DOI:
10.1039/C8NJ03350A
(Paper)
New J. Chem., 2019,
43, 217-229
Fabrication of curcumin-loaded folic acid-tagged metal organic framework for triple negative breast cancer therapy in in vitro and in vivo systems†
Received
5th July 2018
, Accepted 2nd November 2018
First published on 8th November 2018
Abstract
Curcumin has shown therapeutic activity against triple-negative breast cancer (TNBC) cells, but it shows low efficacy and low bioavailability when administered as a free drug. We have developed a general synthesis strategy to encapsulate the natural molecule curcumin in monodispersed isoreticular nanoscale metal organic framework (NMOF-3) nanoparticles for targeted drug delivery in triple-negative breast cancer cells (TNBC). The cytotoxicity of IRMOF-3, curcumin-loaded IRMOF-3 (IRMOF-3@CCM) and folic acid-conjugated curcumin-loaded IRMOF-3 (IRMOF-3@CCM@FA) in human and mice TNBC were tested. IRMOF3@CCM@FA induces apoptosis by upregulating of Bax and downregulating of Bcl-2, and it further upregulates JNK and p53 in human TNBC cells. Simultaneously, we studied their effects in mice bearing TNBC induced by 4T1 cells. In in vivo studies, mice receiving targeted delivery of curcumin had increased survivability and reduced tumor volume compared with those observed for mice receiving non-targeted delivery. Our results highlight that folate-conjugated curcumin-loaded IRMOF-3 may be a useful therapeutic agent to treat TNBC.
Introduction
The most commonly diagnosed cancer is breast cancer, which leads to deaths among females worldwide.1 Among the different subtypes of breast cancer, TNBC refers to the most aggressive breast cancer phenotype where ER, PR and HER2 are negative.2 Most common treatments, such as surgery, radiation and chemotherapy, are not sufficient for metastatic TNBC treatment.3,4 Therefore, the development of novel effective therapeutic approaches against TNBC is urgently needed. Recent findings have shown that natural products have a huge effect in cancer therapy. Most of the anticancer agents used in clinical treatments are either natural or derived from natural products of various sources, such as microorganisms and plants.5 Among them, curcumin, which is derived from the plant Curcuma longa, plays a great role in different types of diseases, including cancer, auto-immune diseases, arthritis, atherosclerosis and diabetes.6,7 This compound is capable of inducing apoptosis pathway in pre-cancerous or cancer cells without affecting normal cells.8 Previous research has demonstrated that retinoic acid-mediated apoptosis in retinoic acid-resistant breast cancer cells is induced by curcumin.9 Regardless of its benefits in cancer therapy, it has poor solubility in aqueous solvent, which limits its wide application in cancer treatment. Therefore, novel strategies for delivery of curcumin into TNBC cells are highly desired. To date, several delivery systems based on nanoscale organic (liposome, polymer micelles and protein nanoparticles) and inorganic materials (Fe3O4, mesoporous silica, silver, gold) have been widely reported. Among them, some possess unique advantages due to their special structures, stable chemico-physical properties and low toxicities.
Recently, nanoscale metal organic frameworks have attracted great research interest for their potential applications in gas adsorption, sensing/biosensing, catalysis as well as biomedical uses.10–12 However, NMOFs have also been considered effective carriers for chemotherapeutic agent delivery.13,14 IRMOF-3, a class of NMOFs that belongs to inorganic–organic hybrid drug delivery systems (DDS), have attracted considerable attention due to their attractive features, such as large surface areas, ultra-high tunable functionality and porosity.15 These IRMOF-3 materials can be used for the delivery of curcumin in TNBC cells. In our experiment, a three dimensional IRMOF-3 was used to encapsulate the small molecule curcumin for intracellular delivery and subsequent release. The folate receptor over-expression in cancer cells acted as a new biomarker for drug delivery.16 As the folate receptor was over-expressed in TNBC cells, we attached folic acid on the surface of IRMOF-3. Folic acid-conjugated IRMOF-3 chains can increase the targeting capacity of nanocarriers due to their high affinity to its receptor, which are over expressed in TNBC, while the affinity is restricted in case of normal cells. Our DDS system possessed diverse properties, such as high biocompatibility, efficient cellular uptake, and therapeutic molecule release at the targeted site with stimuli responsive behaviors. We evaluated the anti-cancerous activity of IRMOF-3, IRMOF-3@CCM and IRMOF-3@CCM@FA in human and mice TNBC cell lines, namely, MDA-MB 468 and 4T1, respectively. We observed that the cost effective FA-conjugated IRMOF-3@CCM was more active in both human and mice TNBC cells compared with that in the IRMOF-3 or IRMOF-3@CCM. Next, we evaluated the antitumor activity of IRMOF-3, IRMOF-3@CCM and IRMOF-3@CCM@FA in vivo in BALB/c mice bearing TNBC induced by 4T1 cells. From this study, it was observed that curcumin was successfully delivered in both in vitro and in vivo systems. Therefore, targeted delivery of curcumin may be used as a therapeutic agent for TNBC therapy.
Materials and methods
Materials
In this study, all chemicals of analytical grade were used. Zinc nitrate hexahydrate (Zn(NO3)2·6H2O, 99%) and folic acid (FA) were purchased from Sigma Aldrich, US. Absolute ethanol, methanol, and N,N-dimethyl formamide (DMF) were purchased from Merck, Germany. Polyvinyl pyrrolidone (PVP), 2-amino terepthalic acid (NH2-H2BDC, 99%), and curcumin (CCM) were obtained from TCI fine chemicals. Ethanol, methanol, and N,N-dimethyl formamide (DMF) were purified by usual procedures. JC-1(mitochondrial staining dye), protease and phosphatase inhibitors and Alexa Fluor® 488 Annexin V/Dead Cell Apoptosis Kit were procured from Invitrogen-Life Technologies. N-Hydroxysuccinimide(NHS), 1-[3-dimethylamino)propyl]-3-ethylcarbodiimide hydrochloride (EDC), thiazolyl blue formazan (MTT), 2′,7′-dichlorofluorescein diacetate (DCF-DA), 4′,6-diamidino-2-phenylindole, and dihydrochloride(DAPI) were purchased from Sigma-Aldrich. Propidium iodide (PI) and ribonuclease were purchased from Himedia. Primary antibodies, namely, Bcl-2, Bax, p53, phospho Bad, JNK, and GAPDH were purchased from Cell Signalling, US. Anti-Notch-1 antibody was purchased from Abcam, US. Anti-β actin mouse monoclonal antibody was purchased from Santa-Cruz Biotechnology, US. Deionised (Millipore) water with resistivity of at least 18 MΩ was used throughout the experiments.
One step fabrication of folic acid-conjugated curcumin-loaded nanoscale IRMOF-3 (IRMOF-3@CCM@FA)
Folic acid-conjugated curcumin-loaded nanoscale IRMOF-3 was synthesized in a one step process. In brief, 15 mg of folic acid and 50 mg of curcumin were dissolved in 10 mL of ethanol and DMF (50
:
50) mixture. Then, 100 g PVP was dissolved into a solvent mixture containing 5 mL absolute ethanol and 5 mL pure DMF. Next, 133.86 mg Zn(NO3)2 and 16.29 mg NH2-H2BDC dissolved in 4 mL DMF were slowly added into the above solution. Following this, the resulting solution was treated under ultra-sonication for 10 min. Subsequently, the final solution was transferred into an autoclave and heated at 100 °C for 8 h. The final product was collected by centrifugation at 8000 rpm for 15 min. The product was again dispersed in 20 mL DMF, heated at 80 °C and dialyzed against distilled water (MWCO 12 K Da) for 12 h to remove unbound organic ligands and unreacted PVP. Subsequently, the mixture solution was added dropwise to the reaction system during the formation of nanoscale IRMOF-3. The obtained IRMOF-3@CCM@FA was separated by centrifugation, followed by washing with distilled water and drying at 50 °C in a vacuum oven for 12 h.
Synthesis of curcumin-loaded nanoscale IRMOF-3 (IRMOF-3@CCM)
Curcumin-loaded nanoscale IRMOF-3 (IRMOF-3@CCM) was synthesized by the following procedure. Briefly, 50 mg of curcumin was dissolved in 5 mL of dry ethanol and added to the reaction system very slowly during the formation of nanoscale IRMOF-3. IRMOF-3@CCM was separated by centrifugation and washed with distilled water three times to eliminate the unbound CCM. Finally, the product was dried in a vacuum oven at 50 °C for 12 h.
Synthesis of nanoscale IRMOF-3 and IRMOF-3@FA
The nanoscale metal organic framework was synthesized according to our previously reported method with slight modification.17 Briefly, 0.20 g of PVP was dissolved in a solvent mixture of 5 mL absolute ethanol and 5 mL pure DMF. Then, 66.93 mg Zn(NO3)2 and 16.29 mg NH2-H2BDC dissolved in 4 mL DMF were slowly added into the above solution. Then, the solution was treated under ultrasonication for 10 min. Subsequently, the final solution was transferred into a Teflon-lined stainless steel autoclave and heated at 100 °C for 8 h (heating rate: 1.5 °C min−1). The final product was collected by centrifugation at 8000 rpm for 15 min. The precipitate was again dispersed in 20 mL DMF, heated at 80 °C and dialyzed against distilled water for 12 h to remove the unreacted product. Finally, the product was dried for 12 h in a vacuum oven at 60 °C.
Folic acid-encapsulated IRMOF-3 (IRMOF-3@FA) was also synthesized for comparison study by the following procedure. First, folic acid (15 mg) was solubilized in dry DMF (5 mL) and added to the reaction system very slowly during the formation of IRMOF-3. Folic acid was conjugated with IRMOF-3 by physical encapsulation. For the elimination of unbound folic acid, the product was dialysed in DMF/water mixture. Finally, the product was dried at 50 °C for 12 h.
Characterizations
The presence of surface functional groups on the IRMOFs system were examined on a Agilent Cary 660 instrument by Fourier transform infrared spectroscopy (FTIR) at 25 °C using ATR method from 400 cm−1 to 4000 cm−1. The morphology, EDAX, and elemental mapping of the as-synthesized IRMOFs were investigated using a Field emission scanning electron microscope (FESEM, Supra 55 (ZEISS)). IRMOF-3, IRMOF-3@CCM, IRMOF3@FA, and IRMOF-3@CCM@FA were dispersed in Millipore water to form 0.5 mg mL−1 diluted suspensions using a sonicator for 30 min. When the particles completely dispersed in water, their size distributions were analyzed using a Brookhaven 90 plus particle size analyzer (DLS). For TEM measurements, one drop each of IRMOF-3 and IRMOF-3@CCM@FA suspensions was deposited onto separate carbon-coated copper grids, followed by drawing off the excess liquid with a filter paper. The grids were dried at room temperature before being examined under TEM.
Cell cultures
Human and Mice TNBC cell lines, namely, MDA-MB-468 and 4T1, respectively, were obtained from National Center for Cell Science (NCCS), Pune, India. Cells were grown in respective medium with 10% FBS (fatal bovine serum), penicillin/streptomycin (100 units per mL) at 37 °C and 5% CO2. All experiments were performed with the LD50 dose, unless otherwise mentioned. All treatments were performed at 37 °C and at a cell density to allow exponential growth.
Cell viability assay
The inhibition of cell growth was measured by MTT assay. In brief, the human and mice TNBC cell lines, namely, MDA-MB468 and 4T1, respectively, were seeded in 96 well plates at 1 × 104 cells per well and exposed to IRMOF-3@CCM@FA, IRMOF-3@CCM, and IRMOF-3 at different concentrations (0 μM, 20 μM, 40 μM, 60 μM, 80 μM, 100 μM, and 120 μM) for 24 h. After incubation, the cells were washed thrice with PBS and incubated with MTT solution (450 μg mL−1) for 3–4 h at 37 °C. The resulting formazan crystals were dissolved in the MTT solubilization buffer and absorbance values were measured at 570 nm using a microplate reader (Biotek, USA). Each analysis point was assessed in triplicate.
Drug release study
In vitro CCM release from the core of IRMOF-3 was examined to measure the amount of impregnated CCM that was discharged at predetermined time intervals.18 The cumulative drug release study was performed in physiological pH condition (pH ∼7.4) and lysosomal pH condition (pH ∼5.5) at 37 °C to evaluate the pH responsiveness of the as-synthesized IRMOF-3. First, 10 mg of IRMOF-3@CCM@FA was dispersed in pH ∼7.4 (physiological pH media), followed by incubation with shaking. After a predetermined interval, supernatant (2.5 mL) was taken out and exactly equal volume (2.5 mL) of PBS (pH ∼7.4) was added to maintain the same volume. A similar experiment was conducted at pH ∼5.5 (lysosomal pH medium). The percentage (%) of CCM released at different time intervals was calculated by measuring absorbance of supernatant solution at 421 nm from a standard curve of CCM.
ROS estimation
ROS were estimated by using DCF-DA dye. DCF-DA dye enters the cell and reacts with reactive oxygen to give a green fluorescent compound, dichlorofluorescein (DCF).19 The estimation of ROS was performed using both fluorometry and microscopy. Briefly, a stock solution of DCF-DA (10 mM) was made in methanol. It was further diluted with culture medium to a working concentration of 100 μM. Cells were treated with LD50 doses of IRMOF-3@CCM@FA, IRMOF-3@CCM, and IRMOF-3 for 12 h. Then, the cells were washed with ice cold Hanks balanced salt solution (HBSS) and incubated with 100 μM (working solution) of DCF-DA for 30 min at 37 °C. Then, the cells were lysed with alkaline solutions, and fluorescence intensity was measured by a fluorometer (Biotek).
Mitochondrial membrane potential measurement
JC-1 dye is used for the measurement of mitochondrial depolarization. It is a cationic dye that exhibits potential-dependent accumulation in mitochondria, indicated by a fluorescence emission shift from red to green as the mitochondrial gets damaged and loses its membrane.19 Cells were treated with the respective LD50 dose of IRMOF-3@CCM@FA, IRMOF-3@CCM, and IRMOF for 12 h. Then, the cells were washed with phosphate buffered saline (PBS) and incubated with (10 μg mL−1) JC-1 dye for 30 min at 37 °C. The cells were then observed under a fluorescent microscope (Leica, Wetzlar, Germany).
Nuclear morphology study
Nuclear morphology was studied by DAPI staining. After exposure to IRMOF-3@CCM@FA, IRMOF-3@CCM, and IRMOF-3 at their respective LD50 doses for 12 h, the cells were washed three times with 1× PBS and stained with 4′,6-diamidino-2-phenylindole (DAPI) in vectashield (0.2 g mL−1, Vector Laboratories Inc.). Nuclear morphology was observed under a fluorescence microscope.
Cell cycle analysis
Propidium iodide stain was used for the measurement of cell cycle in IRMOF-3@CCM@FA-, IRMOF-3@CCM-, and IRMOF-3-treated cells.20 After treatment for the indicated time, the cells were harvested and fixed with 70% ethanol overnight at 4 °C. Next day, the cells were washed with 1× PBS. Prior to staining with 50 μg mL−1 propidium iodide (PI, Sigma-Aldrich, St. Louis, MO), the cells were incubated for 1 h with 100 μg mL−1 of DNase free RNase A (SRL, India) at 37 °C. The cell cycles of the treated cells were analyzed using an FACS instrument (BD Biosciences, FACS Aria, San Jose, CA).
Annexin V-FITC staining
Apoptotic and necrotic cells were measured by annexinV-FITC staining. After treatment, the cells were washed in ice-cold PBS and incubated with 100 μL of annexin binding buffer. Annexin V-FITC (2 μg mL−1) was added and kept in dark at room temperature for 15 min. Then, 400 μL of annexin binding buffer was added. Prior to FACS analysis, 5 mL (0.5 mg mL−1) PI was added.
Western blot analysis
We studied the expression of Notch1 and apoptotic protein by Western blot. After treatment, the whole cell lysate was extracted with a lysis buffer containing 1% Triton X-100, 50 mM NaCl, 50 mM NaF, 20 mM Tris (pH 7.4), 1 mM EDTA, 1 mM EGTA, 1 mM sodium vanadate, 0.2 mM phenylmethanesulfonyl fluoride (PMSF), 0.5% NP-40 and protease inhibitors. Proteins were analyzed by immunoblotting after SDS-PAGE and visualized by ECL according to the manufacturer's instructions.
Reverse transcription and quantitative real-time polymerase chain reaction (qPCR)
The expression of Bcl-2 and Bax mRNA in control and IR-MOF3-, IR-MOF3@CCM-, and IR-MOF3@CCM@FA-treated cells was checked by qPCR. Total RNA was isolated using Trizol reagent according to manufacturer's guidelines (Invitrogen, USA). First strand cDNA was synthesized from mRNA using a PrimeScript® Reverse Transcriptase. The primer sequences were as follows: Bcl-2 (forward-5′-CTG AAT CGG AGA TGG AGA CC-3′, reverse-5′-TGG GAT GTC AGG TCA CTG AA-3′), BAX (forward-5′-GCG AGT GTC TCA AGC GCA TC-3′; reverse-5′-CCA GTT GAA GTT GCC GTC AGA A-3′) and β actin (forward-5′-TGC CGA CAG GAT GCA GAA G-3′, reverse-5′-GCC GAT CCA CAC GGA GTA CT-3′). PCR conditions of 95 °C for 30 s followed by 40 cycles at 95 °C for 5 s, and 60 °C for 34 s were used. β-actin was used as a control gene to normalize the expression of apoptotic genes. Comparative threshold cycle (Ct) method was used for the quantification of Bax and Bcl-2 genes. ΔCt = Ct (apoptotic genes) − Ct (β-actin) was used for the normalization of Bax and Bcl-2 genes with control. The ΔCt values were used to plot the expression of apoptotic genes using the formula 2−ΔΔCt.
In vivo study
6–7 weeks old BALB/c mice, weighing about 25–30 g, were obtained from the Center for Translational Animal Research (CTAR), Bose Institute, Kolkata, India, and were maintained as per the guidelines of animal ethical committee in accordance with CPCSEA guidelines. (IAEC Approval No. IAE/B1/60/2017).
Tumor development in vivo model
A 1 mL syringe without needle was used to draw 600 μL (enough for five mice) of cell suspension from a micro-centrifuge tube with 4T1 cell suspension. A 25-gauge needle was then placed onto the syringe and air was carefully but thoroughly removed. Then, 100 μL of cell suspension was injected subcutaneously (bevel side up) into mammary fat pad number four of eight week old female BALB/c mice. To aid the correct placement of the injection, the fat pad was identified and marked, and the surrounding area was shaved a day before implantation. Since the mice were injected without sedation, a second person was required to properly restrain the mice. The mice were grasped behind the neck firmly using the thumb and index finger; its body stretched along the hand, and its tail and feet were restrained using the last two fingers. The mice were held such that the skin of the abdomen taut was pulled to allow easier implantation of cells. All attempts were made to minimize mice distress, which was assessed by weekly weight measurements and the presence of physiological signs of distress (piloerection, vocalizations, recumbent posture or changes in eating habits).
Experimental design for in vivo study
An initial concentration profile was established with IRMOF-3 NPs and curcumin-encapsulated IRMOF-3 NPs (non-targeted) on separate groups of tumor-induced mice. Mice treated with IRMOF-3@CCM at a concentration of 2000 μg kg−1 showed a decrease in the tumor volume as well as an increase in survivability (days) compared with that of the tumor-induced control mice. Next, the mice were divided into four groups including untreated group, with six mice in each group:
Group 1, Tumor-induced mice (untreated).
Group 2, IRMOF-3 treated with 2000 μg kg−1 of BW.
Group 3, IRMOF-3@CCM treated with 2000 μg kg−1 of BW.
Group 4, IRMOF-3@CCM@FA treated with 2000 μg kg−1 of BW.
Measurement of the tumor volume & mice weight
Tumor volume, V (mm3), was calculated from the measured length (L), width (W), and height (H) of the tumor. It was assumed that the tumors were approximately hemi-ellipsoids whose volume was ½L·W·H. After five day intervals, tumor volume was calculated. Body weight of mice was also measured at end of the experiment.
In vivo tissue study
Tissue samples were fixed in 10% formalin, and then embedded in paraffin. Hematoxylin–eosin (H/E) in 5 μm thick paraffin was used for histological observation. Sections were examined under a microscope (Leica, Wetzlar, Germany).
Estimation of toxicity in vivo
The blood samples obtained were stored at room temperature for 2 h. Next, serum was collected after centrifugation at 840 g for 15 min. Serum SGOT, SGPT, ALP, creatinine and urea were measured with corresponding kits (Arkray-Healthcare-Pvt.-Ltd) according to the manufacturer's instructions.
Result and discussion
FESEM, TEM, DLS and elemental mapping analysis
The surface morphology and size of IRMOF-3, IRMOF-3@FA@CCM, IRMOF-3@CCM and IRMOF-3@FA were investigated using FESEM, as shown in Fig. 1. The as-synthesized IRMOF-3 represents very well dispersed nearly-spherical uniform nanostructures, with particle size of 75 ± 10 nm (Fig. 1(left upper panel). When folic acid was encapsulated into the IRMOF-3 moiety in a single step, the shape as well as size of IR-MOF-3 slightly altered; thus, the particle size of the resultant IRMOF-3@FA increased to about 83 ± 8 nm, as shown in Fig. 1 (right upper panel). Surface morphology of curcumin-encapsulated IRMOF-3 (IRMOF-3@CCM) was 95 ± 7, as shown in Fig. 1. When folic acid and curcumin were both encapsulated in IRMOF-3, there was no significant change in shape, but the particle size was found to be 117 ± 15 nm.
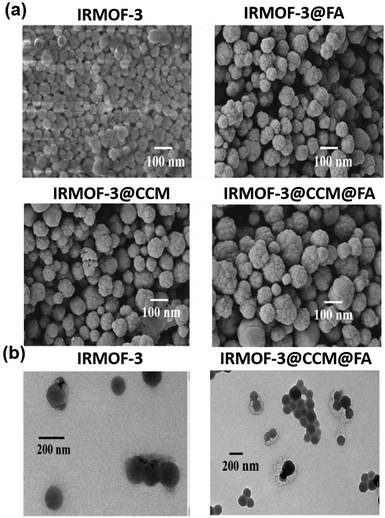 |
| Fig. 1 FESEM image of (a) IRMOF-3, IRMOF-3@FA, IRMOF-3@CCM, and IRMOF-3@FA@CCM (b) HRTEM image of (a) IRMOF-3 (b) IRMOF-3@FA@CCM. | |
Furthermore, elemental mapping analysis was performed to confirm the presence of elements in IRMOF-3@FA@CCM, as shown in Fig. S1 (ESI†). Zinc (Zn) [metal part] was found in IRMOF-3 and nitrogen (N), carbon (C) and oxygen (O) were present in the NH2BDC [organic ligand] moiety, folic acid [targeting ligand] and curcumin [drug]. IRMOF-3@FA@CCM was coated with platinum (Pt) before analysis.
Actual size of the as-synthesized IRMOF-3 and IRMOF-3@CCM@FA were determined by HRTEM analysis, as shown in Fig. 1. The HRTEM image of IRMOF-3 exhibited uniform and well dispersed nearly spherical particles with a size of about 78 ± 5 nm, as shown in Fig. 1(b). When folic acid and CCM were encapsulated inside the IRMOF-3 via a single step in situ hydrothermal process, the size of IRMOF-3 increased to about 90 ± 8 nm, as displayed in Fig. 1 (left panel). This size range of the particles is suitable for drug delivery and biological applications.17
Supplementary to the HRTEM study, DLS was performed to measure the average hydrodynamic particle sizes of the as-synthesized IRMOF-3, IRMOF-3@CCM, and IRMOF-3@CCM@FA. The hydrodynamic size of the as-synthesized IRMOF-3, IRMOF-3@CCM, and IRMOF-3@ CCM@FA were found to be 255.3 ± 7.99 nm, 342.7 ± 1.29 nm and 371.7 ± 8.80 nm, respectively [Table 1]. Particle size obtained via DLS (average particle size) is much higher than that obtained via HRTEM (actual particle size) because DLS was performed in an aqueous medium, whereas HRTEM was studied in a dry state. The surface charge (zeta potential) of IRMOF-3@FA@CCM was about −10.9 mV, which was more negative than only IRMOF-3, suggesting stability and dispersivity of IRMOF-3@FA@CCM in an aqueous medium.
Table 1 The hydrodynamic sizes and zeta potential values of the as-synthesized hybrid MOFs
Sample |
Size |
Zeta value |
PDI |
IRMOF-3 |
255.3 ± 7.99 |
−2.17 mV ± 0.15 |
0.341 ± 0.013 |
IRMOF-3@CCM |
342.7 ± 1.29 |
−0.907 mV ± 0.01 |
0.350 ± 0.015 |
IRMOF-3@CCM@FA |
371.7 ± 8.80 |
−10.9 mV ± 0.50 |
0.397 ± 0.004 |
FTIR analysis
Surface functional groups on the as-synthesized IRMOF-3, IRMOF-3@FA@CCM, IRMOF-3@CCM and IRMOF-3@FA were identified by performing FTIR spectroscopy (Fig. 2). The stretching vibration peaks of amine groups in the nanoscale IRMOF-3 were down-shifted from 3500 cm−1 and 3400 cm−1 to 3450 cm−1 and 3350 cm−1, respectively, in contrast to that of the pure NH2-H2BDC ligand (figure not shown) due to the formation of intra-framework hydrogen bonding of amine group with an electron donating oxygen from the carboxylic group.21 Characteristic FTIR peaks at 900 cm−1 and 1250 cm−1 were due to the C–H vibration band of nanoscale IRMOF-3. The bands at 1550 cm−1 and 1650 cm−1 evolved due to the benzene ring deformation.21 Maximum characteristic peaks of folic acid and curcumin are present in the FTIR spectrum of IRMOF-3@FA@CCM. Our results suggest the successful attachment of FA and CCM to the IRMOF-3.
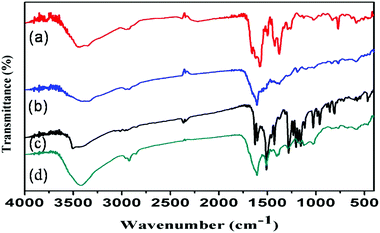 |
| Fig. 2 FTIR spectra of (a) IRMOF-3 (b) IRMOF-3@FA, (c) IRMOF-3@CCM and (d) IRMOF-3@FA@CCM. | |
Cell survivability
We explored the cytotoxic effect of the as-synthesized IRMOF-3, IRMOF-3@CCM, and IRMOF-3@CCM@FA (0–120 μM) on human and mice TNBC cell lines, namely, MDA-MB 468 and 4T1, respectively. It was found that cell survivability decreased in a dose dependent manner in IRMOF-3-, IRMOF-3@CCM-, and IRMOF-3@CCM@FA-treated cells. Also, the decrease in cell survivability was more in IRMOF-3@CCM@FA-treated cells for both cell lines (Fig. 3a and b). This study concludes that IRMOF-3@CCM@FA has a strong cytotoxic effect on both human and mice TNBC cell lines as compared with that of IRMOF-3, and IRMOF-3@CCM. IRMOF-3 has only slight cytotoxic effect. The LD50 doses for each of these complexes are compared in Table 2.
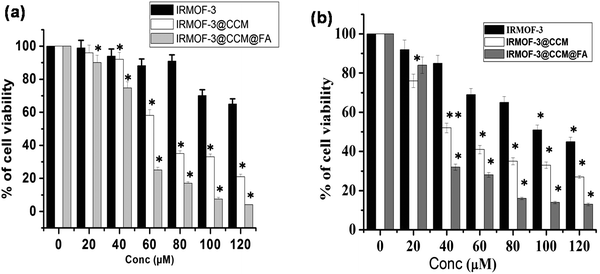 |
| Fig. 3 Cytotoxic effect of IRMOF-3, IRMOF-3@CCM, and IRMOF-3@CCM@FA on (a) MDA-MB 468, (b) 4T1 cells. | |
Table 2 The LD50 values of as-synthesized nanoparticles in 4T1 and MDA-MB-468 cell lines
Sample |
4T1 (μM) |
MDA-MB 468 (μM) |
IRMOF-3 |
98.66 ± 4.16 |
120 ± 5.61 |
IRMOF-3@CCM |
40.33 ± 4.04 |
62 ± 2.88 |
IRMOF-3@CCM@FA |
30.16 ± 3.58 |
45 ± 2.03 |
Drug release study
The cumulative drug release profile of IRMOF-3 was studied to understand the CCM discharge from the core of IRMOF-3@FA@CCM, as shown in Fig. S2 (ESI†). In this DDS, the drug loading content (DLC) and drug encapsulation efficiency (DEE) were found to be 52% and 98%, respectively. The high drug loading content was due to the extensively large surface area of IRMOFs. –NH2 group-functionalization inside and outside the micropores were also responsible for the high drug loading efficiency. Approximately 25% and 31% CCM were released from IRMOF-3@FA@CCM after 12 h and 24 h, respectively at pH 7.4. Furthermore, at pH 5.5, the corresponding release was 41% and 55%, respectively. These results suggest that the CCM release at pH 5.5 (intercellular acidic pH of cancer cells) was quicker than that at pH 7.4 (physiological condition).
Measurement of reactive oxygen species
Reactive oxygen species (ROS) were measured by both fluorescence microscopy and spectrofluorimetry methods using a specific probe, 2′,7′-dichlorofluorescein diacetate (DCF-DA). MDA-MB 468 and 4T1 cells were used for the determination of reactive oxygen species produced after IRMOF-3, IRMOF-3@CCM, and IRMOF-3@CCM@FA treatment at their respective LD50 doses for 12 h. From the fluorescence microscopic image, it can be seen that the green color fluorescence intensity increased for IRMOF-3@CCM@FA-treated cells in both the cell lines compared with that in control cells (Fig. 4a). From the spectrofluorimetry study, it was seen that ROS intensity increased by almost 1.5-fold for both cell lines in IRMOF-3@CCM@FA-treated cells (Fig. 4b).
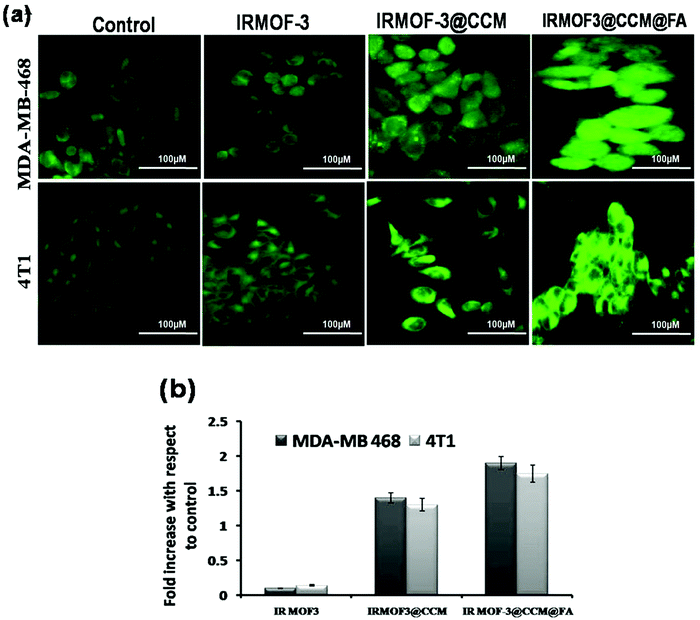 |
| Fig. 4 (a) Fluorescence microscopic images of nanoparticle-treated cells; (b) fluorescence intensity measurements of nanoparticle-treated cells. | |
Apoptotic nuclear morphology study by DAPI staining
Apoptotic morphology was studied in IRMOF-3@CCM@FA-, IRMOF-3@CCM- and IRMOF-3-treated cells at their respective LD50 doses for 12 h by DAPI staining. The untreated cells showed no nucleus shrinkage or polynuclear fragmentation. However, clear nuclear fragmentation and nucleus shrinkage was observed in IRMOF-3@CCM@FA-treated cells (Fig. 5a). Around 31% and 42% apoptotic cells were estimated in IRMOF-3@CCM@FA-treated MDA-MB 468 and 4T1 cells, respectively, whereas in control cells the corresponding values were 2% and 3% (Fig. 5b).
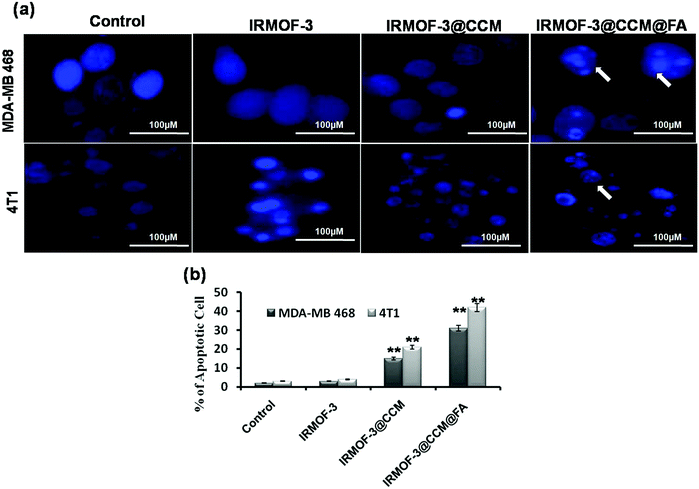 |
| Fig. 5 (a) Cellular morphology study by DAPI staining; (b) quantification data of apoptotic cells. | |
Mitochondrial membrane potential measurement
Production of ROS directly contributed to mitochondrial damage. Thus, we explored the effect of IRMOF-3@CCM@FA-, IRMOF-3@CCM-, IRMOF-3-treated MDA-MB 468 and 4T1 cells on their mitochondrial membrane potentials (MMP). Mitochondria depolarization is specifically indicated by cationic JC-1 dye. This dye exhibits potential-dependent accumulation in mitochondria, as indicated by a fluorescence emission shift from red to green; this occurs because the mitochondrial membrane loses its potential due to its damaged membrane. It was observed that IRMOF-3@CCM@FA- and IRMOF-3@CCM nanoparticle-treated cells have an increased green intensity (monomer-form of the dye) compared with that of the untreated cells, which appear red (aggregated-form of the dye) (Fig. 6a and b). This result showed that IRMOF-3@CCM@FA and IRMOF-3@CCM induced mitochondrial membrane damage and thereby reduced the membrane potential. Green and red color quantification data is also presented for both cell lines (Fig. 6c and d).
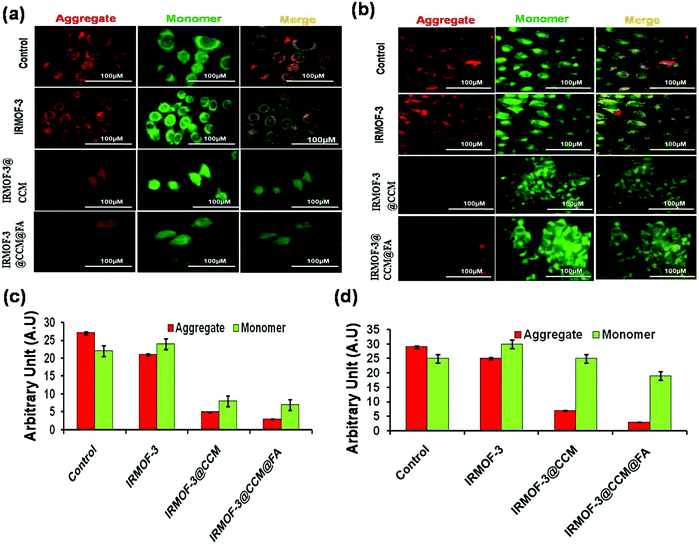 |
| Fig. 6 Mitochondrial membrane potential (MMP) measurement by JC1 dye on (a) MDA-MB 468 (b) 4T1 cells; (c), (d) quantification data of MMP in MDA-MB 468 and 4T1 cells, respectively. | |
Cell cycle and cell death study
As DNA damage and depolarized mitochondria are closely associated with cell cycle arrest, we analyzed the effects of these nanoparticles on cell cycle. As seen in Fig. 7a, when MDAMB-468 cells were treated with respective LD50 doses of IRMOF-3, IRMOF-3@CCM and IRMOF-3@CCM@FA for 12 h and 24 h, the percentage (%) of sub G1 cells increased from 1, 2, 2, and 5 to 8, 10, 23, and 47, respectively, indicating induction of apoptosis. Annexin V-FITC staining was performed and analyzed by FACS caliber for further confirmation. Cells shifted towards early to late apoptosis after treatment with LD50 doses (Fig. 5b). Percentage (%) of the apoptotic cells was higher in IRMOF-3@CCM@FA-treated cells for 12 h and 24 h compared with that of IRMOF-3- and IRMOF-3@CCM-treated cells. Almost 25% and 91% apoptotic cells were observed in IRMOF-3@CCM@FA-treated MDA MB 468 cells after 12 h and 24 h respectively, whereas 17% and 59% apoptotic cells were correspondingly observed in IRMOF-3@CCM-treated cells.
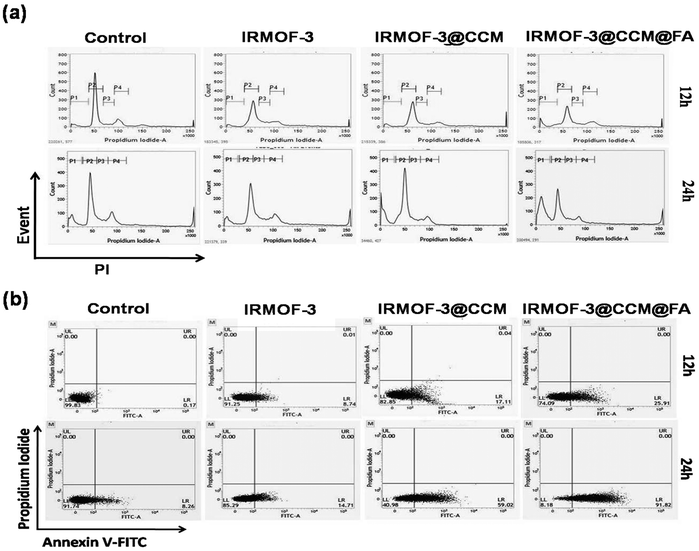 |
| Fig. 7 (a) Flow-cytometric analysis of the cell cycle distribution, illustrating the effect of nanoparticle-treated MDA-MB 468 cells at their respective LD50 doses at 12 h and 24 h respectively. (b) Dot plot of Annexin V-FITC/PI for the evaluation of apoptosis in MDA-MB 468 cells treated with nanoparticles at their respective LD50 doses at 12 h and 24 h. | |
Curcumin increases Bax/Bcl2 regulation through JNK pathway
We further tested the apoptotic proteins Bax, Bcl2, p53, and pBAD by Western blot, along with the Bax, Bcl-2 regulatory JNK pathway. As seen in Fig. 8, Bcl2 (anti-apoptotic), and pBAD expressions are reduced and Bax (pro-apoptotic) expression increased in IR-MOF3@CCM@FA-treated cells compared with that in IR-MOF3- and IR-MOF3@CCM-treated cells. Levels of JNK and p53 also increased. These observations along with data from Fig. 7 conclude that IRMOF-3@CCM@FA led ROS-induced apoptosis by disrupting the mitochondrial membrane potential. The expressions of apoptosis-related proteins (viz., Bax and Bcl2) were also assessed by real time quantitative PCR for further confirmation. The mRNA expression of Bax was upregulated in the curcumin treatment group, while mRNA expression of Bcl-2 was downregulated (Fig. 8g).
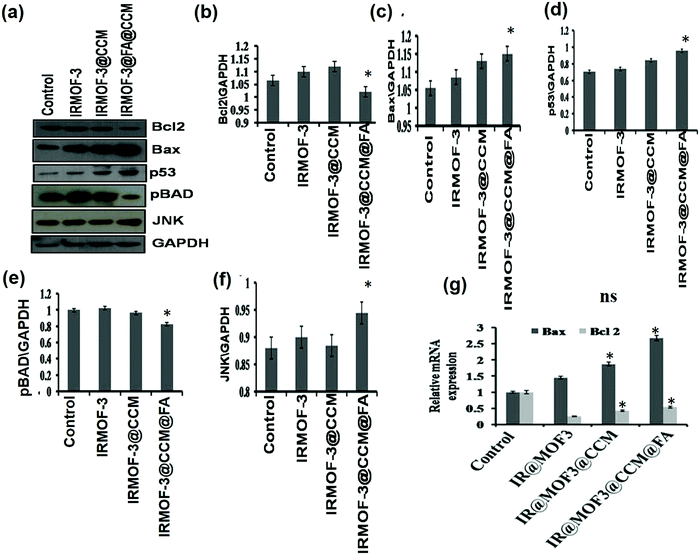 |
| Fig. 8 (a) Expression of apoptotic proteins by Western blot. (b–f) Quantification data of Bcl2, Bax, p53, pBAD and JNK, respectively. (g) Relative mRNA expression of Bax and Bcl2 proteins. | |
Expression of Notch1
Notch signaling has emerged as a pro-oncogene in TNBC. Thus, we explored the expression of Notch1 in IRMOF-3-, IRMOF-3@CCM-, and IRMOF-3@CCM@FA-treated MDA-MB 468 cells. In Fig. 9, it is seen that the expression of Notch1 decreased in IRMOF-3@CCM@FA-treated MDA MB 468 cells compared with that in the IRMOF-3- and IRMOF-3@CCM-treated MDA MB 468 cells.
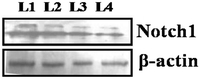 |
| Fig. 9 Notch1 expression in control (L1)-, IR-MOF3 (L2)-, IR-MOF3@CCM (L3)- and IR-MOF3@CCM@FA (L4)-treated cells. | |
In vivo study
For the assessment of tumor targeting in mice model, tumors were developed for 10 days. Next, IRMOF-3, IRMOF-3@CCM, and IRMOF-3@CCM@FA were used on triple negative tumor bearing BALB/c model on days 10, 15, 20, and 25. The tumor volume of mice was measured up to 30 days. As can be seen in Fig. 10a and b, the tumor volume was more suppressed in Group 3 (IRMOF-3@CCM-treated) and Group 4 (IRMOF-3@CCM@FA-treated) compared with that in the untreated group. On the final day (day 30), the average tumor volume of control, IR-MOF-3-, IR-MOF-3@CCM-, and IR-MOF-3@CCM@FA-treated mice were 0.86 mm3, 0.756 mm3, 0.479 mm3, and 0.332 mm3, respectively. We additionally measured the tumor weight and body weight of all mice groups. Tumor weight gradually decreased in Group 1, Group 2, Group 3 and Group 4 mice (Fig. 10c). On day 30, the average tumor weight of untreated, IR-MOF-3-, IR-MOF-3@CCM-, and IR-MOF-3@CCM@FA-treated mice were 451.33 mg, 278.13 mg, 115.8 mg, and 67.26 mg, respectively. Simultaneously, weight of the mice also slightly decreased in Group 1, Group 2, Group 3 and Group 4 mice (Fig. 10d).
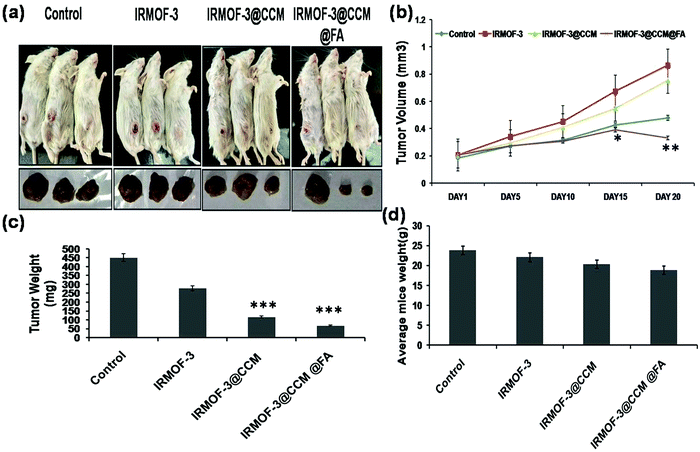 |
| Fig. 10 (a) Pictorial representation of mice; (b) measurement of tumor volume; (c) measurement of tumor weight and (d) measurement of body weight. | |
Histological analysis & toxicity study
To further assess the in vivo therapeutic efficacy and toxicity in the treated mice, histology analysis was employed (Fig. 11). The tumors were collected and sectioned for hematoxylin and eosin (H&E) staining of each group. The tumor cell densities were lower in Group 3 and Group 4 compared with that in Group 1 and Group 2 mice. Next, we measured various liver enzyme parameters, namely, ALP, SGOT, and ALT, as well as creatinine and urea levels in the serum as parameters for kidney function in different groups of untreated and treated mice. From this study, we showed that there is no significant toxicity to the liver and kidneys after treatment with the drugs (Table 3).
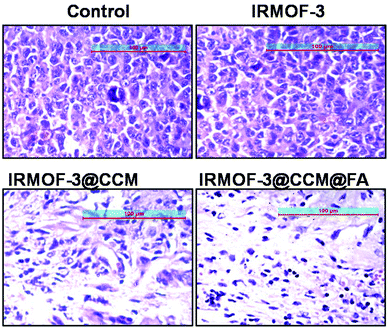 |
| Fig. 11 H & E staining of Group 1 (Control), Group 2 (IRMOF-3), Group 3 (IRMOF-3@CCM), and Group 4 (IRMOF-3@CCM@FA) mice tumor sections. | |
Table 3 Results of serum alkaline phosphatase assay: alanine transaminase, SGOT, serum urea, serum creatinine assay of Group 1 (Control), Group 2 (IRMOF-3), Group 3 (IRMOF-3@CCM) and Group 4 (IRMOF-3@CCM@FA) mice
Assay |
Control (Group 1) |
IR@MOF-3 (Group 2) |
IR@MOF-3@CCM (Group 3) |
IR@MOF-3@CCM@FA (Group 4) |
ALT (IU L−1) |
47.15 ± 2.35 |
49.23 ± 3.31 |
52 ± 1.79 |
48 ± 1.76 |
Serum urea (g mL−1) |
10.34 ± 0.95 |
12.51 ± 1.7 |
12.65 ± 0.86 |
12.9 ± 0.09 |
ALP activity (KA unit) |
12.14 ± 0.87 |
13 ± 0.25 |
13.91 ± 0.98 |
14.46 ± 0.97 |
SGOT activity (IU L−1) |
11.34 ± 0.15 |
18.2 ± 1.33 |
23 ± 1.01 |
13 ± 1.01 |
Serum creatinine (g mL−1) |
1.5 ± 0.08 |
2.1 ± 0.15 |
1.75 ± 0.09 |
1.73 ± 0.07 |
Discussion
Although various techniques are available for cancer therapy, TNBC, a subgroup of breast cancer that is frequently accompanied with higher frequency of gene mutations and negative ER, PR and HER2 receptors, remains a challenge in clinical practice due to the lack of biological targets and its resistance to chemoradiotherapy.22 Instead of treatment with conventional drugs, the administration of a natural compound as a therapeutic agent is an attractive way to maximize the outcome. This study investigated the effect of natural compound “curcumin” in TNBC treatment. Some natural products, particularly those obtained from medicinal herbs, have strong regulatory role in different diseases.23,24 Among these different natural compounds, curcumin has strong anticancer effects by modulating multiple cell signalling pathways.25–27 However, enveloping this drug into nanocarriers has significant advantages, including enhanced delivery, tumour targeting and improved efficacy together with low systemic toxicity.28,29 Current evidence shows that the porous metal organic framework-mediated DDS provides significant improvement in drug delivery.30,31 Thus, we loaded curcumin within a metal organic framework. First, we optimized the drug loading and drug release at different pH in an in vitro system. Folate receptor (FR) has been identified as a potential marker for therapeutic target for TNBC.32 Compared with other targeting agents such as antibodies and peptides, folic acid targeting is economically cheap, and it can be modified easily.32 We have successfully attached folic acid on the surface of MOFs, and the anti-proliferative activities of IRMOF-3, IRMOF-3@CCM, and IRMOF-3@CCM@FA were evaluated by MTT assay, MMP determination, and Annexin V FITC/PI double staining. Herein, we observed that folic acid-tagged MOFs (IRMOF-3@CCM@FA) show more cytotoxicity compared with that of IRMOF-3@CCM and IRMOF-3. Since mitochondria serve as the power house of cells and ROS production is also linked to mitochondrial depolarization, we measured mitochondrial membrane potentials.33 As a critical mitochondria function, MMP perturbation is commonly regarded as characteristic of early apoptosis, which can be observed by the shift in DCFDA fluorescence from red to green. Both generation of reactive oxygen species and mitochondrial membrane potential were observed to be much higher in IRMOF-3@CCM@FA-treated cells compared with that in IRMOF-3- and IRMOF-3@CCM-treated cells. The current study demonstrated that IRMOF-3@CCM@FA induces apoptosis through Bax\Bcl-2 regulation in MDA-MB 468 cells. The tumor suppressor gene p53 is involved in apoptosis partly by inducing Bax expression.34,35 In our study, we showed that p53 and JNK also increased in IF-MOF3@CCM@FA-treated cells. The mitochondrial apoptosis pathway inhibitor Bcl-2 exerts its action by blocking proapoptotic counterparts.36,37 In this study, there was a considerable decrease in Bcl-2 expression in IRMOF3@CCM@FA-treated cells, while the levels of Bax increased, resulting in an increase in ratio of Bax/Bcl-2. Real time PCR results also showed decreased expression Bcl-2 in IRMOF3@CCM@FA-treated cells compared with those in control and IRMOF3@CCM@FA-treated cells. This study clearly demonstrates that IFMOF3@CCM@FA-induced apoptosis in human triple negative breast cancer cells by downregulation of Bcl-2 and upregulation of Bax. Furthermore, JNK marker, the regulatory pathway of Bcl2 and Bax and p53, also increased. Notch signalling has also been described as a major player in triple negative cancer stem cells for its prognosis property.38 We have observed that curcumin-loaded IR-MOFs suppressed Notch1 signalling. Cleaved PARP and decreased Notch1 signalling was observed in cells treated with folic acid-conjugated drug. Next, we evaluated the efficacy of this drug IR-MOF system in mice triple negative breast cancer bearing tumor model. Tumor volume decreased significantly in IRMOF-3@CCM@FA-treated mice and also, no apparent liver and kidney toxicity was observed. In vitro and in vivo studies showed that our targeted DDS effectively delivered the potential anticancer drug, curcumin, to the triple negative breast cancer cell lines. These IR-MOF-3 can be deployed as effective nanovehicles for the targeted delivery of different anticancer drugs to the specific cancer cells, particularly those that are difficult to solubilize.
Conclusion
Herein, we developed a strategy for targeted delivery of natural compound “curcumin” in in vitro and in vivo triple negative breast cancer model. We encapsulated curcumin in a metal organic framework, and then conjugated folic acid on its surface. For in vitro study, we observed that FA-conjugated metal organic framework induced ROS-mediated DNA and mitochondrial DNA damage, followed by apoptosis. Tumor cell targeting was further tested on triple negative breast cancer cell bearing BALB/c model and promising results were observed. The tumor volume reduced significantly and survivability of the mice increased. This approach can be used to target cancer cells and may be implicated as a potential strategy for targeting breast cancer cells.
Conflicts of interest
There are no conflicts to declare.
Acknowledgements
The authors would like to thank Dept. of Biotechnology, Govt. of India for funding the research associate fellowship.
References
- L. A. Torre, F. Bray, R. L. Siegel and J. Ferlay,
et al., Global cancer statistics, Ca-Cancer J. Clin., 2012, 65, 87–108 CrossRef PubMed
.
- T. C. De Ruijter, J. Veeck and J. P. de Hoon,
et al., Characteristics of triple-negative breast cancer, J. Cancer Res. Clin. Oncol., 2011, 137, 183–192 CrossRef PubMed
.
- O. Brouckaert, H. Wildiers and G. Floris,
et al., Update on triple-negative breast cancer: prognosis and management strategies, Int. J. Womens Health, 2012, 4, 511–520 Search PubMed
.
- S. Bayraktar and S. Glück, Molecularly targeted therapies for metastatic triple-negative breast cancer, Breast Cancer Res. Treat., 2013, 138, 21–35 CrossRef CAS PubMed
.
- A. Shindikar, A. Singh and M. Nobrekar,
et al., Curcumin and Resveratrol as Promising Natural Remedies with Nanomedicine Approach for the Effective Treatment of Triple Negative Breast Cancer, J. Oncol., 2016, 9750785 Search PubMed
.
- S. C. Gupta, S. Patchva and B. B. Aggarwal, Therapeutic roles of curcumin: lessons learned from clinical trials, AAPS J., 2013, 15, 195–218 CrossRef CAS PubMed
.
- A. Goel and B. B. Aggarwal, Curcumin, the golden spice from Indian saffron, is a chemosensitizer and radiosensitizer for tumors and chemoprotector and radioprotector for normal organs, Nutr. Cancer, 2010, 62, 919–930 CrossRef CAS PubMed
.
- N. Rainey, L. Motte and B. B. Aggarwal,
et al., Curcumin hormesis mediates a cross-talk between autophagy and cell death, Cell Death Dis., 2015, 3(6), e2003 CrossRef PubMed
.
- P. Thulasiraman, G. Garriga and V. Danthuluri,
et al., Activation of the CRABPII/RAR pathway by curcumin induces retinoic acid mediated apoptosis in retinoic acid resistant breast cancer cells, Oncol. Rep., 2017, 37, 2007–2015 CrossRef CAS PubMed
.
- A. P. Hu, J. V. Morabito and C. K. Tsung, Core–Shell Catalysts of Metal Nanoparticle Core and Metal–Organic Framework Shell, ACS Catal., 2014, 12, 4409–4419 CrossRef
.
- R. D. Kennedy, V. Krungleviciute and D. J. Clingerman,
et al., Carborane-Based Metal–Organic Framework with High Methane and Hydrogen Storage Capacities, Chem. Mater., 2013, 25, 3539–3543 CrossRef CAS
.
- R. C. Huxford, J. Della Rocca and W. Lin, Metal-organic frameworks as potential drug carriers, Curr. Opin. Chem. Biol., 2010, 14, 262–268 CrossRef CAS
.
- T. Kundu, S. Mitra, P. Patra and A. Goswami,
et al., Mechanical downsizing of a gadolinium(III)-based metal-organic framework for anticancer drug delivery, Chemistry, 2014, 20, 10514–10518 CrossRef CAS PubMed
.
- J. Della Rocca, D. Liu and W. Lin, Nanoscale metal-organic frameworks for biomedical imaging and drug delivery, Acc. Chem. Res., 2011, 44, 957–968 CrossRef CAS PubMed
.
- H. Zhang, W. Jiang and R. Liu,
et al., Rational Design of Metal Organic Framework Nanocarrier-Based Codelivery System of Doxorubicin Hydrochloride/Verapamil Hydrochloride for Overcoming Multidrug Resistance with Efficient Targeted Cancer Therapy, ACS Appl. Mater. Interfaces, 2017, 9, 19687–19697 CrossRef CAS PubMed
.
- R. Paulmurugan, R. Bhethanabotla and K. Mishra,
et al., Folate Receptor-Targeted Polymeric Micellar Nanocarriers for Delivery of Orlistat as a Repurposed Drug against Triple-Negative Breast Cancer, Mol. Cancer Ther., 2016, 15, 221–231 CrossRef CAS PubMed
.
- A. R. Chowdhuri, S. Tripathy and C. Haldar, Single step synthesis of carbon dot embedded chitosan nanoparticles for cell imaging and hydrophobic drug delivery, J. Mater. Chem. B, 2015, 3, 9122–9131 RSC
.
- M. Zheng, S. Liu and G. Xingang,
et al., One-Step Synthesis of Nanoscale Zeolitic Imidazolate Frameworks with High Curcumin Loading for Treatment of Cervical Cancer, ACS Appl. Mater. Interfaces, 2015, 7, 22181–22187 CrossRef CAS PubMed
.
- A. Pramanik, D. Laha, S. K. Dash and S. Chattopadhyay,
et al., An in-vivo study for targeted delivery of copper-organic complex to breast cancer using chitosan polymer nanoparticles, Mater. Sci. Eng., C, 2016, 68, 327–337 CrossRef CAS PubMed
.
- D. Laha, A. Pramanik and J. Maity,
et al., Interplay between autophagy and apoptosis mediated by copper oxide nanoparticles in human breast cancer cells MCF7, Biochim. Biophys. Acta, 2014, 1840, 1–9 CrossRef CAS PubMed
.
- M. Zhao, K. Deng and L. He,
et al., Core-shell palladium nanoparticle@metal-organic frameworks as multifunctional catalysts for cascade reactions, J. Am. Chem. Soc., 2014, 136, 1738–1741 CrossRef CAS
.
- E. A. O'Reilly, L. Gubbins and S. Sharma,
et al., The fate of chemo-resistance in triple negative breast cancer (TNBC), BBA Clin., 2015, 12, 257–275 CrossRef
.
- D. A. Dias, S. Urban and U. A. Roessner, Historical overview of natural products in drug discovery, Metabolites, 2012, 16, 303–336 CrossRef
.
- H. F. Ji, X. J. Li and H. Y. Zhang, Natural products and drug discovery. Can thousands of years of ancient medical knowledge lead us to new and powerful drug combinations in the fight against cancer and dementia?, EMBO Rep., 2009, 10, 194–200 CrossRef CAS PubMed
.
- D. O. Levitsky and V. M. Dembitsky, Anti-breast Cancer Agents Derived from Plants, Nat. Prod. Bioprospect., 2015, 5, 1–16 CrossRef CAS
.
- D. Perrone, F. Ardito and G. Giannatempo,
et al., Biological and therapeutic activities, and anticancer properties of curcumin, Exp. Ther. Med., 2015, 10, 1615–1623 CrossRef CAS
.
- M. Rivera, Y. Ramos and M. Rodríguez-Valentín, Targeting multiple pro-apoptotic signalling pathways with curcumin in prostate cancer cells, PLoS One, 2017, 19, 0179587 Search PubMed
.
- R. Watkins, L. Wu and C. Zhang,
et al., Natural product-based nanomedicine: recent advances and issues, Int. J. Nanomed., 2015, 10, 6055–6074 CAS
.
- F. Zhou, B. Feng, T. Wang, D. Wang, Q. Meng, J. Zeng, Z. Zhang, S. Wang, H. Yu and Y. Li, Programmed Multiresponsive Vesicles for Enhanced Tumor Penetration and Combination Therapy of Triple-Negative Breast Cancer, Adv. Funct. Mater., 2017, 27, 1606530 CrossRef
.
- X. Gao, M. Zhai and W. Guan,
et al., Controllable Synthesis of a Smart Multifunctional Nanoscale Metal-Organic Framework for Magnetic Resonance/Optical Imaging and Targeted Drug Delivery, ACS Appl. Mater. Interfaces, 2017, 7, 3455–3462 CrossRef
.
- X. Gao, X. Hai and H. Baigude,
et al., Fabrication of functional hollow microspheres constructed from MOF shells: promising drug delivery systems with high loading capacity and targeted transport, Sci. Rep., 2016, 23(6), 37705 CrossRef
.
- A. Cheung, H. J. Bax and D. H. Josephs, Targeting folate receptor alpha for cancer treatment, Oncotarget, 2016, 32, 52553–52574 Search PubMed
.
- F. Zhou, B. Feng, T. Wang, D. Wang, Z. Cui, S. Wang, C. Ding, Z. Zhang, J. Liu, H. Yu and Y. Li, Theranostic Prodrug Vesicles for Reactive Oxygen Species-Triggered Ultrafast Drug Release and Local-Regional Therapy of Metastatic Triple-Negative Breast Cancer, Adv. Funct. Mater., 2017, 2727, 1703674 CrossRef
.
- L. E. Leard and V. C. Broaddus, Mesothelial cell proliferation and apoptosis, Respirology, 2004, 9, 292–299 CrossRef
.
- S. A. Amundson, T. G. Myers and A. J. Fornace Jr, Roles for p53 in growth arrest and apoptosis: putting on the brakes after genotoxic stress, Oncogene, 1998, 17, 3287–3299 CrossRef PubMed
.
- S. R. Narasimhan, L. Yang and B. I. Gerwin, Resistance of pleural mesothelioma cell lines to apoptosis: relation to expression of Bcl-2 and Bax, Am. J. Physiol., 1998, 275, L165–L171 CAS
.
- C. C. Fang, C. J. Yen and T. J. Tsai, Antibiotics induce apoptosis of human peritoneal mesothelial cells, Nephrology, 2013, 8, 142–149 CrossRef
.
- N. E. Bhola, V. M. Jansen and J. P. Koch, Treatment of Triple-Negative Breast Cancer with TORC1/2 Inhibitors Sustains a Drug-Resistant and Notch-Dependent Cancer Stem Cell Population, Cancer Res., 2016, 76, 440–452 CrossRef CAS
.
Footnote |
† Electronic supplementary information (ESI) available. See DOI: 10.1039/c8nj03350a |
|
This journal is © The Royal Society of Chemistry and the Centre National de la Recherche Scientifique 2019 |