DOI:
10.1039/C8NJ03740J
(Paper)
New J. Chem., 2019,
43, 162-167
Graphite-like carbon nitride quantum dot (CNQD)-modified Bi2MoO6 heterostructure with high visible-light photocatalytic activity†
Received
27th July 2018
, Accepted 12th November 2018
First published on 13th November 2018
Abstract
We have achieved in situ growth of g-C3N4 quantum dots (CNQDs) on the surface of Bi2MoO6 nanosheets. Compared with pure Bi2MoO6, the CNQDs/Bi2MoO6 composite shows enhanced photocatalytic performance for the degradation of rhodamine B (Rh B) under visible light, and 5 wt% CNQDs/Bi2MoO6 nanosheet sample can reduce Rh B around 100%. The enhanced performance is due to the high specific surface area caused by CNQD loading and efficient separation of electron–hole pairs through the two-component composite system.
1. Introduction
Semiconductor photocatalysis has drawn great attention due to its wide application for hydrogen production from splitting water and degradation of environmental pollutants.1–5 The efficient absorption and utilization of solar energy for the degradation of organic pollutants is one of the most promising methods to address the environmental problems.6–9 Recent studies have shown that the oxidation of aurivillius clearly exhibits activity of photocatalytic decomposition of water and degradation of organic pollutants. However, it is still a challenge to improve the photocatalytic efficiency of Bi2MoO6 to meet the practical application requirements, which restricts the quantum yield caused by the rapid recombination of photoinduced electrons and holes.10–14
As is known, g-C3N4 is a metal-free visible-light photocatalyst, which was first reported by Wang et al., and it has a narrow band gap of 2.7 eV; g-C3N4 exhibits good water cracking and hydrogen production15–20 and degradation of organic pollutants under visible light.21–26 Although g-C3N4 exhibits good activity and easy preparation, the high recombination rates of the photogenerated electron–hole pairs and low surface area restrict its utilization of solar energy. In general, g-C3N4 is obtained using suitable precursors such as cyanamide, dicyandiamide, urea, thiourea, melamine and other thermal condensation materials.27–31 To better utilize the electronic structure and physical structure of g-C3N4, a variety of strategies are widely used to improve the photocatalytic properties, including non-metal or metal doping,32,33 coupling with semiconductor composites,34–36 and synthesizing mesoporous structures.37–40 Exploring g-C3N4 quantum dots (CNQDs) based materials with large surface area has great significance to further improve the quantum efficiency.
Herein, we report a successful attempt at the fabrication of g-C3N4 quantum dot (CNQDs)/Bi2MoO6 hierarchical nanostructures via a facile in situ precipitation method, and the photocatalytic activity of the nanostructures is investigated by measuring the degradation of rhodamine (Rh B) under visible light (λ > 420 nm). The experimental results indicate that the prepared CNQDs/Bi2MoO6 nanoheterostructure shows excellent photocatalytic performance. In addition, the possible photocatalysis mechanism of CNQDs/Bi2MoO6 under visible-light irradiation is also discussed in detail.
2. Experimental section
2.1. Preparation of photocatalysts
2.1.1. Preparation of CNQDs.
Bulk g-C3N4 was prepared by heating melamine for 4 h to 550 °C and it was kept at this temperature for another 4 h in air.41 The prepared bulk g-C3N4 was ground and then placed in a muffle furnace to maintain a temperature of 500 °C in a facility of 2 h to prepare g-C3N4 nanosheets. Then, 0.1 g of agglomerated g-C3N4 was sonicated in a mixed solution of concentrated sulfuric acid (H2SO4) (20 mL) and nitric acid (HNO3) (60 mL) for 16 h to obtain CNQDs, which were washed with 400 mL of deionized water to remove impurities and centrifuged. The sample was dispersed in anhydrous ethanol for use.
2.1.2. Preparation of Bi2MoO6 nanosheets.
All reagents for synthesis and analysis were commercially available and used without further treatment. The Bi2MoO6 nanosheets were synthesized through a facile hydrothermal method.42 Briefly, a solution of Bi(NO3)3 (0.40 mol L−1, 5 mL H2O) was stirred for 30 min. Then, (NH4)6Mo7O24 (0.20 mol L−1, 5 mL H2O) was added to the suspension, which was stirred magnetically for another 30 min. After carefully adjusting the pH value of 3 using 25 wt% NH3·H2O solution, the mixed solution was transferred into a 20 mL Teflon-lined steel autoclave, which was heated in an oven at 160 °C for 24 h. Then, the system was allowed to cool to room temperature naturally. At last, the obtained sample was collected and washed with ethanol and distilled water several times and dried at 70 °C for 2 h.
2.1.3. Preparation of CNQDs/Bi2MoO6 nanoheterostructure.
The prepared Bi2MoO6 nanosheets (60 mg) were mixed with 60 mL of ethanol by ultrasonication for 30 min. For loading CNQDs on Bi2MoO6, a certain amount (3, 4.5 and 6 mL) of CNQD ethanol solution (1 g L−1) was added and stirred for 24 h. The resulting suspension was washed with deionized water three times and dried at 60 °C for 12 h. The theoretical loading amounts of CNQDs were 5, 7.5 and 10 wt%.
2.2. Characterization of photocatalysts
The crystal structures of the samples were characterized by X-ray diffraction (XRD) on a Rigaku (Japan) D/max 2500 X-ray diffractometer (Cu Kα radiation, λ = 0.154 18 nm). The morphologies and structure details of the as-synthesized samples were detected using transmission electron microscopy (TEM, JEM-2100F). X-ray photoelectron spectroscopy (XPS) analysis was performed with an ESCALa-b220i-XL electron spectrometer (VG Scientific, England) using 300 W Al Kα radiation. The photoluminescence (PL) spectra of the photocatalysts were obtained by an F4500 (Hitachi, Japan) photoluminescence detector with an excitation wavelength of 325 nm. The UV-vis diffuse reflectance spectra (DRS) were recorded using a scan UV-vis spectrophotometer (UV-2550).
2.3. Photocatalytic activity studies
The photocatalytic properties of the as-prepared samples were evaluated using Rh B as a model compound. Rh B is a very stable compound, which has been used widely as a representative for examining the performance of numerous visible light-active catalysts. In experiments, the Rh B solution (0.001 mmol L−1, 100 mL) containing 0.02 g of photocatalyst was mixed in a pyrex reaction glass. A 300 W Xe lamp (λ > 420 nm) was employed to provide visible light irradiation. A 420 nm cut-off filter was inserted between the lamp and the sample to filter out UV light (λ < 420 nm). Prior to visible illumination, the suspension was strongly stirred in the dark for 30 min. Then, the solution was exposed to visible light irradiation under magnetic stirring. At given time intervals, 4 mL of the suspension was periodically collected and analyzed after centrifugation. The Rh B concentration was analyzed by a UV-2550 spectrometer to record the intensity of the maximum band at 552 nm in the UV-vis absorption spectra.
3. Results and discussion
Fig. 1a shows the XRD patterns of as-synthesized 5% CNQDs/Bi2MoO6, 7.5% CNQDs/Bi2MoO6, 10% CNQDs/Bi2MoO6 and Bi2MoO6. All the peaks in the XRD pattern of Bi2MoO6 can be readily indexed into specific crystal planes of the Bi2MoO6 phase (JCPDS No. 21-0102). It should be noted that no typical diffraction peaks of CNQDs appear in 5% CNQDs/Bi2MoO6, 7.5% CNQDs/Bi2MoO6 and 10% CNQDs/Bi2MoO6 composites. The reason can be low weight loading and small size of CNQDs on the surface of Bi2MoO6 nanosheets.43
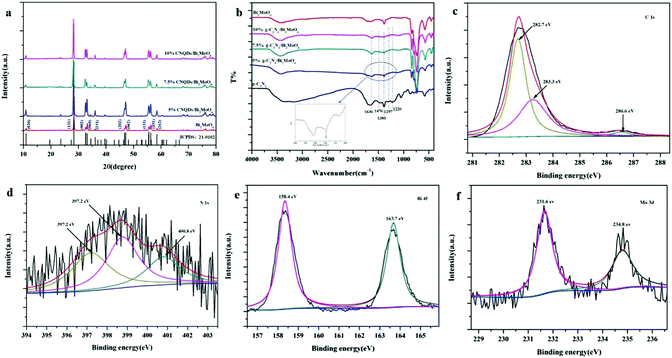 |
| Fig. 1 (a) XRD patterns of as-prepared samples, (b) FT-IR spectra of the as-synthesized samples. XPS spectra of the as-obtained CNQDs/Bi2MoO6 sample: (c) C 1s spectrum, (d) N 1s spectrum, (e) Bi 4f spectrum, (f) Mo 3d spectrum. | |
Here, the successful loading of CNQDs was verified by FT-IR. The FT-IR spectra of the as-prepared CNQDs, Bi2MoO6, 5% CNQDs/Bi2MoO6, 7.5% CNQDs/Bi2MoO6 and 10% CNQDs/Bi2MoO6 samples are displayed in Fig. 1b. The main characteristic peaks of CNQDs are observed in the spectra of 5% CNQDs/Bi2MoO6, 7.5% CNQDs/Bi2MoO6 and 10% CNQDs/Bi2MoO6, showing successful loading of CNQDs. As shown in Fig. 1b, the absorption band at 1636 cm−1 can be ascribed to the C–N heterocycle stretching vibration mode, whereas the strong peaks at 1476, 1380, 1297 and 1220 cm−1 can be assigned to aromatic C–N stretching vibration modes.43 Hence, these characteristic peaks prove the existence of CNQDs in the 5% CNQDs/Bi2MoO6, 7.5% CNQDs/Bi2MoO6 and 10% CNQDs/Bi2MoO6 samples.
XPS analysis was carried out to further analyze the surface chemical composition and chemical status of elements in the as-prepared CNQDs/Bi2MoO6 sample (Fig. 1c–f). The C 1s XPS spectrum (Fig. 1c) clearly displays three characteristic peaks located at 282.7, 283.3 and 288.1 eV; the peaks of 282.7 eV and 283.3 eV correspond to adventitious hydrocarbons from the XPS instrument itself and defect-containing sp2-hybridized carbon atoms present in graphitic domains, whereas the last one is assigned to C–N–C coordination.44 A broad peak at around 397.2 eV in the N 1s spectrum (Fig. 1d) is observed, which corresponds to C–N–C coordination.44 The Bi 4f fine XPS spectrum of the sample is shown in Fig. 1e. XPS signals of Bi 4f are observed at binding energies of about 163.7 eV (Bi 4f7/2) and 158.4 eV (Bi 4f5/2), which are ascribed to Bi3+.45 The characteristic spin-orbital splitting photoelectrons for Mo 3d (231.6 and 234.8 eV) indicate a six-valent oxidation state for Mo6+ (Fig. 1f).45
The SEM images of CNQDs/Bi2MoO6 are shown in Fig. 2. The as-prepared CNQDs/Bi2MoO6 is a composite of nanosheets with average sizes of about 100 nm and thickness of tens of nanometers. The formation of the CNQDs/Bi2MoO6 heterostructure was also confirmed by the elemental mapping of the as-prepared CNQDs/Bi2MoO6 sample (Fig. 2c–g). Maps of C, N, O, Bi and Mo exhibit the same shape and location, demonstrating the existence of CNQDs and Bi2MoO6 in the CNQDs/Bi2MoO6 composite. This gives strong evidence for the formation of a CNQDs/Bi2MoO6 heterostructure.
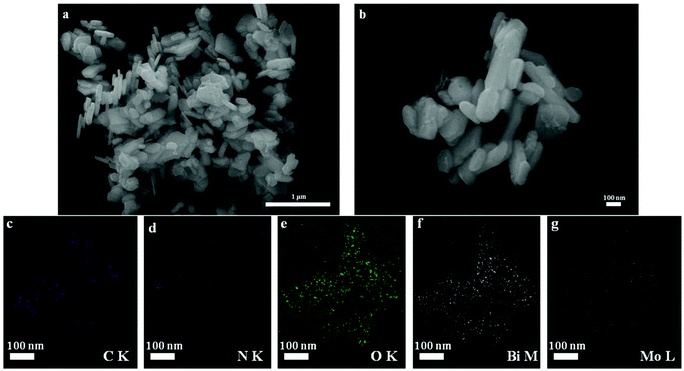 |
| Fig. 2 SEM images of as-synthesized CNQDs/Bi2MoO6 (a and b) and the corresponding EDS elemental mapping images (c–g) of CNQDs/Bi2MoO6 sample. The scale bars in figures are 100 nm. | |
For further investigating heterostructured CNQDs/Bi2MoO6 nanosheets, TEM characterization was performed. From Fig. 3a, the sheet-like morphology of the CNQDs/Bi2MoO6 sample can be clearly observed. The small size of CNQDs is beneficial for the improvement of the specific surface area of the composite photocatalyst (Fig. S3, ESI†).42 By measuring the lattice fringes, the resolved interplanar distances are about 0.314 and 0.336 nm (Fig. 3b and c), which correspond to the (131) plane of Bi2MoO6 and the (002) plane of CNQDs.42
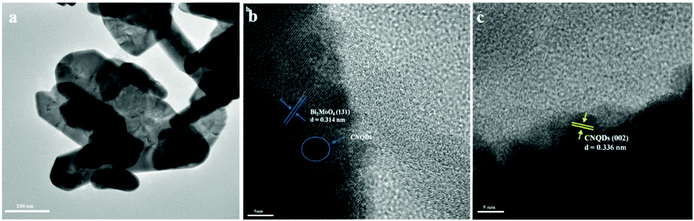 |
| Fig. 3 TEM image of CNQDs/Bi2MoO6 (a) and HRTEM images of CNQDs/Bi2MoO6 (b and c). | |
The absorbance properties of the as-prepared samples were measured using UV-vis diffuse reflectance spectroscopy (DRS). Fig. 4a displays UV-vis DRS of CNQDs, Bi2MoO6, 5% CNQDs/Bi2MoO6, 7.5% CNQDs/Bi2MoO6 and 10% CNQDs/Bi2MoO6. For pure CNQDs, the basal absorption edge occurs at a wavelength shorter than 450 nm, whereas the absorption edge of Bi2MoO6 rises rapidly at about 500 nm. According to the plot of αhν = A(hν − Eg)n/2, the band gaps (Eg) of Bi2MoO6 and CNQDs are estimated to be 2.47 and 2.73 eV, respectively (Fig. 4b).
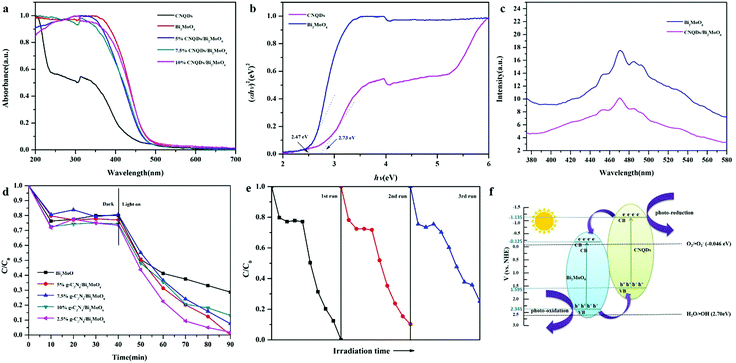 |
| Fig. 4 (a) UV-vis DRS of as-synthesized samples. (b) Plots of (αhν)2versus photon energy (hν) for the band gap energies of CNQDs and Bi2MoO6. (c) PL spectra of the as-prepared samples. (d) Photodegradation efficiencies of Rh B as a function of irradiation time for different photocatalysts. (e) Cycling runs for the photocatalytic degradation of Rh B over CNQDs/Bi2MoO6 sample under visible-light irradiation. (f) Photocatalytic mechanism scheme of CNQDs/Bi2MoO6 composite under visible-light irradiation (λ > 420 nm). | |
To understand the higher photocatalytic activity of the CNQDs/Bi2MoO6 composite relative to those of Bi2MoO6 and CNQDs, the PL spectra of photocatalysts excited at 325 nm were obtained (Fig. 4c). In comparison with the results for CNQDs, the intensities of the peaks for CNQDs/Bi2MoO6 were much lower, which showed that the binary composite samples have a lower recombination rate of photogenerated electron–hole pairs. The as-prepared CNQDs/Bi2MoO6 sample, in particular, revealed almost no emission peak, showing the lowest recombination rate for the heterostructure of CNQDs/Bi2MoO6 in blocking the recombination of photogenerated electrons and holes.
Fig. 4d displays the photocatalytic decolorization of Rh B over different photocatalysts. On the basis of a blank experiment, the self-photolysis of Rh B could be neglected. For a pure Bi2MoO6 nanosheet sample, the concentration of Rh B was only reduced by about 71.25% after 50 min of visible-light irradiation. Compared with pure Bi2MoO6, the composite of heterostructured CNQDs (2.5, 5, 7.5 and 10%)/Bi2MoO6 nanosheet sample exhibited remarkably enhanced photocatalytic activity, and the concentration of Rh B was reduced by around 98.12, 100, 92.41 and 86.87%. Through the visible photocatalytic degradation experiments of colorless organic pollutants, we found that CNQDs/Bi2MoO6 exhibited excellent visible photocatalytic degradation activity to colorless pollutants (Fig. S4, ESI†).
To evaluate the recyclability of the CNQDs/Bi2MoO6 nanosheet sample, additional experiments have been carried out to degrade Rh B under visible light cycled three times (Fig. 4e). The photocatalytic activity of CNQDs/Bi2MoO6 sample for Rh B degradation decreased from 100% to 75% after three cycling runs, which demonstrated that the CNQDs/Bi2MoO6 composite has high stability in the photocatalytic process under visible-light irradiation. The decline in photocatalytic degradation is explained by the difficulty in recovering photocatalytic materials due to the smaller size. In addition, there is no clear difference in SEM images (Fig. S1, ESI†) of the CNQDs/Bi2MoO6 photocatalyst after cycle measurement. The above result demonstrates that the CNQDs/Bi2MoO6 sample described in this paper has high stability in the photocatalytic process under visible-light irradiation.
To investigate the photocatalytic mechanism of the CNQDs/Bi2MoO6 photocatalyst, the effect of scavengers on the degradation of Rh B was tested in the photocatalytic oxidation process, as revealed in Fig. S2 (ESI†). The photocatalytic degradation of Rh B was significantly inhibited in the presence of superoxide radical scavenger BQ. According to the results, it can be concluded that active species, i.e., ˙O2− radicals were the main oxygen active species for the CNQDs/Bi2MoO6 photocatalyst in the Rh B solution under visible-light illumination.
Based on the results and discussion above, the heterostructure formed in the CNQDs/Bi2MoO6 composite played an important role in the efficient separation of photoinduced electron–hole pairs. The potentials of VB and CB of a semiconductor can be calculated according to the following empirical equations:
Here,
EVB is the valence band-edge potential,
ECB is the conduction band-edge potential,
X is the electronegativity of the semiconductor, which is the geometric mean of the electronegativity of the constituent atoms,
Ee is the energy of free electrons on the hydrogen scale (about 4.50 eV), and
Eg is the band gap energy of the semiconductor. Using
eqn (1) and (2), the
ECB potentials of CNQDs and Bi
2MoO
6 are determined to be −1.135 and −0.125 eV, respectively. The
EVB potentials of CNQDs and Bi
2MoO
6 are calculated to be 1.595 and 2.345 eV, respectively.
Under visible-light irradiation, both CNQDs and Bi2MoO6 absorb visible light and can be excited to produce h+ and e−. The electrons on CB of CNQDs can easily migrate to that of Bi2MoO6 because the CB potential of CNQDs is more negative than that of Bi2MoO6, and electron transfer in this process is faster than the electron–hole recombination between VB and CB of CNQDs. In addition, because VB of CNQDs is above the VB of Bi2MoO6, the holes in the VB of Bi2MoO6 can also flow effectively into the VB of CNQDs, and this process is also faster than the electron–hole recombination between VB and CB of Bi2MoO6. The heterostructure formed between CNQDs and Bi2MoO6 prevents electron and hole recombination, which is beneficial for the enhancement of photocatalytic activity. Furthermore, the holes in the VB of Bi2MoO6 with strong oxidation power can directly degrade Rh B. Additionally, the electrons in the CB of CNQDs with strong reduction power can be transferred to adsorbed O2 to form ˙O2−, which can further oxidize Rh B to water and carbon dioxide.46
4. Conclusions
In summary, heterostructure CNQDs/Bi2MoO6 nanosheet samples were successfully constructed via an easily accessible route. The resulting CNQDs/Bi2MoO6 nanoheterostructures exhibited considerable improvement in the photocatalytic activity for Rh B degradation. The experimental results showed that the close interfacial connections between CNQDs and Bi2MoO6, where photoinduced electrons and holes were efficiently separated in space, were beneficial for retarding the charge recombination and improving the photoactivity. Moreover, the CNQDs/Bi2MoO6 nanosheet sample displayed good recyclability under visible-light illumination.
Conflicts of interest
There are no conflicts to declare.
Acknowledgements
This work was supported by the National Natural Science Foundation of China (No. 51778268, 21407059) and the Open Subject of the State Key Laboratory of Rare Earth Resource Utilization (RERU2017011).
References
- L. Shi, L. Liang, F. Wang, J. Ma and J. Sun, Catal. Sci. Technol., 2014, 4, 3235 RSC.
- G. H. Liu, J. L. Xu and K. Y. Wang, Nano Energy, 2017, 41, 269 CrossRef CAS.
- R. Asahi, T. Morikawa, T. Ohwaki, K. Aoki and Y. Taga, Science, 2001, 293, 269 CrossRef CAS PubMed.
- I. K. Konstantinou and T. A. Albanis, Appl. Catal., B, 2004, 49, 1 CrossRef CAS.
- J. X. Xia, D. Jun, H. T. Li, H. M. Li and S. J. Guo, Appl. Catal., B, 2016, 181, 260 CrossRef CAS.
- Y. Jia, Y. Ma, J. Tang and W. Shi, Dalton Trans., 2018, 47, 5542 RSC.
- D. Chen, F. Huang, Y. B. Cheng and R. A. Caruso, Adv. Mater., 2009, 21, 2206 CrossRef CAS.
- H. M. Chen, C. K. Chen, M. L. Tseng, P. C. Wu, C. M. Chang, L. C. Cheng and D. P. Tsai, Small, 2013, 9, 2926 CrossRef CAS PubMed.
- K. Y. Cheung, C. T. Yip, A. B. Djurišić, Y. H. Leung and W. K. Chan, Adv. Funct. Mater., 2007, 17, 555 CrossRef CAS.
- Y. S. Xu and W. D. Zhang, Dalton Trans., 2013, 42, 1094 RSC.
- L. Zhu, W. D. Zhang, C. H. Chen, B. Xu and M. F. Hou, J. Nanosci. Nanotechnol., 2011, 11, 4948 CrossRef CAS PubMed.
- J. Zhao, Y. Yang, W. S. Yu, Q. L. Ma, X. T. Dong, X. L. Wang, J. X. Wang and G. X. Liu, J. Mater. Sci.: Mater. Electron., 2017, 28, 543 CrossRef CAS.
- W. Yin, W. Wang and S. Sun, Catal. Commun., 2010, 11, 647 CrossRef CAS.
- G. Tian, Y. Chen, W. Zhou, K. Pan, Y. Dong, C. Tian and H. Fu, J. Mater. Chem., 2011, 21, 887 RSC.
- L. Shi, L. Liang, F. Wang, J. Ma and J. Sun, Catal. Sci. Technol., 2014, 4, 3235 RSC.
- A. Suryawanshi, P. Dhanasekaran, D. Mhamane, S. Kelkar, S. Patil, N. Gupta and S. Ogale, Int. J. Hydrogen Energy, 2012, 37, 9584 CrossRef CAS.
- H. W. Kang, S. N. Lim, D. Song and S. B. Park, Int. J. Hydrogen Energy, 2012, 37, 11602 CrossRef CAS.
- S. Martha, A. Nashim and K. M. Parida, J. Mater. Chem. A, 2013, 1, 7816 RSC.
- H. Yan, Chem. Commun., 2012, 48, 3430 RSC.
- Y. Wang, X. Wang and M. Antonietti, Angew. Chem., Int. Ed., 2012, 51, 68 CrossRef CAS PubMed.
- L. Shi, L. Liang, J. Ma, F. Wang and J. Sun, Catal. Sci. Technol., 2014, 4, 758 RSC.
- Y. Yang, Y. Guo, F. Liu, X. Yuan, Y. Guo, S. Zhang and M. Huo, Appl. Catal., B, 2013, 142, 828 CrossRef.
- C. Pan, J. Xu, Y. Wang, D. Li and Y. Zhu, Adv. Funct. Mater., 2012, 22, 1518 CrossRef CAS.
- S. C. Yan, Z. S. Li and Z. G. Zou, Langmuir, 2010, 26, 3894 CrossRef CAS PubMed.
- S. Ye, L. G. Qiu, Y. P. Yuan, Y. J. Zhu, J. Xia and J. F. Zhu, J. Mater. Chem. A, 2013, 1, 3008 RSC.
- J. Fu, Y. Tian, B. Chang, F. Xi and X. Dong, J. Mater. Chem., 2012, 22, 21159 RSC.
- X. Wang, K. Maeda, A. Thomas, K. Takanabe, G. Xin, J. M. Carlesson and M. Antonietti, Nat. Mater., 2009, 8, 76 CrossRef CAS PubMed.
- P. Niu, G. Liu and H. M. Cheng, J. Phys. Chem. C, 2012, 116, 11013 CrossRef CAS.
- F. Dong, L. Wu, Y. Sun, M. Fu, Z. Wu and S. C. Lee, J. Mater. Chem., 2011, 21, 15171 RSC.
- G. Zhang, J. Zhang, M. Zhang and X. Wang, J. Mater. Chem., 2012, 22, 8083 RSC.
- S. C. Yan, Z. S. Li and Z. G. Zou, Langmuir, 2009, 25, 10397 CrossRef CAS PubMed.
- G. Zhang, M. Zhang, X. Ye, X. Qiu, S. Lin and X. Wang, Adv. Mater., 2014, 26, 805 CrossRef CAS PubMed.
- L. Ge, C. Han, J. Liu and Y. Li, Appl. Catal., A, 2011, 409, 215 CrossRef.
- S. Kumar, T. Surendar, A. Baruah and V. Shanker, J. Mater. Chem. A, 2013, 1, 5333 RSC.
- X. Zhou, B. Jin, L. Li, F. Peng, H. Wang, H. Yu and Y. Fang, J. Mater. Chem., 2012, 22, 17900 RSC.
- J. X. Sun, Y. P. Yuan, L. G. Qiu, X. Jiang, A. J. Xie, Y. H. Shen and J. F. Zhu, Dalton Trans., 2012, 41, 6756 RSC.
- S. C. Lee, H. O. Lintang and L. Yuliati, Chem. – Asian J., 2012, 7, 2139 CrossRef CAS PubMed.
- Y. Cui, J. Huang, X. Fu and X. Wang, Catal. Sci. Technol., 2012, 2, 1396 RSC.
- J. Hong, X. Xia, Y. Wang and R. Xu, J. Mater. Chem., 2012, 22, 15006 RSC.
- Y. Zhang, J. Liu, G. Wu and W. Chen, Nanoscale, 2012, 4, 5300 RSC.
- W. Wang, C. Y. Jimmy, Z. Shen, D. K. Chan and T. Gu, Chem. Commun., 2014, 50, 10148 RSC.
- X. Lin, D. Xu, R. Zhao, Y. Xi, L. N. Zhao, M. Song and L. M. Chang, Sep. Purif. Technol., 2017, 178, 163 CrossRef CAS.
- X. Chen, Y. Zhou, Q. Liu, Z. Li, J. Liu and Z. Zou, ACS Appl. Mater. Interfaces, 2012, 4, 3372 CrossRef CAS PubMed.
- H. Li, J. Liu, W. Hou, N. Du, R. Zhang and X. Tao, Appl. Catal., B, 2014, 160, 89 CrossRef.
- Y. S. Xu and W. D. Zhang, Dalton Trans., 2013, 42, 1094 RSC.
- Y. Bao and K. Chen, Nano-Micro Lett., 2016, 8, 182 CrossRef PubMed.
Footnote |
† Electronic supplementary information (ESI) available. See DOI: 10.1039/c8nj03740j |
|
This journal is © The Royal Society of Chemistry and the Centre National de la Recherche Scientifique 2019 |