DOI:
10.1039/C9NH00368A
(Review Article)
Nanoscale Horiz., 2019,
4, 1256-1276
Engineering surface states of hematite based photoanodes for boosting photoelectrochemical water splitting
Received
31st May 2019
, Accepted 21st June 2019
First published on 21st June 2019
Abstract
Hematite-based photoanodes are promising candidates for photoelectrochemical water splitting. However, the performance of pristine hematite semiconductors is unsatisfactory due to charge recombination occurring at different interfaces: back contact, bulk and semiconductor/electrolyte interfaces. Increasing efforts have been focused on enhancing the performance of hematite based photoanodes via nanostructure control, doping, heterojunction construction, and surface modification with a secondary semiconductor or an oxygen evolution electrocatalyst. Most of the previous studies attributed the enhanced PEC water splitting performance to the changes in the donor density via doping, the formation of type II heterojunction via a secondary semiconductor coating and the improved water oxidation kinetics via coating oxygen evolution electrocatalysts. However, the role of surface states presented at the semiconductor/electrolyte interfaces of hematite-based photoanodes has been overlooked in previous investigations, which virtually play a critical role in determining the photoelectrochemical water oxidation process. In this review, we summarize the recent progress of various techniques employed for the detection of surface states of hematite photoanodes and highlight the important role of modifying surface states in the development of high performance hematite based photoanodes for photoelectrochemical water splitting application. The challenges and future prospects in the study of hematite based photoanodes are also discussed.
1. Introduction
The increasing global energy demand of modern society drastically conflicts with the finite fossil fuel supply in nature, motivating plenty of research efforts for the development of sustainable and environmentally friendly energy sources.1 Inexhaustible solar energy is among the most promising candidates, and has been utilized widely in photovoltaic approaches.2 However, the fluctuant and intermittent nature of insolation make such applications unsuitable for the storage and dispatch of solar energy for consumption.3 Accordingly, conversion of solar energy into the form of chemical bonds is an attractive approach for efficient, economical, and convenient utilization of solar energy.4–6 Among these strategies, photoelectrochemical (PEC) water splitting devices, using earth abundant semiconductors, have for long been considered to be the ‘Holy Grail’ of the solar energy conversion revolution.7–9
As an earth abundant semiconductor, hematite photoanodes have been intensively investigated as photoanodes for PEC water splitting because of several promising properties, like high abundancy in nature, environmental-friendliness, high photochemical stability, a narrow bandgap (1.9–2.2 eV), and a theoretical maximum solar-to-hydrogen (STH) efficiency of 15.4%.10,11 However, their relatively low absorption coefficient, short excited-state lifetime (10−6 s),12,13 poor oxygen evolution reaction kinetics, short hole diffusion length, and poor electrical conductivity lead to multiple electron–hole recombination pathways occurring in the bulk, interfaces, and surfaces, which significantly limits the PEC activity of hematite photoanodes.11 A rapid charge transport and transfer between the back substrate, the photoactive semiconductor, the electrocatalyst and the electrolyte are necessary for an efficient STH performance.14 Recently, plenty of researchers have devoted their efforts to the development of various nanostructures, doping, heterojunction construction, and surface modification with a secondary semiconductor or oxygen evolution electrocatalyst for improving the PEC performance of hematite based photoanodes.11,15–35 Typically, they attributed the enhanced PEC water splitting performance of hematite composite electrodes to the changes in the donor density via doping, improved charge separation efficiency via constructing type II heterojunctions, and the enhanced water oxidation kinetics via coating an oxygen evolution electrocatalyst.11,15–35 However, the investigation about the surface state mediated charge transfer at the semiconductor–electrolyte interfaces of hematite based photoanodes has not been properly considered by the research community of PEC water splitting, which is critical for understanding the PEC mechanism and further improving their PEC performance.
In this review, we will correct this deficiency and highlight the role of surface states present at the hematite/electrolyte interfaces, which is important for further development of hematite-based photoanodes for PEC water splitting.36,37 Specifically, we will review the development of hematite-based photoanodes following this outline: (i) the fundamental concept of photoelectrochemistry and surface states; (ii) detecting the surface states of hematite via different techniques; (iii) engineering of surface states of hematite photoanodes.
2. The fundamental concept of photoelectrochemistry and surface states
The free energy required for the conversion of 1H2O molecule to H2 and 1/2O2 molecules under standard conditions is ΔG = 237.2 kJ mol−1.3 According to the Nernst equation, it corresponds to ΔE° = 1.23 V per transferred electron. Theoretically, a semiconductor with a band gap energy (Eg) larger than 1.23 eV can drive the hydrogen evolution reaction (HER) and oxygen evolution reaction (OER) using electrons/holes generated under illumination (Fig. 1) if it has a conduction band edge energy (Ecb) and a valence band edge energy (Evb) that straddle the electrochemical potentials of E°(H+/H2) and E°(O2/H2O). To drive these two reactions, photoinduced electrons or holes in the semiconductor bulk must travel to the semiconductor/electrolyte interfaces (SEI), and then react with the electrolyte species directly at the semiconductor surface without recombination. The charge-transfer process at SEI results in losses because of the concentration and kinetic overpotentials for driving the HER and the OER. Therefore, the energy required for photoelectrochemical water splitting at a photoelectrode is usually reported as 1.6–2.4 eV per generated electron–hole pair.38,39
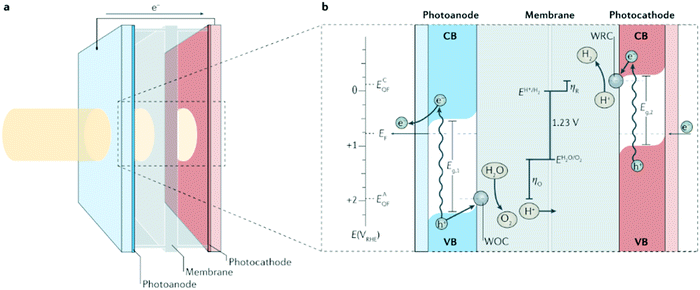 |
| Fig. 1 Photoelectrochemical cell basics. (a) Schematic of a ‘wired’-type tandem cell for water splitting with incident solar illumination striking the photoanode and transmitting to the photocathode. (b) Working principle of the tandem cell for water splitting using a photoanode with bandgap energy Eg,1, and a photocathode with Eg,2 (where Eg,1 > Eg,2). Briefly, on absorption of a solar photon, an electron (e−) from the valence band (VB) is promoted to the conduction band (CB) leaving the corresponding electron hole (h+). The electric field in the depletion layer physically separates these charges and, in the photocathode, the electrons in the CB drift to the semiconductor–liquid junction, increasing the quasi-Fermi energy of the cathode, ECQF, to drive the reduction of H+ to H2 at a water reduction catalysis (WRC) site. Analogously, in the photoanode, electron holes in the VB drift to the semiconductor–liquid junction, increasing the photoanode's quasi-Fermi energy, EAQF, sufficiently to surmount the overpotential for oxidation (ηO) and oxidize water to O2 at a water oxidation catalysis (WOC) site. Photogenerated electrons in the CB of the photoanode travel through the external circuit to recombine with the holes in the VB of the photocathode. EF, Fermi energy; E(VRHE), electronic potential with respect to the reversible hydrogen electrode; ηR, overpotential for reduction. Reproduced with permission from ref. 10. | |
Whereas, at the surface of a practical semiconductor, the periodic crystal symmetry is broken and thus, produces electronic states within the bandgap, which are named surface states/mid-gap states.40 The surface states present at the surface of semiconductors can either be intrinsic or extrinsic, which relies on the environmental conditions of the semiconductors. In the field of semiconductor photoelectrochemistry, surface states play a vital role in the kinetics of interfacial reactions at illuminated semiconductor electrodes. Recently, the investigation of surface states affecting the kinetics of Fe2O3, BiVO4, CuWO4, GaN, CuFeO2, Ta3N5, and Cu(In,Ga)(Se,S)2 photoelectrodes for PEC water splitting has drawn plenty of attention.41–48
Typically, in photoanodes, surface states can act as recombination centres for holes generated by light, therefore, surface recombination competes intensively with charge transfer from the semiconductor to the electrolyte. Specifically, under illumination, the valence band of a photoanode obtains abundant excess of holes, which can be transferred to the electrolyte by a direct charge transfer mechanism to launch the solar fuel production reaction. However, surface states trap hole carriers, leading to another favourable pathway for indirect charge transfer and a new undesirable recombination pathway.49,50 A simple kinetic model neglecting any electrostatic influence, like the presence of an electric field has been presented in Fig. 2 to show the effect of surface state-assisted electron–hole recombination and indirect charge transfer processes on photoelectrochemical water splitting. At low bias potential, direct hole transfer from the valence band can be neglected and only three processes occur at this interface: trapping/detrapping of electrons, trapping/detrapping of holes and charge transfer of holes. Hence, from this picture, it is clear that surface state-assisted recombination and hole transfer to the solution have a common factor, which is hole trapping/detrapping by surface states.51,52 Therefore, it is of great interest to distinctly detect the surface states present on the photoelectrodes and to deeply understand the critical role that the surface states play in the PEC water splitting process.
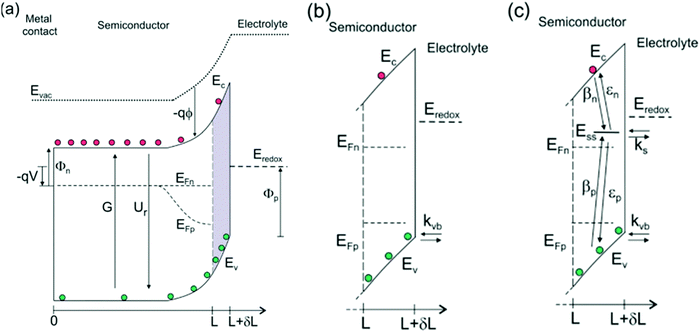 |
| Fig. 2 (a) Energy diagrams of an n-type semiconductor of thickness L where electrons and holes are generated at a rate G and recombine at a rate Ur. At the interface of length δL, hole transfer to the redox level Eredox can take place (b) directly from the valence band (kinetic constant kvb) and (c) indirectly from surface states at low bias and directly from the valence band at higher bias. In this latter model, we consider trapping of electrons and holes (βn and βp) and detrapping (εn,εp) and hole transfer from surface states (ks) at the energy level Ess, in competition with direct hole transfer. In these schemes Ec and Ev are the energies of the lower edge of the conduction band and the higher edge of the valence band, respectively. EFn and EFp are the quasi-Fermi levels, Φn and Φp are the injection barriers of electrons and holes, Evac is the local vacuum level, ϕ is the local electrostatic potential and V is the applied voltage. Reproduced with permission from ref. 51. | |
3. Detecting the surface states of hematite via different techniques
3.1 Photoelectrochemical impedance spectroscopy (PEIS)
The photoelectrochemical oxygen evolution process on hematite involves 4-electron transfer/O2 molecule. It is hypothesized that the OER reaction steps involve the formation of higher-valent iron states at the surface by hole capture. Eqn (1)–(4) illustrate the complications arising from the fact that the oxidation of water is a 4-electron process, therefore, holes need to be ‘stored’ in intermediate states. The Fe(IV) and Fe(V) intermediates in this scheme can also act as electron acceptors, so that surface recombination reactions of the kind shown in eqn (5) and (6) are likely to take place. The Fe(IV) and Fe(V) states can be thought as ‘surface-trapped holes’, which may have sufficient surface mobility to allow second order reactions of the type illustrated by the last step shown in eqn (1)–(4).53 | Fe(III)surf + h+ → Fe(IV)surf | (2) |
| Fe(IV)surf + h+ → Fe(V)surf | (3) |
| 2Fe(V) + 2H2O → 2Fe(III) + O2 + 4H+ | (4) |
Based on the assumed possible mechanism in eqn (1)–(6), L. M. Peter et al. firstly discussed and derived the possibility of using photoelectrochemical impedance spectroscopy (PEIS) to illustrate the surface states present at the surface of hematite photoanodes, as displayed in Fig. 3.53
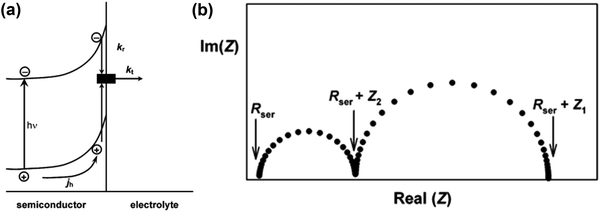 |
| Fig. 3 (a) Phenomenological kinetic scheme for PEIS analysis. (b) Typical PEIS response predicted by eqn (7). Reproduced with permission from ref. 53. | |
Fig. 3a is a simplified version of the model used in the previous discussions to describe the surface state mediated charge transport in hematite photoanodes, which excludes direct hole transfer from the valence band to solution redox; and a typical PEIS response shown in Fig. 3b predicted by L. M. Peter et al. based on the derivation of eqn (7):
| 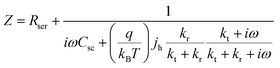 | (7) |
where
Rser is the series resistance,
Csc is the space charge capacitance,
jh is the current density corresponding to the flux of holes reaching the interface, and
ω is the radial frequency.
kt and
kr are the first order rate constants for interfacial transfer and recombination, respectively.
53
Furthermore, J. Bisquert and T. Hamann et al. systematically investigated the selection of applicable electrical equivalent circuits for fitting the PEIS data to the interpretation of the surface states present on photoelectrodes.54–59 In order to illustrate the PEC measurements of the hematite photoanodes under illumination and dark conditions, J. Bisquert and T. Hamann et al. adopted a classical view, as depicted in Fig. 4a, to interpret the electrochemical impedance spectrum (EIS) measurements.60 The equivalent circuit model (ECM) shown in Fig. 4b highlights the central role of the surface states acting as a recombination center, trapping electrons from the conduction band and holes from the valence band, as given by Rtrapping. The surface states affecting the charge transfer of holes to the donor species in solution are described by Rct,trap, and another pathway for direct charge transfer from the semiconductor bands is included as Rct,bulk.54,61
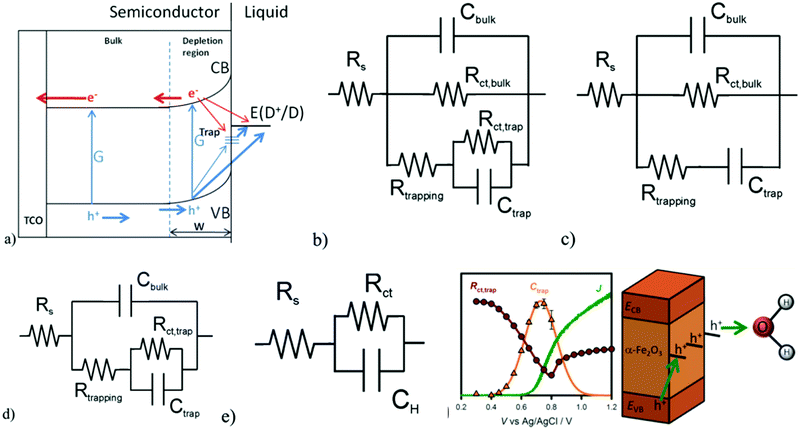 |
| Fig. 4 (a) Proposed physical model for the charge carrier dynamics in hematite electrodes, showing their generation, G, by light absorption, surface-state trapping, and interfacial charge-transfer reactions. (b) Equivalent circuit corresponding to the physical model in (a). (c) Simplified model used for IS interpretation created by removing Rct,trap. (d) Simplified model used for IS interpretation created by removing Rct,bulk. (e) Randles circuit. (f) J(V) curve (green solid line) and Ctrap (orange triangles) and Rct,trap (red circles) values obtained for a 60 nm hematite electrode under 1 sun illumination and pH 6.9 with the schematic showing the surface state mediated hole transportation at the hematite/electrolyte interface. Reproduced with permission from ref. 54. | |
However, the general ECM proposed in Fig. 4b, including the surface-state hole-trapping process, cannot unambiguously fit the EIS because it does not discriminate between Rct,bulk and Rct,trap. Therefore, two simplifications of this general ECM have been employed, as shown in Fig. 4c and 4d. In these simplifications, either Rct,trap or Rct,bulk is eliminated. The simplified ECs are excellent approximations if charge transfer for PEC water oxidation is dominated by one route, either from the valence band (Fig. 4c) or surface states (Fig. 4d). They fitted the EIS data under illumination with these simplified models and found that more consistent results were obtained for the ECM displayed in Fig. 4d.54 Therefore, the simplified ECM in Fig. 4d is employed to derive the fitting parameters from all the EIS measurements under illumination for the EIS analysis, while the Randle circuit in Fig. 4e is utilized for the fitting of EIS data obtained under dark conditions. Fig. 4f reveals that the strong correlation between the Ctrap peak with the Rct,trap valley and the photocurrent onset clearly indicates that the hole-transfer step leading to water oxidation takes place predominantly from surface trapped holes, and not directly from valence band holes. The PEIS methodology proposed by J. Bisquert and T. Hamann et al. not only provides new insight into the PEC water oxidation process of pristine hematite photoanodes, but also will be of great utility in further investigations of complex hematite composite systems to determine the density and the energy level of the surface states and charge transfer efficiency.54
3.2 Intensity modulated photocurrent spectroscopy (IMPS), intensity modulated photovoltage spectroscopy (IMVS) and distribution of relaxation times (DRT) analysis
IMPS and IMVS are relatively uncommon techniques that probe the dynamic relation between light illumination and the electrochemical response of the photoelectrodes. IMPS is a powerful small amplitude perturbation technique that can deconvolute the rate constants for charge transfer and recombination at illuminated semiconductor electrodes mediated by the surface states.62–66 L. M. Peter et al. proposed a generalized theoretical model for intensity modulated photocurrent spectroscopy (IMPS) and derived the analytical equations for the frequency dependence of the photocurrent and photovoltage responses.62 This theory treats the influence of the space charge, the surface states and the Helmholtz capacitances on the IMPS response. In the model, the charge transfer and recombination at the SEI are described by kinetic equations, whereas the surface states charging and the current through the external circuit are treated using linear circuit equations.62 In this way, the kinetic constant of the surface state-mediated charge transfer and charge recombination in the photoelectrode system can be readily obtained from the IMPS plot. IMVS is conceptually similar to IMPS, but it is not as popular as IMPS as a measurement technique. It probes the relationship between light intensity and photovoltage at a constant photocurrent response. These photoelectrochemical immittance triplets (PIT), consisting of three electrochemical techniques (PEIS, IMPS and IMVS) based on electrochemical impedance and optical modulation used to probe the dynamic performance of photoelectrodes for PEC water splitting, have been mathematically and phenomenologically compared by A. Rothschild et al.67
In addition to these established PIT analysis methods, there is another approach, DRT-based empirical analysis, which is a distribution function that can be calculated for any impedance spectrum without any a priori assumption.67,68 The most useful characteristic of DRT analysis is its capability to separate polarization processes more clearly than in common Nyquist or Bode plots, where they usually appear convoluted. Since it circumvents the construction of ECM, which always depend on presumptions and are never unique, DRT analysis is a powerful tool to support impedance data analysis.67,68 For instance, A. Rothschild et al. employed DRT analysis to study hematite photoanodes in alkaline electrolytes, where they identified two dominant polarization processes: the first process is surface recombination, which is dominant at low potentials and is suppressed by adding a hole scavenger (H2O2) to the electrolytes; the second process is suspected to be the formation of double-bonded oxygen intermediates (Fe
O), which dominates the water oxidation reaction at high potentials (without H2O2) and the H2O2 photo-oxidation reaction at all potentials.68,69
3.3 Cyclic voltammetry
T. W. Hamann et al. demonstrated that the surface state trapped holes in hematite photoanodes could be measured by using cyclic voltammetry in the dark.63 Specifically, holes could accumulate on the hematite photoanode surface by applying a positive potential under illumination followed by measuring the cathodic current in the dark as the potential is scanned negatively and the surface states are reduced. As shown in Fig. 5, the cathodic current peak observed in the dark cyclic voltammetry scan is well consistent with the Css peak obtained in the fitted EIS data, indicating the presence of surface states in hematite photoanodes.63
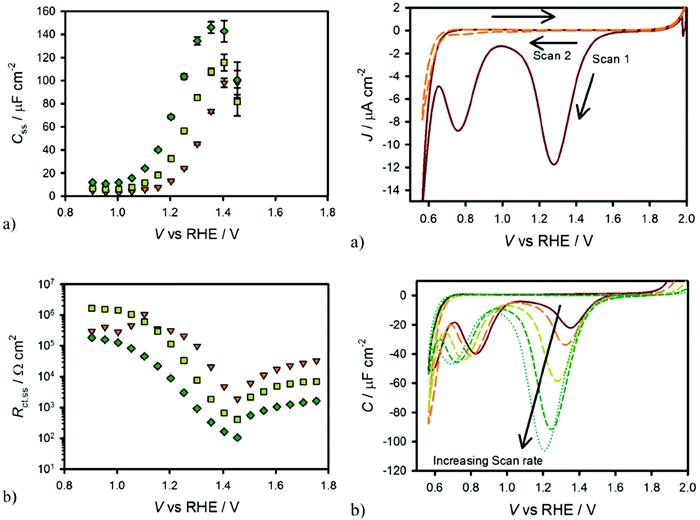 |
| Fig. 5 Left: Equivalent circuit parameters obtained from fitting EIS data for a hematite electrode in contact with H2O (a and b) under 0.1 sun (orange triangles), 0.33 sun (yellow squares) and 1 sun (green diamonds) illuminations. Right: (a) Cyclic voltammetry of hematite in H2O scanned at 200 mV s−1 after holding the electrode at a potential of 2 V vs. RHE for 60 seconds under 1 sun illumination. The first cycle is a solid red line and the second cycle is a dashed orange line. (b) CVs measured under the same conditions as in (a) at different scan rates: 20 mV s−1 (red, solid), 50 mV s−1 (orange, long dash), 200 mV s−1 (yellow, medium dash), 500 mV s−1 (green, short dash) and 1000 mV s−1 (blue, dots). Reproduced with permission from ref. 63. | |
3.4 Operando UV-vis
Considering that the hematite surface plays a significant role in determining the efficiency of water oxidation, it is of great interest to directly identify the identity of the relevant hematite surface species via in situ techniques to evidence the surface state mediated charge transfer mechanism hypotheses. T. Hamann and H. Irie et al. detected the intermediate species during the oxygen evolution reaction via in situ UV-vis absorption spectroscopy (Fig. 6a) for atomic layer deposition and spray deposition hematite photoanodes.70,71 For instance, T. Hamann et al. quantitatively measured the formation of the Fe(IV) intermediate states by integrating the absorption peak at 572 nm via operando UV-vis experiments, as displayed in Fig. 6b and c; the absorption features at 572 nm could be correlated with the Css obtained in PEIS, which is assigned to the oxidation of a low-valent Fe–H2O or Fe–OH to a high-valent Fe
O group at the hematite photoanode surface.70 Additionally, the coating of a single layer of alumina by atomic layer deposition onto the bare hematite photoanode reduced the UV-vis absorption features because there are fewer exposed iron atoms at the hematite surface, and fewer iron hydroxyls are able to be generated upon oxidation by a photogenerated hole.70
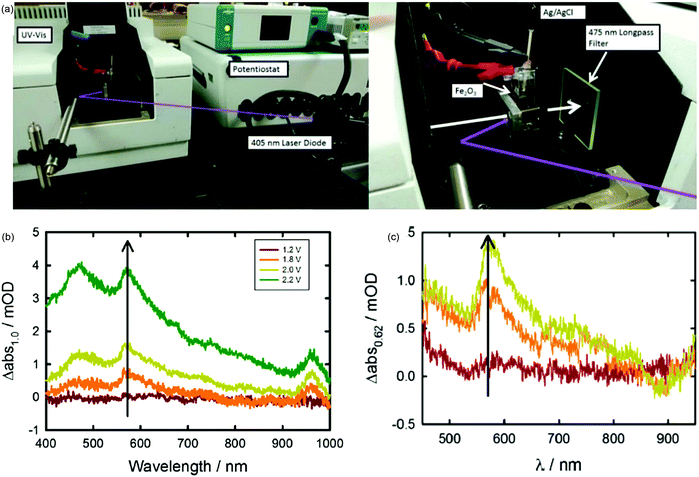 |
| Fig. 6 (a) Experimental setup of spectroelectrochemical measurements performed under monochromatic illumination. (b) Change in absorption spectra measured vs. 1.0 V vs. RHE. Spectra measured in the dark and under applied potentials of 1.2 (red line), 1.8 (orange line), 2.0 (yellow line) and 2.2 (green line) V vs. RHE. (c) Change in absorption spectra measured at 0.82 V (red), 1.22 V (orange), and 1.62 V versus RHE (yellow) measured under 0.1 mW cm−2 405 nm illumination with respect to the absorption spectra measured at 0.62 V. Reproduced with permission from ref. 70. | |
3.5
In situ infrared (IR) spectroscopy
It is well established that infrared spectroscopy is a powerful technique for the determination of intermediate species in catalytic reactions.72–76 Additionally, the utilization of attenuated total reflectance infrared (ATR-IR) spectroscopy is supposed to overcome challenges including the low concentration of intermediates on electrode surfaces and the competitive infrared absorption by water.75 T. Hamann et al. investigated the water-oxidation reaction on hematite via in situ ATR-IR spectroscopy, and directly observed the existence of the FeIV
O intermediate during the PEC water splitting process, as shown in Fig. 7.72 As displayed in Fig. 7(c) and (d), a potential- and light-dependent absorption peak located at 898 cm−1 in in situ ATR-IR spectra is assigned to a FeIV
O group, which is an intermediate in the PEC water oxidation reaction. These results provide direct evidence of high-valent iron–oxo intermediates as the product of the first hole transfer reaction on the hematite surface and represent an important step in establishing the mechanism of PEC water oxidation on semiconductor electrodes.72 J. C. Zhao et al. investigated the reaction order of interfacial hole transfer by rate law analysis based on EIS measurements and probed the high-valent FeIV
O intermediates by operando Fourier-transform infrared (FT-IR) spectroscopy, finding that in highly alkaline electrolytes, coupling of adjacent surface trapped holes becomes the dominant mechanism with a reaction orders of ∼2.76
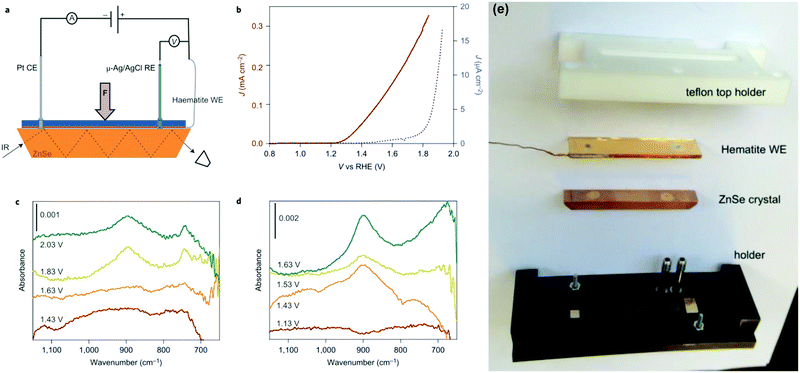 |
| Fig. 7 Experimental set-up and results of infrared spectroscopy measurements during electrochemical and PEC water oxidation. (a) This schematic of the set-up used for operando PEC infrared (IR) measurements depicts the hematite working electrode (WE), platinum counter electrode (CE), Ag/AgCl reference electrode (RE) and ZnSe ATR crystal. A thin layer of electrolyte was introduced between the hematite WE and the ATR crystal. (b) J–V curves of a hematite electrode in the operando PEC infrared set-up measured in contact with D2O in the dark (blue) and under illumination (dark red). (c) Infrared spectra of hematite scanned at constant applied potentials, from 1.43 to 2.03 V versus RHE, in the dark. (d) Infrared spectra of hematite scanned at constant applied potentials, from 1.13 to 1.63 V versus RHE, under illumination. Peaks at 898 and 743 cm−1 appear at potentials positive to the onset of the water-oxidation current, 1.7 V versus RHE in the dark and 1.25 V versus RHE under illumination, which indicates that they may be species involved in the water-oxidation reaction. (e) The ATR-IR cell assembly parts. Reproduced with permission from ref. 72. | |
3.6. Four dimensional transmission electron microscopy (4D TEM)
Nonetheless, the aforementioned mechanistic investigations of hematite based photoanodes are limited to obtain macroscopic information of the FeIV
O intermediates via in situ spectroscopies, like UV-vis, in situ ATR-IR spectroscopy, PEIS, IMPS, IMVS, and DRT, but without spatially disclosing the location of the surface intermediates or active sites.78 The shortage of an accurate spatial identification of the OER active sites (FeIV
O) in hematite photoanodes currently hinders the clear determination of the OER mechanism of hematite photoanodes for water splitting. Recently, by employing core-loss electron energy loss spectroscopy (EELS) signals (of several hundred eV), coupled to femtosecond temporal resolution as well as ultrafast energy-filtered 4D TEM, A. H. Zewail et al. have identified that FeIV
O has a lifetime of a few picoseconds; they also studied the associated photoinduced electronic transitions and charge transfer processes in hematite photocatalysts, as shown in Fig. 8.77 It demonstrates that the direct observation of FeIV
O intermediates at hematite photoanodes with spatial, temporal and energy resolution is possible via 4D TEM.
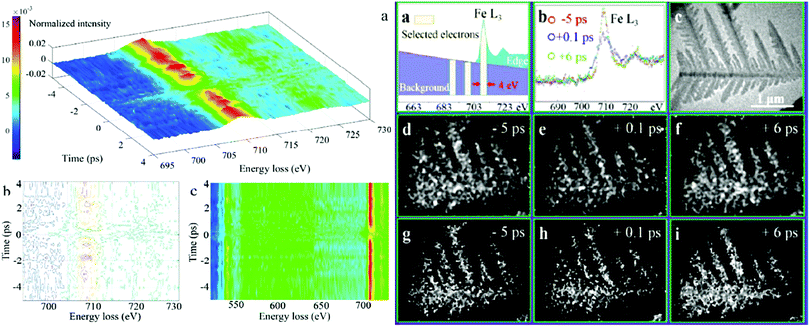 |
| Fig. 8 Left: (a) 3D intensity-energy-time dynamics of the overall Fe L-edge FEELS. (b) Intensity contour map of (a). (c) Top view of Fe L3-edge (above 700 eV) and O K-edge (around 540 eV) FEELS. Right: (a) Electrons centred at 709.5, 699.5 and 689.5 eV energy loss are selected by a slit window of 4 eV for the elemental mapping and the jump-ratio images. (b) Time-dependent EELS of Fe L3-edge with background subtracted. (c) Ultrafast TEM image of a typical Fe2O3 particle without energy filtering. Temporal change of iron elemental mapping images (d–f) and jump-ratio images (g–i) of the α-Fe2O3 particle obtained by a three-window method with two pre-edge (699.5 ± 2 eV, 689.5 ± 2 eV) and one post-edge (709.5 ± 2 eV), and a two-window method with one pre-edge (699.5 ± 2 eV) and one post-edge (709.5 ± 2 eV), respectively. Reproduced with permission from ref. 77. | |
3.7 Density functional theory (DFT)
Some other theoretical researchers investigated the nature of surface states in hematite based photoanodes via DFT calculations.79–81 B. Iandolo et al. investigated the surface states on hematite under photoelectrochemical conditions and calculated the density of states for two relevant hematite atomic terminations via DFT calculations, where the presence and the role of surface states were rationalized. It predicted a Nerstian dependence of the OER onset potential on pH, which was to a very good extent confirmed by PEC measurements and EIS characterization.79 Meanwhile, M. C. Toroker et al. performed first-principles calculations with DFT+U on several possible adsorbates at the α-Fe2O3(0001) surface, ruling out the existence of a stable peroxo Fe–O–O–Fe adsorbate. They demonstrated that the origin of the surface absorption peak at 580 nm could be a Fe–O˙ type bond that acts as an essential intermediate for water oxidation; the projected density of states (PDOS) of the *O intermediate shows a mid-gap state, which is located far below the conduction band edge, but close to the valence band edge, as shown in Fig. 9.80
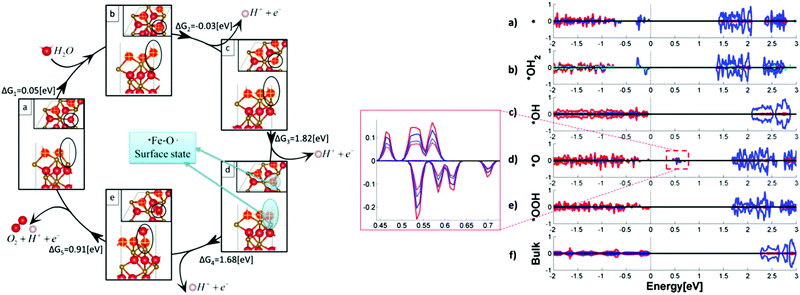 |
| Fig. 9 Left: Intermediates and free energies at zero bias for water oxidation over pure Fe2O3(0001). In the frames, side and top views of the intermediates’ surface: (a) *vac, (b) *OH2, (c) *OH, (d) *O, and (e) *OOH. Spheres in red, gold, and white represent O, Fe, and H atoms, respectively. Marked in yellow are the surface oxygen atoms. The location of the active site with adsorbates is circled in black. The location of the surface state is circled in blue at the *O intermediate. Right: Projected density of states (PDOS) of five water oxidation intermediates of the pure Fe2O3(0001) surface: (a) *vacancy, (b) *OH2, (c) *OH, (d) *O, (e) *OOH intermediates, and (f) bulk Fe2O3. O, Fe, and H total DOS is in red, blue, and green plain lines, respectively. Enlarged is the mid-gap states for the *O intermediate, with the p orbital of O atom at the active site and the d orbital of the neighbouring Fe atom in red and blue broken lines, respectively. DOS is normalized to a maximum total density of one. Reproduced with permission from ref. 80. | |
3.8 Scanning electrochemical microscopy (SECM)
SECM is a versatile electrochemical platform used in the characterization of electrocatalysts and photoelectrodes to collect products and measure electron transfer kinetics, which is useable in the PEC water splitting field.82–84 For instance, A. J. Bard et al. employed surface interrogation scanning electrochemical microscopy (PEC SI-SECM) for the quantitative determination of the reaction intermediates, where a light pulse to a hematite film at a given potential generates intermediates that are then analysed by a tip generated titrant at known times after the light pulse.83 The density of photoactive sites on a hematite surface, proposed to be Fe4+ species in a redox titration, was successfully quantified to be 18 Fe atoms nm−2; the pseudo-first order reaction rate constants were determined as 0.03 to 0.19 s−1 based on time-dependent redox titration experiments.83
3.9 Kinetic isotope effect (KIE)
Understanding the mechanism of water oxidation to O2 represents the bottleneck towards the design of an efficient PEC water splitting device. It is well established that water oxidation on hematite is mediated by surface trapped holes, characterized to be the high valent –Fe
O species. However, the mechanism of the subsequent rate-limiting O–O bond formation step is still a missing piece. Recently, mechanistic studies of water oxidation at hematite photoanodes via kinetic isotope effects have drawn researchers's attention.76,85,86 For instance, J. C. Zhao et al. demonstrated the pivotal role of proton transfer in water oxidation on hematite photoanodes using PEC characterization, the H/D kinetic isotope effect, and PEIS. They found that the rate-determining interfacial hole transfer to water molecules at the hematite surface is a concerted proton–electron transfer process at pH < 12. A mechanistic transition in the rate-determining step from concerted proton–electron transfer (CPET) to electron transfer (ET) occurred after OH− became the dominant hole acceptor.85
4. Engineering of surface states on hematite photoanodes
4.1 Intrinsic modification engineering
4.1.1 Sintering parameters.
The sintering parameters, including temperature, period and atmosphere, play a vital role in the PEC performance of hematite based photoanodes because they are supposed to reduce the crystal defects, to affect the donor density via Sn doping from FTO substrates, to create oxygen vacancies (VO) or to modify the surface states of the photoelectrodes.87–99 For instance, J. S. Jang et al. examined the role of FTO deformation during the activation of hematite photoanodes on FTO substrates, as displayed in Fig. 10a.89 The incorporation of Sn dopants from the FTO substrates into the hematite lattice was confirmed by X-ray photoelectron spectroscopy and was found to increase with sintering time. Specifically, under 800 °C annealing, the FTO substrates underwent a stoichiometric change that directly affected their electrical conductivity; their resistivity was doubled after 20 min of sintering at 800 °C. Activation of hematite photoanodes by high-temperature sintering entails a kinetic competition between Sn dopant diffusion from the FTO substrate into the hematite and the resulting thermal deformation and conductivity loss in the FTO substrates.89 Therefore, the increasing photocurrent response is attributed to the enhanced Sn doping and thus the improved donor density in the hematite based photoanodes.87–89
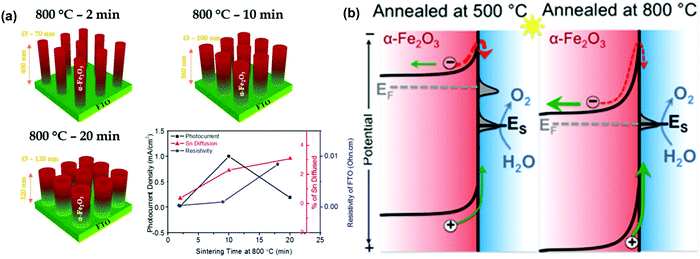 |
| Fig. 10 (a) Activation of hematite photoanodes by high-temperature sintering entails a kinetic competition between Sn dopant diffusion from the FTO substrate into the hematite, and the resulting thermal deformation and conductivity loss in the FTO substrate. Reproduced with permission from ref. 89 (b) simplified band diagram of hematite electrodes under conditions with (left) and without (right) Fermi level pinning by the sub-conduction band surface states. Reproduced with permission from ref. 90. | |
It is reported that controlling the sintering temperature may not only induce Sn diffusion into the hematite matrix, but also alter the surface states present at the surface of the hematite based photoanodes.90–94 For instance, T. W. Hamann et al. demonstrated that annealing hematite at 800 °C substantially improves the water oxidation efficiency of the ultrathin film hematite electrodes, which is ascribed to the removal of the surface states close to the conductance band of the hematite photoanodes and the reduction in recombination and Fermi level pinning, as displayed in Fig. 10b.90 Moreover, Y. Li et al. reported a novel fire treatment, which was adopted to activate two types of hematite photoanodes (sol–gel thin films and hydrothermal nanowires), which successfully removed the surface trap states (surface hydroxyls) on hematite and thus resulted in an optimized photocurrent density of 2.63 mA cm−2.92 Additionally, Y. D. Li et al. found that the cooling rate during the sintering process is an important factor deciding PEC water splitting of hematite photoanodes. Rapid cooling of the hematite photoanodes increased the photocurrent from 0.635 mA cm−2 for the normally cooled sample to 0.856 mA cm−2 at 1.23 V vs. RHE. The rapidly cooled hematite nanowires showed improved Ctrap and decreased Rct,trap values, indicating the improvement of the hole transfer capability and the acceleration of the surface charge transfer via modifying the surface states, respectively.93
On the other hand, the sintering atmosphere is another important parameter that might affect the PEC performance of hematite photoanodes. It is well established that oxygen vacancies (VO) in hematite photoanodes can be readily obtained via sintering the electrodes in an oxygen deficient atmosphere.95–97 Y. Li et al. reported a novel method for the preparation of highly conductive hematite photoanodes through thermal decomposition of FeOOH in an oxygen-deficient atmosphere (N2 + air). They showed substantially enhanced photoactivity compared to the pristine hematite prepared in air. The oxygen content during thermal activation significantly affects the formation of VO and thus the photoactivity of hematite nanowires for PEC water oxidation.95,96 A. J. Cowan et al. proposed that VO may act as a ‘mixed blessing’, with the enhanced electrical properties being balanced with a potential increase in trap-mediated electron–hole recombination at the surface defect sites introduced.96 Recently, L. Z. Wang et al. carried out a comprehensive analysis of the key charge transfer and surface reaction steps in PEC processes on hematite photoanodes to understand the role of VO in hematite photoanodes. Moreover, they also clarified that VO can facilitate the surface electrocatalytic processes while leading to severe interfacial recombination at the semiconductor/electrolyte interface, in addition to the well-reported improvements in bulk conductivity.97 Additionally, H2 treatment during the sintering process could introduce vacancies as well as mid-gap states in the photoactive semiconductor for water splitting.98–100 For instance, G. A. Ozin et al. presented a means of activating hematite towards PEC water splitting via thermal treatment of ultrathin films of hematite under an atmosphere of 5% H2; it was determined that VO is the most likely structural change following hydrogen treatment. Surface state analyses using electrochemical techniques revealed a probable change in the mechanism of PEC water oxidation as well as the appearance of a new surface state coincident with the onset of photocurrent.99
4.1.2 Acid/alkali media surface treatments.
It has been reported that the surface states of hematite electrodes can be modified via treating the hematite photoanodes in acidic or alkaline media, leading to enhanced charge separation efficiency and thus improved PEC water splitting performance.101–103 Y. Li et al. investigated the effect of acidic media (including acetic acid, HCl, HNO3, and H3PO4) treatment on hematite photoanodes. They demonstrated that a simple acid treatment method modifies either the nature or position of the available trap sites, which substantially enhances the efficiency of electrons moving out of traps in hematite nanowire photoanodes, and thus reduces the electron–hole recombination loss and improves PEC performance.101 Meanwhile, J. H. Ye et al. found that the photocurrent densities increased 3- and 2-times for α-Fe2O3 and Ti:α-Fe2O3 photoanodes after KOH treatment, respectively. They demonstrated that a conformal thin layer grafted with hydroxyl (–OH) groups was formed on the hematite surface, which behaved like an electrocatalyst to accelerate the water oxidation kinetics on hematite photoanodes.102
4.1.3 Re-growth.
Disordered hematite structures can be synthesized at low cost and on a large scale; however, they typically suffer from charge recombination and poor PEC water splitting performance. Additionally, the structural disorders on or near the hematite surfaces are important causes of the low photovoltages. Recent investigations reveal that the surface disordered hematite structures can be corrected by a re-growth strategy, which pave the way for producing efficient photoanodes based on hematite.104,105 For instance, D. W. Wang et al. developed a facile regrowth strategy to reduce surface disorders and as a consequence, a turn-on voltage of 0.45 V (versus reversible hydrogen electrode) was achieved.104 Moreover, A. Tricoli et al. employed capillary force-induced re-structuring and chemically-induced re-growth methods, to drastically increase the photocurrent by 24 times from 0.032 mA cm−2 of the initially disordered hematite photoanodes to 0.79 mA cm−2 at 1.23 V vs. RHE under simulated 1 sun irradiation.105
4.2 Extrinsic engineering
4.2.1 Doping/a secondary semiconductor coating.
Considerable attention has been drawn to the development of composite hematite photoanodes with doping or secondary semiconductors, to further enhance the PEC water oxidation efficiency.106–116 Meanwhile, other researchers spared no effort in correlating PEC performance improvement with the surface state evolution upon doping or coating a secondary semiconductor onto hematite photoanodes.117–128 For instance, D. Monllor-Satoca et al. prepared mesoporous hematite–titania composite films by mixing the respective preformed nanoparticles obtained by a non-aqueous sol–gel route in a wide range of loading levels (0–20 mol%). Voltammetric and EIS studies were performed observing an optimum 10% doping, with a 15-fold photocurrent increase (up to 1.3 mA cm−2 at 1.23 V vs. RHE) and a 100-fold decrease of the charge transfer resistance. The roles of surface states and donor densities were highlighted, assuming a charge transfer mechanism through hole trapping at surface states and its isoenergetic transfer to water; an optimum 10–15% doping range was obtained, where the maximum overlapping between surface and water states was prevalent (Fig. 11). The tailoring of the donor states (conductivity), phase coexistence (solubility), heterojunctions (energetics) and surface states (kinetics) in the hematite composite photoanodes plays a decisive role in determining the PEC water oxidation performance.117
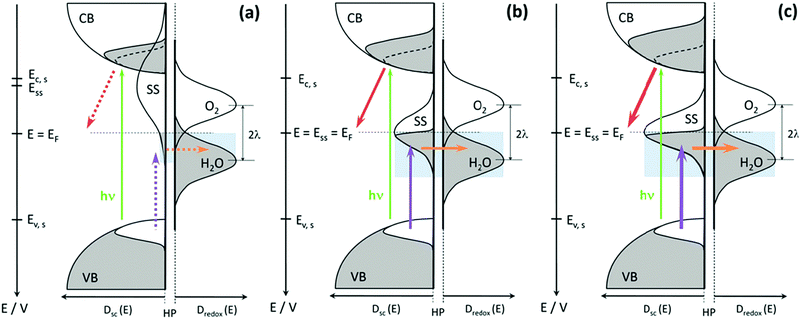 |
| Fig. 11 Kinetic scheme of the charge generation and transfer processes at the biased (1.23 VRHE) semiconductor electrolyte interface (SEI) under illumination and for different titania doping levels, including: (a) no doping (0%), (b) low or large doping (5% and/or 20%) and (c) optimum doping (10–15%). Grey and white areas refer to electron filled or empty states, respectively. The dotted lines inside the conduction band (CB) filled states denote photogenerated electrons with the same relative area as the empty states at the valence band (VB); the enlarged grey areas highlight the bulk (core) doping levels, which are shown intentionally oversized for comparison purposes. The green arrow refers to the charge generation process upon visible photon absorption (hν); the purple arrow refers to the hole trapping process at surface states (SS); the orange arrow refers to the hole transfer process from SS to water molecules; the red arrow refers to (photo)electron transfer from semiconductor CB states to the underlying conducting substrate (FTO). The thickness and shape of the arrows indicate the relative rates of the charge transfer processes, where the dotted line represents the slowest rate (a) and the thickest line represents the fastest rate (c). The light blue shaded areas refer to the relative overlapping of the SS and water density of states (DOS). Note that the relative size of the SS distribution for the non-doped sample (a) has been intentionally enlarged to highlight the overlapping between the semiconductor and the redox couple states. Dredox: normalized DOS of the redox couple in the electrolyte; Dsc: DOS of the semiconductor; E: electrode potential; Ec,s: surface CB edge potential; EF: Fermi level of the semiconductor that matches the electrode potential (E) and the O2/H2O couple thermodynamic potential (1.23 VRHE); ESS: center potential of the SS distribution; Ev,s: surface VB edge potential; HP: Helmholtz plane at the SEI, across which the charge transfer process occurs; l: redox couple reorganization energy. Reproduced with permission from ref. 117. | |
C. Li et al. systematically investigated the energy distribution of surface states (Css) and dj/dV on pure Fe2O3, Ti–Fe2O3, Al2O3/Ti–Fe2O3 and H2O2/Ti–Fe2O3 electrodes.118 As displayed in Fig. 12, there is only one surface state present in pure Fe2O3, Ti–Fe2O3 and H2O2/Ti–Fe2O3, whereas there are two kinds of surface states appearing on the Al2O3/Ti–Fe2O3 electrode; additionally, compared to the energy levels of the surface state peaks in pure Fe2O3, those of Ti–Fe2O3, Al2O3/Ti–Fe2O3 and H2O2/Ti–Fe2O3 have been shifted close to the valence band edge.118 Therefore, these results reveal that surface Ti modification (Ti–Fe2O3 electrode), Al2O3 coating by ALD (Al2O3/Ti–Fe2O3 electrode), and H2O2 treatment (H2O2/Ti–Fe2O3 electrode) indeed modify the energy levels and density of the surface states and the corresponding PEC water splitting performance of hematite based photoanodes. B. X. Zhou et al. revealed that coating of ZnFe2O4 and ZnO layers onto hematite modified the surface states of hematite and thus affected its PEC performance.126 C. M. Li et al. employed a co-doping method by sequential in situ Ti-doping and ex situ Mg-doping in order to tailor the surface states of hematite photoanodes for great improvement of the charge transfer at the interface and the subsequent transport ability by the suppressed charge recombination. This resulted in about 11-fold higher photocurrent than that of undoped Fe2O3 at 1.23 V vs. RHE. This was mainly due to Mg and Ti-doping into Fe2O3 modifying the surface states enabling participation of more holes in water oxidation and better kinetics that enhanced charge transfer and suppressed charge recombination for efficient water oxidation.121 Additionally, T. A. Kandiel et al. reported that Ba- and Sr-modified hematite photoanodes exhibited higher density of surface states and higher charge transfer rate constants at low bias potential than those of bare hematite, which might be the reason for the higher photocurrent observed for Ba- and Sr-modified hematite photoanodes.128
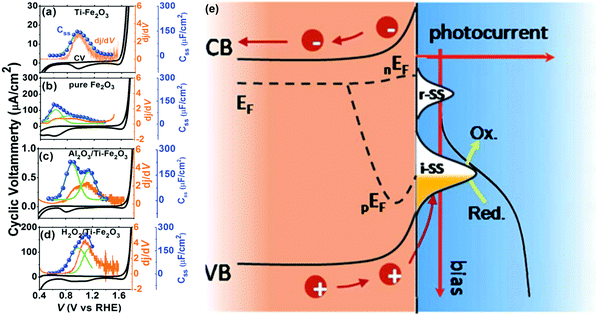 |
| Fig. 12 The relation between surface states and photocurrent based on the results of CV (black), Css (blue) and dj/dV (orange) resulted from the electrodes of (a) Ti–Fe2O3, (b) pure Fe2O3, (c) Al2O3/Ti–Fe2O3, and (d) H2O2/Ti–Fe2O3 photoanodes. The Css is fitted with Gauss plots (green line) and the iR-drop is compensated. (e) The schematic illustration of photo-electrochemical water oxidation on a hematite photoanode with surface states as intermediates. Reproduced with permission from ref. 118. | |
4.2.2 Oxygen evolution catalyst (OEC) overlayer deposition.
The deposition of oxygen evolution catalysts (OEC) onto the hematite photoanodes would alter the surface states and thus enhance the PEC performance of hematite photoanodes.129–139 For instance, G. A. Ozin et al. demonstrated that for the case of hematite/iridium catalyst composite photoanodes, the iridium catalyst not only improved the kinetics of the water oxidation reaction both in the dark and under illumination, but also increased the density of photoholes at the surface and decreased the rate of recombination of majority charge carriers with these surface-bound charges at moderate to high applied potentials.129 A. B. F. Martinson et al. reported that hematite photoanodes coated with an ultrathin Co(OH)2/Co3O4 layer by atomic layer deposition (ALD) resulted in significantly enhanced photoelectrochemical water oxidation performance. The enhanced PEC performance (100–200 mV cathodic shifts in the photocurrent onset potential, 0.81 mA cm−2 photocurrent responses at 1.23 V vs. RHE) is attributed to modified surface states which enable faster charge transfer from the surface states of Co(II)/Co3O4-modified hematite photoanodes than the charge transfer from the surface states of bare hematite electrodes.130 It was reported by T. W. Hamann and D. R. Gamelin et al. that the deposition of cobalt phosphate (Co-Pi) onto the hematite photoanode surface changes the nature of the surface states in hematite and thus could introduce an alternative pathway for water oxidation catalyzed by Co-Pi, which allows the composite photoanode to avoid positive charge accumulation at the a-Fe2O3 surface.131,132
Meanwhile, D. W. Wang et al. reported that when a thin layer of a heterogenized molecular Ir catalyst (het-WOC) is applied onto a hematite photoanode, the photocurrent response is improved from ca. 0.25 mA cm−2 to 0.66 mA cm−2 at 1.23 V vs. RHE in an acidic electrolyte.133 A detailed mechanistic investigation about the system via PEIS and IMPS reveals that the enhanced PEC performance is primarily due to the improved charge transfer (42 fold), while the surface recombination rate remains unchanged. In stark contrast, heterogeneous oxide catalyst (IrOx) coatings improve the PEC performance of hematite by significantly reducing the surface recombination rate. These results suggest that the het-WOC provides additional charge-transfer pathways across the Fe2O3|H2O interface, while IrOx and similar bulk metal-oxide catalysts replace the Fe2O3|H2O interface with a fundamentally different one, as displayed in Fig. 13.134 Recently, S. Eslava et al. unravelled the nature of the interactions between different loadings of an electrocatalyst (CoFeOx) and a hematite semiconductor via a combination of electrochemical approaches of PEIS and IMPS. They found that a thin CoFeOx layer mainly reduces surface charge recombination, while an extremely thin CoFeOx layer enhances charge transfer kinetics; an interlayer of GaOx modifies the surface state distribution and increases the charge transfer rate.137
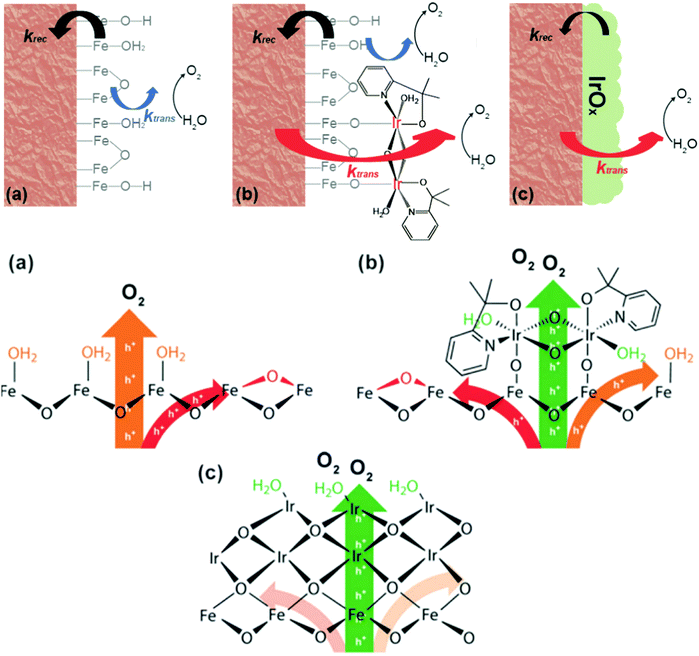 |
| Fig. 13 Top: Schematic illustrations of the kinetic models for (a) bare hematite (b) hematite with het-WOC and (c) hematite with IrOx. Bottom: Mechanistic perspective of bare hematite (a), hematite with het-WOC (b) and hematite with IrOx (c). Reproduced with permission from ref. 134. | |
4.2.3 Doping, secondary semiconductors and OEC overlayers.
Other researchers have devoted their efforts to the combination of secondary semiconductors and OEC overlayers to modify the donor density and the surface states of hematite photoanodes for further enhancing the PEC performance of hematite-based photoanodes.14,120,125,140 X. L. Zheng et al. reported a facile and rapid three-step approach to simultaneously reduce the bulk, interface, and surface recombinations for a hematite nanorod array based photoanode, leading to a greatly improved photocurrent density at low bias potential.140 In this strategy, flame-doping enhances the Ti doping concentration without hampering the morphology and surface properties of the hematite nanorods, which effectively reduces both the bulk and surface recombinations. Then, the addition of a dense layer between the hematite nanorods and the FTO glass substrate reduces the interfacial recombination by suppressing the electron back-injection into the electrolyte. Finally, sequential oxalic acid etching and FeOOH deposition improve both the interface quality between the FeOOH electrocatalyst and the hematite nanorods and the surface catalytic activity.140
Meanwhile, J. T. Ma et al. fabricated a NiO/P-Fe2O3 composite photoanode which produced a high photocurrent density of 2.08 mA cm−2 at 1.23 V vs. RHE and a 110 mV cathodic shift of the onset potential, which was ascribed to the improved electrical conductivity and the modified surface states because of the P doping and NiO coating.120 Moreover, J. T. Ma et al. further demonstrated that F-doping and NiOOH coating onto hematite photoanodes resulted in enhanced PEC water splitting performance, fulfilling the requirements of low surface trapping sites, high conductivity, efficient charge separation and injection efficiency, as shown in Fig. 14.125
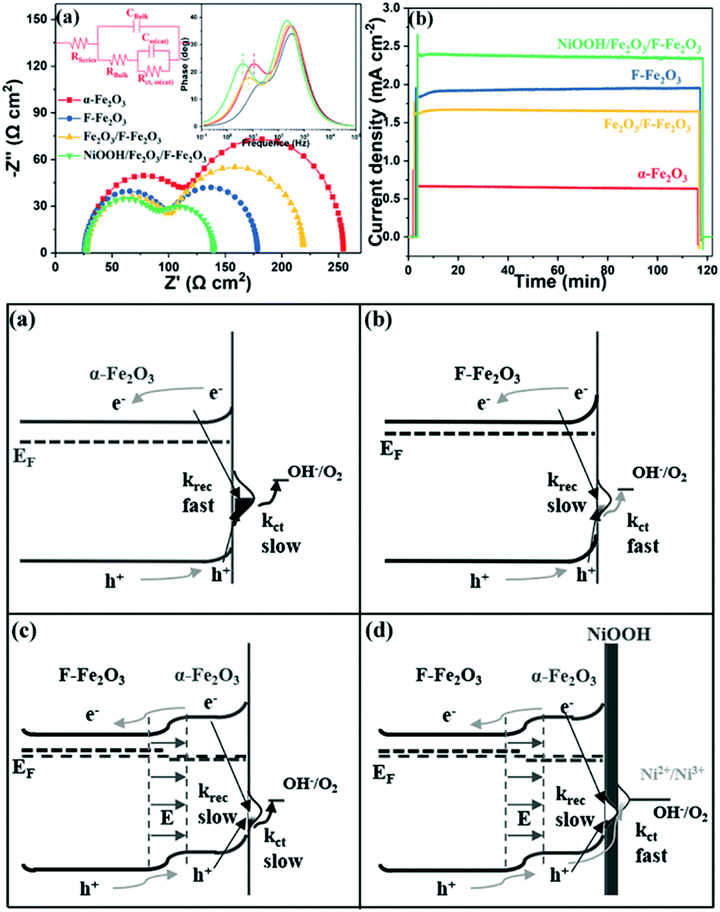 |
| Fig. 14 Top: (a) Nyquist plots of the samples at 1.05 V vs. RHE under illumination and (b) chronoamperometry of all photoanodes in a period of 2 h. Bottom: Energetic schemes for (a) Fe2O3, (b) F-Fe2O3, (c) Fe2O3/F-Fe2O3 NRs and (d) NiOOH/Fe2O3/F-Fe2O3 photoanode, where krec refers to the surface recombination and kct represents charge transfer through surface states. Reproduced with permission from ref. 125. | |
Recently, we have reported the fabrication of ITO/Fe2O3/Fe2TiO5/FeNiOOH multi-layer nanowires (Fig. 15) for PEC water splitting and elucidated the mechanism underlying the interfacial coupling effect of the quaternary hematite composite photoanode.14 In this work, secondary semiconductors (ITO, Fe2TiO5) and OECs were combined to modify the donor density and surface states in the composite photoanodes, delivering 2.2 mA cm−2 at 1.23 V vs. RHE and 1 sun, which is 10 times higher than that of pristine hematite photoanodes. A deep and careful investigation into the mechanism responsible for the enhanced PEC performance of ITO/Fe2O3/Fe2TiO5/FeNiOOH multi-layer nanowires shows that: (i) the ITO underlayer is used as a high quality electrical back contact to reduce the back-contact interface charge recombination and as a Sn doping source for tuning the donor density, (ii) the atomic level Fe2TiO5 coating serves as the surface state density and energy level modulation layer, effectively suppressing the charge recombination at the semiconductor junction interface, (iii) the FeNiOOH nanodots increase the surface active sites, and thus accelerate the OER kinetics at the SEI. The interfacial coupling effect between the ITO underlayer, the ultrathin Fe2TiO5 layer and the FeNiOOH nanodots in the ITO/Fe2O3/Fe2TiO5/FeNiOOH photoanodes simultaneously enhances the charge separation and water oxidation efficiency.14 Most recently, we integrated Fe2O3 nanowires with an ultrathin Fe2TiO5 heterojunction and CoFe-Prussian blue analogue (PBA) decoration for enhanced PEC water splitting in an acidic electrolyte (pH = 1). Thanks to the combination of core–shell Fe2O3/Fe2TiO5 type II heterojunction nanowires and the catalytic function of CoFe–PBA, Fe2O3/Fe2TiO5/CoFe–PBA composite photoanodes are able to deliver 1.25 mA cm−2 photocurrent at 1.23 V vs. RHE, almost one order of magnitude photocurrent increment in comparison to the pristine Fe2O3 nanowires. A systematic electrochemical investigation reveals that the enhanced PEC performance of the Fe2O3/Fe2TiO5/CoFe–PBA composite electrode can be attributed to the modified surface state density after successive coatings.141
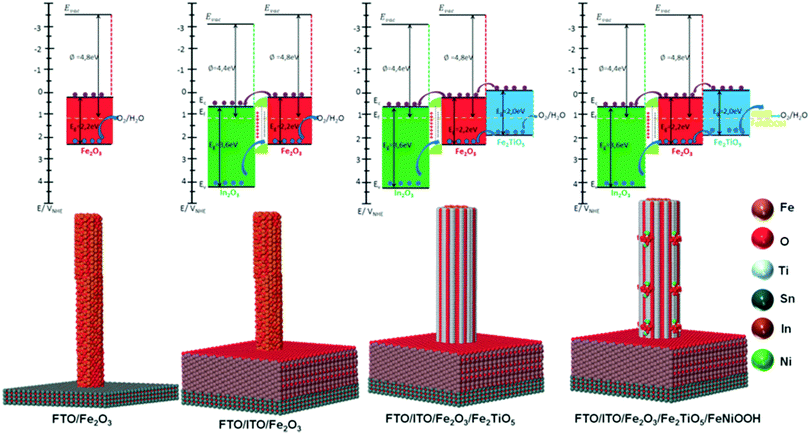 |
| Fig. 15 Top: Thermodynamic scheme for the charge transfer processes at 1.23 V vs. RHE of SEI under illumination for the Fe2O3, ITO/Fe2O3, ITO/Fe2O3/Fe2TiO5, and ITO/Fe2O3/Fe2TiO5/FeNiOOH electrodes. The black arrows indicate the bandgap of In2O3 (ITO matrix), hematite and Fe2TiO5. The dark blue arrow refers to the hole transfer process at the heterojunction interfaces. The purple arrow refers to the electron transfer process present at the heterojunction interfaces. For simplicity, interfacial charge transfer is considered to occur through the Ev and/or Ec states directly, without the intervention of interfacial SS. In all photoanodes, 4 electron–hole couples per visible active semiconductor are depicted. In2O3 (main ingredient of ITO matrix): green; Fe2O3: red; Fe2TiO5: indigo; FeNiOOH: yellow. Bottom: The atomic supercell models illustrate the interfaces existing at In2O3 (ITO matrix), hematite, Fe2TiO5 and FeNiOOH species in the integrated electrodes. Reproduced with permission from ref. 14. | |
5. Conclusions and prospects
Their earth abundant nature and excellent visible-light response endow hematite based photoanodes with promising photoelectrochemical water oxidation activity in the field of PEC water splitting. Studies centred on hematite based photoanodes will be beneficial for the future development of other photoelectrodes. Considerable work has been performed to control the composition, morphology, and nanostructure of hematite based photoanodes to improve their PEC water splitting performance. Although significant progress has been achieved, great efforts are still required to further explore the surface state mediated charge transfer mechanism of hematite based photoanodes for PEC water oxidation. In this review, we have summarized the recent progress in hematite photoanodes for PEC water splitting and highlighted the critical role of surface states in the development of high-performance hematite based photoanodes for PEC water splitting application. As a model system, the approach summarized here of hematite composite photoanodes might also be employed for the investigation of other surface state mediated photoelectrodes for PEC water splitting, including TiO2, BiVO4, CuWO4, GaN, CuFeO2, Ta3N5, and Cu(In,Ga)(Se,S)2.41–48,98,142,143 The following three aspects deserve special attention in the future investigations of hematite based photoanode systems in order to definitely improve their PEC efficiency:
(1) Typically, previous investigations attributed the enhanced PEC water splitting performance of hematite composite photoanodes either to the improved donor density or the changed surface states. Indeed, it is essential to simultaneously monitor the evolution of the donor density and surface states of hematite based photoanodes upon doping, type II heterojunction construction and OEC deposition.
(2) Previous studies on hematite based photoanodes have mainly focused on the strategy for the improvement of PEC water oxidation performance via the design of nanostructures, doping, heterojunction and OEC deposition. Few studies have reported the investigation about the mechanisms that are responsible for the enhanced PEC performance. Several previous mechanistic investigations have focused on simple hematite based photoanode systems. However, the main obstacle is to correlate the charge transfer efficiency at the SEI with the evolution of surface states upon coating with a secondary semiconductor or OEC. More hematite based composite photoanode systems should be extensively explored to overcome these obstacles.
(3) Most of the designing of composite hematite based photoanodes are limited to pristine or binary systems rather than ternary or quaternary composite photoelectrode systems. The integration of ternary or quaternary composite photoelectrodes with a secondary underlayer, overlayer and OEC is favourable for the improvement of the hematite-based photoanodes because the charge recombination simultaneously occurs at the different interfaces, including the back contact, bulk interfaces and SEI interfaces. Therefore, extending exploration of more complex hematite-based photoanodes might be of high interest.
(4) Various techniques have been employed to probe the nature of the surface states in hematite-based photoanode systems, including PEIS, CV, IMPS, IMVS, DRT, in situ UV-vis, in situ ATR-IR, 4D TEM, DFT, SECM and KIE. Nevertheless, the accurate observation of surface states/surface intermediates of hematite photoanodes with spatial, temporal and energy resolution is still limited. Further deep investigations about PEC water oxidation in hematite photoanodes will require emerging in situ techniques, like atomic force microscopy,144 single-molecule super-resolution fluorescence microscopy,78,145in situ (S)TEM,146 surface photovoltage microscopy (SPVM),147 on-line inductively coupled plasma mass spectrometry (ICP-MS),148 and dual-working-electrode photoelectrochemistry techniques.149–151
Conflicts of interest
There are no conflicts to declare.
Acknowledgements
The authors acknowledge funding from Generalitat de Catalunya 2017 SGR 327, the Spanish MINECO project ENE2017-85087-C3 and the BIST Ignite project InWOC2. ICN2 is supported by the Severo Ochoa program from Spanish MINECO (Grant No. SEV-2017-0706) and is funded by the CERCA Programme/Generalitat de Catalunya. Part of the present work was conducted in the framework of the Universitat Autònoma de Barcelona Materials Science PhD program.
References
- P. Zhang, T. Wang and J. L. Gong, Current mechanistic understanding of surface reactions over water-splitting photocatalysts, Chem, 2017, 4, 223–245 Search PubMed.
- G. Chen, J. Seo, C. Yang and P. N. Prasad, Nanochemistry and nanomaterials for photovoltaics, Chem. Soc. Rev., 2013, 42, 8304–8338 RSC.
- M. G. Walter, E. L. Warren, J. R. McKone, S. W. Boettcher, Q. Mi, E. A. Santori and N. S. Lewis, Solar water splitting cells, Chem. Rev., 2010, 110, 6446–6473 CrossRef CAS PubMed.
- N. S. Lewis and D. G. Nocera, Powering the planet: chemical challenges in solar energy utilization, Proc. Natl. Acad. Sci. U. S. A., 2006, 103, 15729–15735 CrossRef CAS PubMed.
- X. Chen, S. Shen, L. Guo and S. S. Mao, Semiconductor-based photocatalytic hydrogen generation, Chem. Rev., 2010, 110, 6503–6570 CrossRef CAS PubMed.
- F. Urbain, P. Y. Tang, N. M. Carretero, T. Andreu, L. G. Gerling, C. Voz, J. Arbiol and J. R. Morante, Energy Environ. Sci., 2017, 10, 2256–2266 RSC.
- A. Fujishima and K. Honda, Electrochemical photolysis of water at a semiconductor electrode, Nature, 1972, 238, 37–38 CrossRef CAS PubMed.
- A. J. Bard and M. A. Fox, Artificial photosynthesis: solar splitting of water to hydrogen and oxygen, Acc. Chem. Res., 1995, 28, 141–145 CrossRef CAS.
- N. S. Lewis, Toward cost-effective solar energy use, Science, 2007, 315, 798–801 CrossRef CAS PubMed.
- K. Sivula and R. V. D. Krol, Semiconducting materials for photoelectrochemical energy conversion, Nat. Rev. Mater., 2016, 1, 15010 CrossRef CAS.
- S. H. Shen, S. A. Lindley, X. Y. Chen and J. Z. Zhang, Hematite heterostructures for photoelectrochemical water splitting: rational materials design and charge carrier dynamics, Energy Environ. Sci., 2016, 9, 2744–2775 RSC.
- Z. Q. Huang, Y. J. Lin, X. Xiang, W. Rodríguez-Córdoba, K. J. McDonald, K. S. Hagen, K. S. Choi, B. S. Brunschwig, D. G. Musaev, C. L. Hill, D. W. Wang and T. Q. Lian, In situ probe of photocarrier dynamics in water-splitting hematite (α-Fe2O3) electrodes, Energy Environ. Sci., 2012, 5, 8923–8926 RSC.
- S. R. Pendlebury, A. J. Cowan, M. Barroso, K. Sivula, J. H. Ye, M. Grätzel, D. R. Klug, J. W. Tang and J. R. Durrant, Correlating long-lived photogenerated hole populations with photocurrent densities in hematite water oxidation photoanodes, Energy Environ. Sci., 2012, 5, 6304–6312 RSC.
- P. Y. Tang, H. B. Xie, C. Ros, L. J. Han, M. Biset-Peiró, Y. M. He, W. Kramer, A. Perez-Rodriguez, E. Saucedo, J. Galan-Mascaros, T. Andreu, J. R. Morante and J. Arbiol, Enhanced photoelectrochemical water splitting of hematite multilayer nanowires photoanode with tuning surface state via bottom-up interfacial engineering, Energy Environ. Sci., 2017, 10, 2124–2136 RSC.
- A. G. Tamirat, J. Rick, A. A. Dubale, W. N. Su and B. J. Hwang, Using hematite for photoelectrochemical water splitting: a review of current progress and challenges, Nanoscale Horiz., 2016, 1, 243–267 RSC.
- M. Y. Li, Y. Y. Qiu, W. T. Qiu, S. T. Tang, S. Xiao, S. L. Ma, G. F. Ouyang, Y. X. Tong and S. H. Yang, Boosting the photoelectrochemical water oxidation at hematite photoanode by innovating a hierarchical ball-on-wire-array, ACS Appl. Energy Mater., 2018, 1, 5836–5841 CrossRef.
- L. Wang, N. T. Nguyen, Z. Q. Shen, P. Schmuki and Y. P. Bi, Hematite dodecahedron crystals with high-index facets grown and grafted on one dimensional structures for efficient photoelectrochemical H2 generation, Nano Energy, 2018, 50, 331–338 CrossRef CAS.
- H. M. Zhang, Y. K. Kim, H. Y. Jeong and J. S. Lee, A few atomic FeNbO4 overlayers on hematite nanorods: Microwave-induced high temperature phase for efficient photoelectrochemical water splitting, ACS Catal., 2019, 9, 1289–1297 CrossRef CAS.
- A. Annamalai, P. S. Shinde, A. Subramanian, J. Y. Kim, J. H. Kim, S. H. Choi, J. S. Lee and J. S. Jang, Bifunctional TiO2 underlayer for a-Fe2O3 nanorod based photoelectrochemical cells: enhanced interface and Ti4+ doping, J. Mater. Chem. A, 2015, 3, 5007–5013 RSC.
- P. Zhang, L. Yu and X. W. Lou, Construction of heterostructured Fe2O3-TiO2 microdumbbells for photoelectrochemical water oxidation, Angew. Chem., 2018, 130, 15296–15300 CrossRef.
- Z. B. Luo, T. Wang, J. J. Zhang, C. C. Li, H. M. Li and J. L. Gong, Dendritic hematite nanoarray photoanode modified with a conformal titanium dioxide interlayer for effective charge collection, Angew. Chem., Int. Ed., 2017, 56, 12878–12882 CrossRef CAS PubMed.
- A. Tsyganok, D. Klotz, K. D. Malviya, A. Rothschild and D. A. Grave, Different roles of Fe1−xNixOOH cocatalyst on hematite (α-Fe2O3) photoanodes with different dopants, ACS Catal., 2018, 8, 2754–2759 CrossRef CAS.
- B. Eftekharinia, A. Moshaii, N. S. Vayghan and A. Dabirian, Efficient nanoporous hematite photoanodes prepared by electron beam evaporation and Au modification, ChemCatChem, 2018, 10, 4665–4675 CrossRef CAS.
- J. J. Deng, Q. Z. Zhang, K. Feng, H. W. Lan, J. Zhong, M. Chaker and D. L. Ma, ChemSusChem, 2018, 11, 3783–3789 CrossRef CAS PubMed.
- S. H. Shen, S. A. Lindley, C. L. Dong, E. F. Chen, Y. R. Lu, J. G. Zhou, Y. F. Hu, D. A. Wheeler, P. H. Guo, J. Z. Zhang, D. S. Kliger and S. S. Mao, Sol. RRL, 2019, 3, 1800285 Search PubMed.
- S. S. Yi, B. R. Wulan, J. M. Yan and Q. Jiang, Highly efficient photoelectrochemical water splitting: Surface modification of cobalt-phosphate-loaded Co3O4/Fe2O3 p-n heterojunction nanorod arrays, Adv. Funct. Mater., 2019, 29, 1801902 CrossRef.
- Y. Choi, D. Jeon, Y. Choi, D. Kim, N. Kim, M. S. Gu, S. Bae, T. Lee, H. W. Lee, B. S. Kim and J. Ryu, Interface engineering of hematite with nacre-like catalytic multilayers for solar water oxidation, ACS Nano, 2019, 13, 467–475 CrossRef CAS PubMed.
- J. J. Zhao, Y. H. Li, P. F. Liu, Y. L. Wang, X. L. Du, X. L. Wang, H. D. Zeng, L. R. Zheng and H. G. Yang, Local coulomb attraction for enhanced H2 evolution stability of metal sulfide photocatalysts, Appl. Catal., B, 2018, 221, 152–157 CrossRef CAS.
- S. M. Ho-Kimura, B. A. D. Williamson, S. Sathasivam, S. J. A. Moniz, G. J. He, W. J. Luo, D. O. Scanlon, J. W. Tang and I. P. Parkin, ACS Omega, 2019, 4, 1449–1459 CrossRef CAS.
- J. J. Deng, X. X. Lv and J. Zhong, Photocharged Fe2TiO5/Fe2O3 photoanode for enhanced photoelectrochemical water oxidation, J. Phys. Chem. C, 2018, 122, 29268–29273 CrossRef CAS.
- P. Peerakiatkhajohn, J. H. Yun, H. J. Chen, M. Q. Lyu, T. Butburee and L. Z. Wang, Adv. Mater., 2016, 28, 6405–6410 CrossRef CAS PubMed.
- C. C. Li, A. Li, Z. B. Luo, J. J. Zhang, X. X. Chang, Z. Q. Huang, T. Wang and J. L. Gong, Surviving high-temperature calcination: ZrO2-induced hematite nanotubes for photoelectrochemical water oxidation, Angew. Chem., Int. Ed., 2017, 56, 4150–4155 CrossRef CAS PubMed.
- M. P. Cardona, M. Y. Li, W. Li, J. McCall, D. W. Wang, Y. Li and C. Yang, The role of graphene as an overlayer on nanostructured hematite photoanodes for improved solar water oxidation, Mater. Today Energy, 2018, 8, 8–14 CrossRef.
- H. M. Zhang, W. Y. Noh, F. Li, J. H. Kim, H. Y. Jeong and J. S. Lee, Three birds, one-stone strategy for hybrid microwave synthesis of Ta and Sn codoped Fe2O3@FeTaO4 nanorods for photo-electrochemical water oxidation, Adv. Funct. Mater., 2019, 29, 1805737 CrossRef.
- H. Lin, X. Long, Y. M. An, D. Zhou and S. H. Yang, Three-dimensional decoupling Co-catalyst from a photoabsorbing semiconductor as a new strategy to boost photoelectrochemical water splitting, Nano Lett., 2019, 19, 455–460 CrossRef CAS PubMed.
- S. D. Tilley, M. Cornuz, K. Sivula and M. Grätzel, Light-induced water splitting with hematite: Improved nanostructure and iridium oxide catalysis, Angew. Chem., Int. Ed., 2010, 49, 6405–6408 CrossRef CAS PubMed.
- F. L. Formal, N. Tétreault, M. Cornuz, T. Moehl, M. Grätzel and K. Sivula, Passivating surface states on water splitting hematite photoanodes with alumina overlayers, Chem. Sci., 2011, 2, 737–743 RSC.
- J. A. Turner, A realizable renewable energy future, Science, 1999, 285, 687–689 CrossRef CAS PubMed.
- J. R. Bolton, S. J. Strickler and J. S. Connolly, Limiting and realizable efficiencies of solar photolysis of water, Nature, 1985, 316, 495–500 CrossRef CAS.
-
S. Giménez and J. Bisquert, Photoelectrochemical solar fuel production from basic principles to advanced devices, Photoelectrochemical solar fuel production, Springer, 2016 Search PubMed.
- J. J. Kelly and R. Memming, The influence of surface recombination and trapping on the cathodic photocurrent at p-type III–V electrodes, J. Electrochem. Soc., 1982, 129, 730–738 CrossRef CAS.
- M. P. Dareedwards, J. B. Goodenough, A. Hamnett and P. R. Trevellick, Electrochemistry and photoelectrochemistry of Iron(III) oxide, J. Chem. Soc., Faraday Trans. 1, 1983, 79, 2027–2041 RSC.
- Q. Shi, S. Murcia-López, P. Y. Tang, C. Flox, J. R. Morante, Z. Y. Bian, H. Wang and T. Andreu, Role of tungsten doping on the surface states in BiVO4 photoanodes for water oxidation: Tuning the electron trapping process, ACS Catal., 2018, 8, 3331–3342 CrossRef CAS.
- Y. Gao and T. W. Hamann, Elucidation of CuWO4 surface states during photoelectrochemical water oxidation, J. Phys. Chem. Lett., 2017, 8, 2700–2704 CrossRef CAS PubMed.
- P. Varadhan, H. C. Fu, D. Priante, J. R. D. Retamal, C. Zhao, M. Ebaid, T. K. Ng, I. Ajia, S. Mitra, I. S. Roqan, B. Ooi and J. H. He, Surface passivation of GaN nanowires for enhanced photoelectrochemical water-splitting, Nano Lett., 2017, 17, 1520–1528 CrossRef CAS PubMed.
- M. S. Prévot, X. A. Jeanbourquin, W. S. Bourée, F. Abdi, D. Friedrich, R. V. Krol, N. Guijarro, F. L. Formal and K. Sivula, Evaluating charge carrier transport and surface states in CuFeO2 photocathodes, Chem. Mater., 2017, 29, 4952–4962 CrossRef.
- Y. M. He, J. E. Thorne, C. H. Wu, P. Y. Ma, C. Du, Q. Dong, J. H. Guo and D. W. Wang, What limits the performance of Ta3N5 for solar water splitting?, Chem, 2016, 1, 640–655 CAS.
- S. Y. Chae, S. J. Park, S. G. Han, H. Jung, C. W. Kim, C. Jeong, O. S. Joo, B. K. Min and Y. J. Hwang, J. Am. Chem. Soc., 2016, 138, 15673–15681 CrossRef CAS PubMed.
- P. Salvador and C. Gutierrez, The nature of surface states involved in the photo- and electroluminescence spectra of n-titanium dioxide electrodes, J. Phys. Chem., 1984, 88, 3696–3698 CrossRef CAS.
- P. Salvador and C. Gutierrez, Mechanisms of charge transfer at the semiconductor-electrolyte interface, J. Electrochem. Soc., 1984, 131, 326–336 CrossRef CAS.
- L. Bertoluzzi, P. L. Varo, J. A. J. Tejada and J. Bisquert, Charge transfer processes at the semiconductor/electrolyte interface for solar fuel production: insight from impedance spectroscopy, J. Mater. Chem. A, 2016, 4, 2873–2879 RSC.
- J. Bisquert, Theory of the impedance of charge transfer via surface states in dye-sensitized solar cells, J. Electroanal. Chem., 2010, 646, 43–51 CrossRef CAS.
- K. G. U. Wijayantha, S. Saremi-Yarahmadi and L. M. Peter, Kinetics of oxygen evolution at α-Fe2O3 photoanodes: a study by photoelectrochemical impedance spectroscopy, Phys. Chem. Chem. Phys., 2011, 13, 5264–5270 RSC.
- B. Klahr, S. Gimenez, F. Fabregat-Santiago, T. Hamann and J. Bisquert, Water oxidation at hematite photoelectrodes: The role of surface states, J. Am. Chem. Soc., 2012, 134, 4294–4302 CrossRef CAS PubMed.
- B. Klahr, S. Gimenez, F. Fabregat-Santiago, J. Bisquert and T. W. Hamann, Electrochemical and photoelectrochemical investigation of water oxidation with hematite electrodes, Energy Environ. Sci., 2012, 5, 7626–7636 RSC.
- L. Bertoluzzi and J. Bisquert, Equivalent circuit of electrons and holes in thin semiconductor films for photoelectrochemical water splitting applications, J. Phys. Chem. Lett., 2012, 3, 2517–2522 CrossRef CAS PubMed.
- T. Lopes, L. Andrade, F. L. Formal, M. Gratzel, K. Sivula and A. Mendes, Phys. Chem. Chem. Phys., 2014, 16, 16515–16523 RSC.
- O. Zandi, A. R. Schon, H. Hajibabaei and T. W. Hamann, Enhanced charge separation and collection in high-performance electrodeposited hematite films, Chem. Mater., 2016, 28, 765–771 CrossRef CAS.
- C. Y. Cummings, F. Marken, L. M. Peter, K. G. U. Wijayantha and A. A. Tahir, New Insights into Water Splitting at Mesoporous α-Fe2O3 Films: A Study by Modulated Transmittance and Impedance Spectroscopies, J. Am. Chem. Soc., 2011, 134, 1228–1234 CrossRef PubMed.
- J. Reichman, The current-voltage characteristics of semiconductor-electrolyte junction photovoltaic cells, Appl. Phys. Lett., 1980, 36, 574–577 CrossRef CAS.
- J. Bisquert, Theory of the impedance of charge transfer via surface states in dye-sensitized solar cells, J. Electroanal. Chem., 2010, 646, 43–51 CrossRef CAS.
- E. A. Ponomarev and L. M. Peter, A generalized theory of intensity modulated photocurrent spectroscopy (IMPS), J. Electroanal. Chem., 1995, 396, 219–226 CrossRef.
- C. Y. Cummings, F. Marken, L. M. Peter, A. A. Tahir and K. G. U. Wijayantha, Kinetics and mechanism of light-driven oxygen evolution at thin film α-Fe2O3 electrodes, Chem. Commun., 2012, 48, 2027–2029 RSC.
- L. M. Peter, Energetics and kinetics of light-driven oxygen evolution at semiconductor electrodes: the example of hematite, J. Solid State Electrochem., 2013, 17, 315–326 CrossRef CAS.
- H. K. Dunn, J. M. Feckl, A. Muller, D. Fattakhova-Rohlfing, S. G. Morehead, J. Roos, L. M. Peter, C. Scheu and T. Bein, Tin doping speeds up hole transfer during light-driven water oxidation at hematite photoanodes, Phys. Chem. Chem. Phys., 2014, 16, 24610–24620 RSC.
- J.
E. Thorne, J. W. Jang, E. Y. Liu and D. W. Wang, Understanding the origin of photoelectrode performance enhancement by probing surface kinetics, Chem. Sci., 2016, 7, 3347–3354 RSC.
- D. Klotz, D. S. Ellis, H. Dotan and A. Rothschild, Empirical in operando analysis of the charge carrier dynamics in hematite photoanodes by PEIS, IMPS and IMVS, Phys. Chem. Chem. Phys., 2016, 18, 23438–23457 RSC.
- D. Klotz, D. A. Grave, H. Dotan and A. Rothschild, Empirical analysis of the photoelectrochemical impedance response of hematite photoanodes for water photo-oxidation, J. Phys. Chem. Lett., 2018, 9, 1466–1472 CrossRef CAS PubMed.
- Y. Y. Avital, H. Dotan, D. Klotz, D. A. Grave, A. Tsyganok, B. Gupta, S. Kolusheva, I. Visoly-Fisher1, A. Rothschild and A. Yochelis, Two-site H2O2 photo-oxidation on haematite photoanodes, Nat. Commun., 2018, 9, 4060 CrossRef PubMed.
- B. Klahr and T. Hamann, Water oxidation on hematite photoelectrodes: insight into the nature of surface states through in situ spectroelectrochemistry, J. Phys. Chem. C, 2014, 118, 10393–10399 CrossRef CAS.
- T. Takashima, K. Ishikawa and H. Irie, Detection of Intermediate Species in Oxygen Evolution on Hematite Electrodes Using Spectroelectrochemical Measurements, J. Phys. Chem. C, 2016, 120, 24827–24834 CrossRef CAS.
- O. Zandi and T. W. Hamann, Determination of photoelectrochemical water oxidation intermediates on haematite electrode surfaces using operando infrared spectroscopy, Nat. Chem., 2016, 8, 778–783 CrossRef CAS PubMed.
- J. P. Bigi, W. H. Harman, B. Lassalle-Kaiser, D. M. Robles, T. A. Stich, J. Yano, R. D. Britt and C. J. Chang, A high-spin iron(IV)-oxo complex supported by a trigonal nonheme pyrrolide platform, J. Am. Chem. Soc., 2012, 134, 1536–1542 CrossRef CAS PubMed.
- A. R. McDonald and L. Que, High-valent nonheme iron-oxo complexes: synthesis, structure, and spectroscopy, Coord. Chem. Rev., 2013, 257, 414–428 CrossRef CAS.
- H. Shi, J. A. Lercher and X. Y. Yu, Sailing into uncharted waters: recent advances in the in-situ monitoring of catalytic processes in aqueous environments, Catal. Sci. Technol., 2015, 5, 3035–3060 RSC.
- Y. C. Zhang, H. N. Zhang, A. N. Liu, C. C. Chen, W. J. Song and J. C. Zhao, Rate-limiting O-O bond formation pathways for water oxidation on hematite photoanode, J. Am. Chem. Soc., 2018, 140, 3264–3269 CrossRef CAS PubMed.
- Z. X. Su, J. S. Baskin, W. Z. Zhou, J. M. Thomas and A. H. Zewail, Ultrafast elemental and oxidation-state mapping of hematite by 4D electron microscopy, J. Am. Chem. Soc., 2017, 139, 4916–4922 CrossRef CAS PubMed.
- J. B. Sambur, T. Y. Chen, E. Choudhary, G. Q. Chen, E. J. Nissen, E. M. Thomas, N. M. Zou and P. Chen, Sub-particle reaction and photocurrent mapping to optimize catalyst-modified photoanodes, Nature, 2016, 530, 77–80 CrossRef CAS PubMed.
- B. Iandolo and A. Hellman, The role of surface states in the oxygen evolution reaction on hematite, Angew. Chem., Int. Ed., 2014, 53, 13404–13408 CrossRef CAS PubMed.
- N. Yatom, O. Neufeld and M. C. Toroker, Toward settling the debate on the role of Fe2O3 surface states for water splitting, J. Phys. Chem. C, 2015, 119, 24789–24795 CrossRef CAS.
- J. Noh, H. Li, O. I. Osman, S. G. Aziz, P. Winget and J. L. Brédas, Impact of hydroxylation and hydration on the reactivity of α-Fe2O3 (0001) and (1-102) surfaces under environmental and electrochemical conditions, Adv. Energy Mater., 2018, 1800545 CrossRef.
- H. Li, M. S. Du, M. J. Mleczko, A. L. Koh, Y. Nishi, E. Pop, A. J. Bard and X. L. Zheng, Kinetic study of hydrogen evolution reaction over strained MoS2 with sulphur-vacancies using scanning electrochemical microscopy, J. Am. Chem. Soc., 2016, 138, 5123–5129 CrossRef CAS PubMed.
- J. Y. Kim, H. S. Ahn and A. J. Bard, Surface interrogation scanning electrochemical microscopy for a photoelectrochemical reaction-water oxidation on a hematite surface, Anal. Chem., 2018, 90, 3045–3049 CrossRef CAS PubMed.
- M. R. Krumov, B. H. Simpson, M. J. Counihan and J. Rodriguez-Lopez, In situ quantification of surface intermediates and correlation to discharge products on hematite photoanodes using a combined scanning electrochemical microscopy approach, Anal. Chem., 2018, 90, 3050–3057 CrossRef CAS PubMed.
- Y. C. Zhang, H. N. Zhang, H. W. Ji, W. H. Ma, C. C. Chen and J. C. Zhao, Pivotal role and regulation of proton transfer in water oxidation on hematite photoanodes, J. Am. Chem. Soc., 2016, 138, 2705–2711 CrossRef CAS PubMed.
- S. Haschke, M. Mader, S. Schlicht, A. M. Roberts, A. M. Angeles-Boza, J. A. C. Barth and J. Bachmann, Direct oxygen isotope effect identifies the rate-determining step of electrocatalytic OER at an oxidic surface, Nat. Commun., 2018, 9, 4565 CrossRef PubMed.
- R. Morrish, M. Rahman, J. M. Don MacElroy and C. A. Wolden, Activation of hematite nanorod arrays for photoelectrochemical water splitting, ChemSusChem, 2011, 4, 474–479 CrossRef CAS PubMed.
- P. S. Shinde, A. Annamalai, J. H. Kim, S. H. Choi, J. S. Lee and J. S. Jang, Exploiting the dynamic Sn diffusion from deformation of FTO to boost the photocurrent performance of hematite photoanodes, Sol. Energy Mater. Sol. Cells, 2015, 141, 71–79 CrossRef CAS.
- A. Annamalai, A. Subramanian, U. Kang, H. Park, S. H. Choi and J. S. Jang, Activation of hematite photoanodes for solar water splitting: Effect of FTO deformation, J. Phys. Chem. C, 2015, 119, 3810–3817 CrossRef CAS.
- O. Zandi and T. W. Hamann, Enhanced Water Splitting Efficiency Through selective surface state removal, J. Phys. Chem. Lett., 2014, 5, 1522–1526 CrossRef CAS PubMed.
- P. S. Shinde, S. H. Choi, Y. Kim, J. Ryu and J. S. Jang, Onset potential behavior in a-Fe2O3 photoanodes: the influence of surface and diffusion Sn doping on the surface states, Phys. Chem. Chem. Phys., 2016, 18, 2495–2509 RSC.
- Y. Li and H. Chen, Facile fire treatment of nanostructured hematite with an enhanced photoelectrochemical water splitting performance, J. Mater. Chem. A, 2016, 4, 14974–14977 RSC.
- J. R. Xiao, H. L. Huang, Q. Y. Huang, L. Zhao, X. Li, X. L. Hou, H. Chen and Y. D. Li, Suppressing the electron-hole recombination rate in hematite photoanode with a rapid cooling treatment, J. Catal., 2017, 350, 48–55 CrossRef CAS.
- M. Pyeon, T. P. Ruoko, J. Leduc, Y. Gönüllü, M. Deo, N. V. Tkachenko and S. Mathur, Critical role and modification of surface states in hematite films for enhancing oxygen evolution activity, J. Mater. Res., 2018, 33, 455–466 CrossRef CAS.
- Y. C. Ling, G. M. Wang, J. Reddy, C. C. Wang, J. Z. Zhang and Y. Li, The influence of oxygen content on the thermal activation of hematite nanowires, Angew. Chem., Int. Ed., 2012, 51, 4074–4079 CrossRef CAS PubMed.
- M. Forster, R. J. Potter, Y. C. Ling, Y. Yang, D. R. Klug, Y. Li and A. J. Cowan, Chem. Sci., 2015, 6, 4009–4016 RSC.
- Z. L. Wang, X. Mao, P. Chen, M. Xiao, S. A. Monny, S. C. Wang, M. Konarova, A. J. Du and L. Z. Wang, Understanding the roles of oxygen vacancies in hematite-based photoelectrochemical processes, Angew. Chem., 2019, 131, 1042–1046 CrossRef.
- X. B. Chen, L. Liu, P. Y. Yu and S. S. Mao, Increasing solar absorption for photocatalysis
with black hydrogenated titanium dioxide nanocrystals, Science, 2011, 331, 746–750 CrossRef CAS PubMed.
- J. Moir, N. Soheilnia, K. Liao, P. O’Brien, Y. Tian, K. S. Burch and G. A. Ozin, Activation of ultrathin films of hematite for photoelectrochemical water splitting via H2 treatment, ChemSusChem, 2015, 8, 1557–1567 CrossRef CAS PubMed.
- M. Li, J. J. Deng, A. W. Pu, P. P. Zhang, H. Zhang, J. Gao, Y. Y. Hao, J. Zhong and X. H. Sun, Hydrogen-treated hematite nanostructures with low onset potential for highly efficient solar water oxidation, J. Mater. Chem. A, 2014, 2, 6727–6733 RSC.
- Y. Yang, M. Forster, Y. C. Ling, G. M. Wang, T. Zhai, Y. X. Tong, A. J. Cowan and Y. Li, Acid treatment enables suppression of electron-hole recombination in hematite for photoelectrochemical water splitting, Angew. Chem., Int. Ed., 2016, 55, 3403–3407 CrossRef CAS PubMed.
- X. L. Zhang, X. Wang, X. L. Yi, J. H. Ye and D. F. Wang, Alkali treatment for enhanced photoelectrochemical water oxidation on hematite photoanode, ACS Sustainable Chem. Eng., 2019, 7, 5420–5429 CrossRef CAS.
- Q. N. Wu, D. D. Meng, Y. Zhang, Q. D. Zhao, Q. J. Bu, D. J. Wang, X. X. Zou, Y. H. Lin, S. Li and T. F. Xie, Acid-treated Ti4+ doped hematite photoanode for efficient solar water oxidationd Insight into surface states and charge separation, J. Alloys Compd., 2019, 782, 943–951 CrossRef CAS.
- J. W. Jang, C. Du, Y. F. Ye, Y. J. Lin, X. H. Yao, J. Thorne, E. Liu, G. McMahon, J. F. Zhu, A. Javey, J. H. Guo and D. W. Wang, Enabling unassisted solar water splitting by iron oxide and silicon, Nat. Commun., 2015, 6, 7447 CrossRef PubMed.
- G. Y. Liu, S. K. Karuturi, H. J. Chen, L. Spiccia, H. H. Tan, C. Jagadish, D. W. Wang, A. N. Simonov and A. Tricoli, Tuning the morphology and structure of disordered hematite photoanodes for improved water oxidation: A physical and chemical synergistic approach, Nano Energy, 2018, 53, 745–752 CrossRef CAS.
- F. L. Formal, N. Tetreault, M. Cornuz, T. Moehl, M. Gratzel and K. Sivula, Passivating surface states on water splitting hematite photoanodes with alumina overlayers, Chem. Sci., 2011, 2, 737–743 RSC.
- L. Steier, I. Herraiz-Cardona, S. Gimenez, F. Fabregat-Santiago, J. Bisquert, S. D. Tilley and M. Grätzel, Understanding the role of underlayers and overlayers in thin film hematite photoanodes, Adv. Funct. Mater., 2014, 24, 7681 CrossRef CAS.
- A. Mettenbörger, Y. Gönüllü, T. Fischer, T. Heisig, A. Sasinska, C. Maccato, G. Carraro, C. Sada, D. Barreca, L. Mayrhofer, M. Moseler, A. Held and S. Mathur, Interfacial insight in multi-junction metal oxide photoanodes for water-splitting applications, Nano Energy, 2016, 19, 415–427 CrossRef.
- P. Zhang, T. Wang, X. X. Chang, L. Zhang and J. L. Gong, Synergistic cocatalytic effect of carbon nanodots and Co3O4 nanoclusters for the photoelectrochemical water oxidation on hematite, Angew. Chem., Int. Ed., 2016, 55, 5851–5855 CrossRef CAS PubMed.
- M. Forster, R. J. Potter, Y. Yang, Y. Li and A. J. Cowan, Stable Ta2O5 overlayers on hematite for enhanced photoelectrochemical water splitting efficiencies, ChemPhotoChem, 2018, 2, 183–189 CrossRef CAS.
- H. J. Ahn, K. Y. Yoon, M. J. Kwak, J. Park and J. H. Jang, Boron doping of metal-doped hematite for reduced surface recombination in water splitting, ACS Catal., 2018, 8, 11932–11939 CrossRef CAS.
- T. Hisatomi, F. L. Formal, M. Cornuz, J. Brillet, N. Tétreault, K. Sivula and M. Grätzel, Cathodic shift in onset potential of solar oxygen evolution on hematite by 13-group oxide overlayers, Energy Environ. Sci., 2011, 4, 2512–2515 RSC.
- Y. C. Ling, G. M. Wang, D. A. Wheeler, J. Z. Zhang and Y. Li, Sn-doped hematite nanostructures for photoelectrochemical water splitting, Nano Lett., 2011, 11, 2119–2125 CrossRef CAS PubMed.
- M. A. Mahadik, A. Subramanian, J. H. Ryu, M. Cho and J. S. Jang, A hydrothermally grown CdS nanograin-sensitized 1D Zr:α-Fe2O3/FTO photoanode for efficient solar-light-driven photoelectrochemical performance, Dalton Trans., 2017, 46, 2377–2386 RSC.
- M. Y. Li, Y. Yang, Y. C. Ling, W. T. Qiu, F. X. Wang, T. Y. Liu, Y. Song, X. X. Liu, P. P. Fang, Y. X. Tong and Y. Li, Morphology and doping engineering of Sn-doped hematite nanowire photoanodes, Nano Lett., 2017, 17, 2490–2495 CrossRef CAS PubMed.
- C. Lohaus, A. Klein and W. Jaegermann, Limitation of Fermi level shifts by polaron defect states in hematite photoelectrodes, Nat. Commun., 2018, 9, 4309 CrossRef PubMed.
- D. Monllor-Satoca, M. Bartsch, C. Fabrega, A. Genç, S. Reinhard, T. Andreu, J. Arbiol, M. Niederberger and J. R. Morante, What do you do, titanium? Insight into the role of titanium oxide as a water oxidation promoter in hematite-based photoanodes, Energy Environ. Sci., 2015, 8, 3242–3254 RSC.
- Z. L. Wang, F. T. Fan, S. Y. Wang, C. M. Ding, Y. L. Zhao and C. Li, Bridging surface states and current-potential response over hematite-based photoelectrochemical water oxidation, RSC Adv., 2016, 6, 85582–85586 RSC.
- P. S. Bassi, R. P. Antony, P. P. Boix, Y. N. Fang, J. Barber and L. H. Wong, Crystalline Fe2O3/Fe2TiO5 heterojunction nanorods with efficient charge separation and hole injection as photoanode for solar water oxidation, Nano Energy, 2016, 22, 310–318 CrossRef CAS.
- O. Zandi, B. M. Klahr and T. W. Hamann, Highly photoactive Ti-doped a-Fe2O3 thin film electrodes: resurrection of the dead layer, Energy Environ. Sci., 2013, 6, 634–642 RSC.
- F. Li, J. Li, J. Zhang, L. L. Gao, X. F. Long, Y. P. Hu, S. W. Li, J. Jin and J. T. Ma, NiO nanoparticles anchored on phosphorus-doped a-Fe2O3 nanoarrays: An efficient hole extraction p-n heterojunction photoanode for water oxidation, ChemSusChem, 2018, 11, 2156–2164 CrossRef CAS PubMed.
- L. P. Gong, J. L. Xie, X. R. Liang, J. Y. Xiong, S. L. Yi, X. Y. Zhang and C. M. Li, Tailoring surface states by sequential doping of Ti and Mg for kinetically enhanced hematite photoanode, J. Colloid Interface Sci., 2019, 542, 441–450 CrossRef CAS PubMed.
- J. W. Park, M. A. Mahadik, H. Q. Ma, G. W. An, H. H. Lee, S. H. Choi, W. S. Chae, H. S. Chung and J. S. Jang, Improved interfacial charge transfer dynamics and onset shift in nanostructured hematite photoanodes via efficient Ti4+/Sn4+ heterogeneous self-doping through controlled TiO2 underlayers, ACS Sustainable Chem. Eng., 2019, 7, 6947–6958 CrossRef CAS.
- M. A. Mahadik, A. Subramanian, H. S. Chung, M. Cho and J. S. Jang, CdS/Zr:Fe2O3 nanorod arrays with Al2O3 passivation layer for photoelectrochemical solar hydrogen generation, ChemSusChem, 2017, 10, 2030–2039 CrossRef CAS PubMed.
- F. Li, J. Li, L. L. Gao, Y. P. Hu, X. F. Long, S. Q. Wei, C. L. Wang, J. Jin and J. T. Ma, Construction of an efficient hole migration pathway on hematite for efficient photoelectrochemical water oxidation, J. Mater. Chem. A, 2018, 6, 23478–23485 RSC.
- S. Chen, J. H. Li, J. Bai, L. G. Xia, Y. Zhang, L. S. Li, Q. J. Xu and B. X. Zhou, Electron blocking and hole extraction by a dual-function layer for hematite with enhanced photoelectrocatalytic performance, Appl. Catal., B, 2018, 237, 175–184 CrossRef CAS.
- A. G. Tamirat, A. A. Dubale, W. N. Su, H. M. Chen and B. J. Hwang, Sequentially surface modified hematite enables lower applied bias photoelectrochemical water splitting, Phys. Chem. Chem. Phys., 2017, 19, 20881–20890 RSC.
- A. Y. Ahmed, M. G. Ahmed and T. A. Kandiel, Hematite photoanodes with size-controlled nanoparticles for enhanced photoelectrochemical water oxidation, Appl. Catal., B, 2018, 236, 117–124 CrossRef CAS.
- J. W. Moir, E. V. Sackville, U. Hintermair and G. A. Ozin, Kinetics versus charge separation: Improving the activity of stoichiometric and non-stoichiometric hematite photoanodes using a molecular iridium water oxidation catalyst, J. Phys. Chem. C, 2016, 120, 12999–13012 CrossRef CAS.
- S. C. Riha, B. M. Klahr, E. C. Tyo, S. Seifert, S. Vajda, M. J. Pellin, T. W. Hamann and A. B. F. Martinson, Atomic layer deposition of a submonolayer catalyst for the enhanced photoelectrochemical performance of water oxidation with hematite, ACS Nano, 2013, 7, 2396–2405 CrossRef CAS PubMed.
- B. Klahr, S. Gimenez, F. Fabregat-Santiago, J. Bisquert and T. W. Hamann, Photoelectrochemical and impedance spectroscopic investigation of water oxidation with “Co-Pi”-coated hematite electrodes, J. Am. Chem. Soc., 2012, 134, 16693–16700 CrossRef CAS PubMed.
- G. M. Carroll and D. R. Gamelin, Kinetic analysis of photoelectrochemical water oxidation by mesostructured Co-Pi/a-Fe2O3 photoanodes, J. Mater. Chem. A, 2016, 4, 2986–2994 RSC.
- W. Li, S. W. Sheehan, D. He, Y. M. He, X. H. Yao, R. L. Grimm, G. W. Brudvig and D. W. Wang, Hematite-based solar water splitting in acidic solutions: Functionalization by mono- and multilayers of iridium oxygen-evolution catalysts, Angew. Chem., 2015, 127, 11590–11594 CrossRef.
- W. Li, D. He, S. W. Sheehan, Y. M. He, J. E. Thorne, X. H. Yao, G. W. Brudvig and D. W. Wang, Comparison of heterogenized molecular and heterogeneous oxide catalysts for photoelectrochemical water oxidation, Energy Environ. Sci., 2016, 9, 1794–1802 RSC.
- Y. Y. Zhao, K. R. Yang, Z. C. Wang, X. X. Yan, S. F. Cao, Y. F. Ye, Q. Dong, X. Z. Zhang, J. E. Thorne, L. Jin, K. L. Materna, A. Trimpalis, H. Y. Bai, S. C. Fakra, X. Y. Zhong, P. Wang, X. Q. Pan, J. H. Guo, M. Flytzani-Stephanopoulos, G. W. Brudvig, V. S. Batista and D. W. Wang, Stable iridium dinuclear heterogeneous catalysts supported on metal-oxide substrate for solar water oxidation, Proc. Natl. Acad. Sci. U. S. A., 2018, 115, 2902–2907 CrossRef CAS PubMed.
- C. H. Cui, M. Heggen, W. D. Zabka, W. Cui, J. Osterwalder, B. Probst and R. Alberto, Atomically dispersed hybrid nickel-iridium sites for photoelectrocatalysis, Nat. Commun., 2017, 8, 1341 CrossRef PubMed.
- J. F. Zhang, R. Garcia-Rodriguez, P. Cameron and S. Eslava, Role of cobalt-iron (oxy)hydroxide (CoFeOx) as oxygen evolution catalyst on hematite photoanodes, Energy Environ. Sci., 2018, 11, 2972–2984 RSC.
- D. K. Zhong, M. Cornuz, K. Sivula, M. Gratzel and D. R. Gamelin, Photo-assisted electrodeposition of cobalt-phosphate (Co-Pi) catalyst on hematite photoanodes for solar water oxidation, Energy Environ. Sci., 2011, 4, 1759–1764 RSC.
- J. Y. Kim, D. H. Youn, K. Kang and J. S. Lee, Highly conformal deposition of an ultrathin FeOOH layer on a hematite nanostructure for efficient solar water splitting, Angew. Chem., Int. Ed., 2016, 55, 10854–10858 CrossRef CAS PubMed.
- I. S. Cho, H. S. Han, M. Logar, J. Park and X. L. Zheng, Enhancing low-bias performance of hematite photoanodes for solar water splitting by simultaneous reduction of bulk, interface, and surface recombination pathways, Adv. Energy Mater., 2016, 6, 1501840 CrossRef.
- P. Y. Tang, L. J. Han, F. S. Hegner, P. Paciok, M. Biset-Peiró, H. C. Du, X. K. Wei, L. Jin, H. B. Xie, Q. Shi, T. Andreu, M. Lira-Cantú, M. Heggen, R. E. Dunin-Borkowski, N. López, J. R. Galán-Mascarós, J. R. Morante and J. Arbiol, Boosting Photoelectrochemical Water Oxidation of Hematite by Surface States Modification,
DOI:10.2139/ssrn.3327226.
- H. Zhu, M. M. Zhao, J. K. Zhou, W. C. Lia, H. Y. Wang, Z. Xu, L. Lu, L. Pei, Z. Shi, S. C. Yan, Z. S. Li and Z. G. Zou, Surface states as electron transfer pathway enhanced charge separation in TiO2 nanotube water splitting photoanodes, Appl. Catal., B, 2018, 234, 100–108 CrossRef CAS.
- M. Antuch, P. Millet, A. Iwase and A. Kudo, The role of surface states during photocurrent switching: Intensity modulated photocurrent spectroscopy analysis of BiVO4 photoelectrodes, Appl. Catal., B, 2018, 237, 401–408 CrossRef CAS.
- M. R. Nellist, F. A. L. Laskowski, J. J. Qiu, H. Hajibabaei, K. Sivula, T. W. Hamann and S. W. Boettcher, Potential-sensing electrochemical atomic force microscopy for in operando analysis of water-splitting catalysts and interfaces, Nat. Energy, 2018, 3, 46–52 CrossRef CAS.
- N. Zou, X. Zhou, G. Chen, N. M. Andoy, W. Jung, G. Liu and P. Chen, Cooperative communication within and between single nanocatalysts, Nat. Chem., 2018, 10, 607–614 CrossRef CAS PubMed.
- B. H. Han, K. A. Stoerzinger, V. Tileli, A. D. Gamalski, E. A. Stach and Y. Shao-Horn, Nanoscale structural oscillations in perovskite oxides induced by oxygen evolution, Nat. Mater., 2017, 16, 121–126 CrossRef CAS PubMed.
- R. T. Chen, S. Pang, H. Y. An, J. Zhu, S. Ye, Y. Y. Gao, F. T. Fan and C. Li, Charge separation via asymmetric illumination in photocatalytic Cu2O particles, Nat. Energy, 2018, 3, 655–663 CrossRef CAS.
- J. Knöppel, S. Y. Zhang, F. D. Speck, K. J. J. Mayrhofer, C. Scheu and S. Cherevko, Time-resolved analysis of dissolution phenomena in photoelectrochemistry-A case study of WO3 photocorrosion, Electrochem. Commun., 2018, 96, 53–56 CrossRef.
- J. J. Qiu, H. Hajibabaei, M. R. Nellist, F. A. L. Laskowski, T. W. Hamann and S. W. Boettcher, Direct in Situ measurement of charge transfer processes during photoelectrochemical water oxidation on catalyzed hematite, ACS Cent. Sci., 2017, 3, 1015–1025 CrossRef CAS PubMed.
- J. J. Qiu, H. Hajibabaei, M. R. Nellist, F. A. L. Laskowski, S. Z. Oener, T. W. Hamann and S. W. Boettcher, Catalyst deposition on photoanodes: The roles of intrinsic catalytic activity, catalyst electrical conductivity, and semiconductor Morphology, ACS Energy Lett., 2018, 3, 961–969 CrossRef CAS.
- F. A. L. Laskowski, M. R. Nellist, J. J. Qiu and S. W. Boettcher, Metal oxide/(oxy)hydroxide overlayers as hole collectors and oxygen-evolution catalysts on water-splitting photoanodes, J. Am. Chem. Soc., 2019, 141, 1394–1405 CrossRef CAS PubMed.
|
This journal is © The Royal Society of Chemistry 2019 |