144 °C, which corresponds to the loss of crystal water of compound 1. The strong endothermic peak at about 499 °C can be assigned to the decomposition of POMs. Because of the wide and weak endothermic peaks in the range of 144–450 °C, the decomposition peak of the enzyme is not obvious in the DSC of HRP/1. However, the TGA curve of HRP/1 reveals a clear weight loss at 260 °C. This can be regarded as the decomposition temperature of the enzyme. The increased decomposition temperature of the enzyme in HRP/1 may be related to the supermolecular or electrostatic interactions of the enzyme and compound 1, which show that the enzyme can be stabilized. Therefore, we suggest that the enzymes were immobilized successfully in HRP/1.
Catalytic activity of the immobilized HRP and H2O2 detection
The model color-reaction of phenol with 4-AAP catalyzed by HRP in the presence of H2O2 can evaluate the activity of free HRP or HRP/1 (Fig. S7, ESI†). As shown in Fig. 2b, for curve B, within the range of pH 3.5–8.5, HRP/1 has catalytic activity and showed maximum activity at pH 6. In the catalytic oxidation reaction, it is obvious that H2O2 concentration can affect the intensity of the color-product, so a colorimetric system was developed for determination of H2O2 concentration.28,29 Here, the detection of H2O2 was investigated based on this method (Fig. 4). As can be seen from Fig. 4, the dose-dependent H2O2 enhancement shows a good linearity in the H2O2 concentration (c(H2O2)) range from 0.04 to 0.20 mmol L−1, which can be expressed as A = 2.123c (mmol L−1) − 0.035 with the correlation coefficient (R2) of 0.9959 (the inset) and the detection limit of H2O2 is 3.11 × 10−3 mmol L−1 (S/N = 3). Compared to the reported materials Au nanoparticles/POM/graphene (a linear response range of 5 × 10−3–18 mmol L−1) and FF@PW12@GO (the detection range of 1 × 10−3–7.5 × 10−2 mmol L−1),28,29 our HRP/1 detection system shows a better response for H2O2 concentration, indicating that the high catalytic activity of HRP remained after immobilization on compound 1, and HRP/1 can be used as a new catalyst material to detect trace amounts of H2O2 in solution.
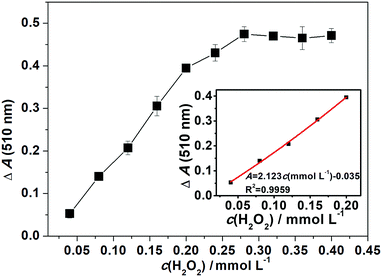 |
| Fig. 4 The linear calibration plot for H2O2 detection by the use of HRP/1 as catalyst. ΔA = A (the immobilized HRP, 510 nm) − A (blank, 510 nm). Reaction time is 5 min (inset: kinetics curve). | |
To investigate the reusability of the immobilized enzyme, the used solid catalyst HRP/1 was easily separated from the reaction system by centrifugation, washed with PBS and freeze-dried, and then reused in the next cycle of catalytic co-oxidation of phenol and 4-AAP. Under the HRP load of 90.1 mg g−1 at pH 6, as per the previous operation in the assay of the immobilized HRP activity, the reusability of HRP/1 in this model reaction was tested (Fig. S11, ESI†). As shown in Fig. S11 (ESI†), the catalytic activity of HRP/1 slightly decreases in the first three reaction cycles, and it is still above 50% after the seventh cycle. The TOF values fell from 75 to about 25 s−1 after 12 cycles, which illustrates that the catalytic activity of HRP/1 still remained. To further test the activity of HRP/1, the re-isolated HRP/1 after recycles was reused to test H2O2 concentration, for example, the result of the sample of six recycles is illustrated in Fig. S12 (ESI†). As shown in Fig. S12 (ESI†), the limit of detection has not changed much compared with that of Fig. 4, which proves that the activity of HRP/1 is stable. The decrease is probably due to leaching during repeated washing after a long time in contact with the high concentration of substrate.24 Furthermore, after nine cycles, the activity of HRP/1 remained almost unchanged. The above results show that compound 1 exhibits good capability for HRP loading, and the as-prepared HRP/1 retains high catalytic activity for the model reaction. In addition, the reusability of HRP/1 indicates that there is a certain force between the POM support and the enzyme. Based on the analysis of interactions between POM and enzymes or proteins in relevant references,7,30–33 together with the structural characteristics of compound 1, it can be speculated that the interactions between HRP and POM are mainly the H-bonding, electrostatic and van der Waals interactions, which are still not strong enough to maintain a long-time loading stability.
Conclusion
The highly negative-charged Dawson-type POM polyoxoanion and {Zn(II)-H2biim} complex cation moieties were assembled into a new crystalline organic–inorganic hybrid (compound 1) possessing a 2-D network with (6,3)-net topology under hydrothermal conditions. Compound 1 is a good enzyme support for immobilizing HRP with a high HRP load of 90.1 mg g−1. The as-prepared immobilized enzyme HRP/1 shows a high catalytic activity in the co-oxidation color-reaction of phenol and 4-AAP by H2O2 and good stability in twelve recycling experiments. In addition, HRP/1 can be used to detect trace amounts of H2O2 in solution with a detection limit of 3.11 × 10−3 mmol L−1. HRP/1 exhibits a new feasibility for the exploration of Dawson-type POM-based organic–inorganic hybrid materials as a new type of enzyme support.
Conflicts of interest
There are no conflicts to declare.
Acknowledgements
This work was financially supported by the National Natural Science Foundation of China (grant no. 21671036 and 21503103), Fundamental Research Funds for the Central Universities (grant no. 2412016KJ018), and the Opening Project of Key Laboratory of Polyoxometalate Science of Ministry of Education (grant no. 130028721).
References
- R. A. Sheldon and S. van Pelt, Chem. Soc. Rev., 2013, 42, 6223–6235 RSC.
- M. Hartmann and X. Kostrov, Chem. Soc. Rev., 2013, 42, 6277–6289 RSC.
- R. DiCosimo, J. McAuliffe, A. J. Poulose and G. Bohlmann, Chem. Soc. Rev., 2013, 42, 6437–6474 RSC.
- S. C. Sun, X. Liu, L. Yang, H. Q. Tan and E. B. Wang, Eur. J. Inorg. Chem., 2016, 4179–4184 CrossRef CAS.
- P. F. Gong, S. Y. Luo, L. Kang, X. X. Jiang, J. Xu, G. C. Zhang, Z. S. Lin, Y. C. Wu and C. T. Chen, Inorg. Chem., 2015, 46, 6066–6068 CrossRef PubMed.
- G. J. Cao, J. D. Liu, T. T. Zhuang, X. H. Cai and S. T. Zheng, Chem. Commun., 2015, 51, 2048–2051 RSC.
- J. Du, M. D. Cao, S. L. Feng, F. Su, X. J. Sang, L. C. Zhang, W. S. You, M. Yang and Z. M. Zhu, Chem. – Eur. J., 2017, 23, 14614–14622 CrossRef CAS PubMed.
- C. Molitor, A. Bijelic and A. Rompel, Chem. Commun., 2016, 52, 12286–12289 RSC.
- F. Boussema, A. J. Gross, F. Hmida, B. Ayed, H. Majdoub, S. Cosnier, A. Maaref and M. Holzinger, Biosens. Bioelectron., 2018, 109, 20–26 CrossRef CAS PubMed.
- C. Kirchner and B. Krebs, Inorg. Chem., 1987, 26, 3569–3576 CrossRef CAS.
- P. P. Zhang, J. Peng, H. J. Pang, J. Q. Sha, M. Zhu, D. D. Wang and M. G. Liu, CrystEngComm, 2011, 13, 3832–3841 RSC.
- Z. L. Li, Y. Wang, L. C. Zhang, J. P. Wang, W. S. You and Z. M. Zhu, Dalton Trans., 2014, 43, 5840–5846 RSC.
- R. Contant, W. G. Klemperer and O. Yaghi, Inorg. Synth., 1990, 27, 104–111 CAS.
- G. M. Sheldrick, Acta Crystallogr., 2015, C71, 3–8 CrossRef PubMed.
- L. Gao, Z. Chen and J. H. Zhu, New J. Chem., 2011, 35, 1867–1875 RSC.
-
L. A. Decker, Worthington Enzyme Manual, Worthington Biochemical Corp, New Jersey, 1977, pp. 43–45 Search PubMed.
- L. Y. Zhou, W. Tang, Y. J. Jiang, L. Ma, Y. He and J. Gao, RSC Adv., 2016, 6, 90061–90068 RSC.
- J. A. Nicell and H. Wright, Enzyme Microb. Technol., 1997, 21, 302–310 CrossRef CAS.
- L. Y. Fan, K. Yu, J. H. Lv, H. Zhang, Z. H. Su, L. Wang, C. M. Wang and B. B. Zhou, Dalton Trans., 2017, 46, 10355–10363 RSC.
- J. Q. Sha, J. Peng, Y. Q. Lan, Z. M. Su, H. J. Pang, A. X. Tian, P. P. Zhang and M. Zhu, Inorg. Chem., 2008, 47, 5145–5153 CrossRef CAS PubMed.
- K. Yu, Y. Yu, B. D. Jiang and B. B. Zhou, CrystEngComm, 2013, 15, 5156–5167 RSC.
- C. Y. Yang, L. C. Zhang, Z. J. Wang, L. Wang, X. H. Li and Z. M. Zhu, J. Solid State Chem., 2012, 194, 270–276 CrossRef CAS.
- C. Chao, J. D. Liu, J. T. Wang, Y. W. Zhang, B. Zhang, Y. T. Zhang, X. Xiang and R. F. Chen, ACS Appl. Mater. Interfaces, 2013, 5, 10559–10564 CrossRef CAS PubMed.
- H. J. Qiu, L. Lu, X. R. Huang, Z. H. Zhang and Y. B. Qu, Bioresour. Technol., 2010, 101, 9415–9420 CrossRef CAS PubMed.
- M. Monier, D. M. Ayad, Y. Wei and A. A. Sarhan, Int. J. Biol. Macromol., 2010, 46, 324–330 CrossRef CAS PubMed.
- J. L. Zhang, F. Zhang, H. J. Yang, X. L. Huang, H. Liu, J. Y. Zhang and S. W. Guo, Langmuir, 2010, 26, 6083–6085 CrossRef CAS PubMed.
- X. L. Wu, J. Ge, C. Yang, M. Hou and Z. Liu, Chem. Commun., 2015, 51, 13408–13411 RSC.
- R. J. Liu, S. W. Li, X. L. Yu, G. J. Zhang, S. J. Zhang, J. N. Yao, B. Keita, L. Nadjo and L. J. Zhi, Small, 2012, 8, 1398–1406 CrossRef CAS PubMed.
- Z. Ma, Y. F. Qiu, H. H. Yang, Y. M. Huang, J. J. Liu, Y. Lu, C. Zhang and P. A. Hu, ACS Appl. Mater. Interfaces, 2015, 7, 22036–22045 CrossRef CAS PubMed.
- A. Bijelic and A. Rompel, Coord. Chem. Rev., 2015, 299, 22–38 CrossRef CAS PubMed.
- G. Hungerford, F. Hussain, G. R. Patzke and M. Green, Phys. Chem. Chem. Phys., 2010, 12, 7266–7275 RSC.
- L. Zheng, Y. Ma, G. J. Zhang, J. N. Yao, B. S. Bassil, U. Kortz, B. Keita, P. de Oliveira, L. Nadjo, C. T. Craescu and S. Miron, Eur. J. Inorg. Chem., 2009, 5189–5193 CrossRef CAS.
- G. J. Zhang, B. Keita, J. C. Brochon, P. de Oliveira, L. Nadjo, C. T. Craescu and S. Miron, J. Phys. Chem. B, 2007, 111, 1809–1814 CrossRef CAS PubMed.
Footnote |
† Electronic supplementary information (ESI) available: ORTEP views of compounds 1 and 2; IR, XRPD, TG-DTA, catalytic results. CCDC 1441744 and 1851431. For ESI and crystallographic data in CIF or other electronic format see DOI: 10.1039/c8nj03776k |
|
This journal is © The Royal Society of Chemistry and the Centre National de la Recherche Scientifique 2019 |
Click here to see how this site uses Cookies. View our privacy policy here.