DOI:
10.1039/C9NH00344D
(Communication)
Nanoscale Horiz., 2019,
4, 1277-1285
A champagne inspired dual chain-responsive thrombolytic drug release platform based on black phosphorus nanosheets for accelerated thrombolysis†
Received
23rd May 2019
, Accepted 28th June 2019
First published on 4th July 2019
Abstract
To face the clinical challenges related to thrombolytic drugs, such as short blood circulation time, the large dosage required and the side effects of bleeding, the fabrication of a smart controlled thrombolytic drug release nano-system for physical assistant thrombolysis would be very significant. Inspired by champagne, an integrated chain-responsive thrombolytic drug delivery system, uPA@black phosphorus nanosheets-perfluoro-n-pentane@mesoporous silica nanoparticles (uPA@BPNs–PFP@MSNs) was fabricated as a multifunctional platform. The dual chain-responsive thrombolytic drug delivery platform, is first triggered by the photothermic effect of the black phosphorus nanosheets using near-infrared (NIR) laser irradiation (808 nm, 0.2 W cm−2), followed by accelerated thrombolytic drug release and the generation of bubbles from the phase change of the material. This platform was integrated via electrostatic adsorption and the thrombolysis, stability and cytocompatibility in vitro were assessed. Additionally, a murine acute jugular vein thrombosis model was built to evaluate the thrombolysis, biosafety and distribution in vivo. In light of these results, the platform has potential applications for delivering thrombolytic drugs safely and accelerating thrombolysis with a reduced dose of drugs clinically.
New concepts
Thrombolytic drugs are easily neutralized in blood resulting in a less than ideal therapeutic effect. However, excess amounts of thrombolysis drugs can increase the lethal risk of hemorrhage in thrombolysis therapy clinically. Herein, we designed a dual chain-responsive nanoplatform based on black phosphorus (BP) and mesoporous silica nanoparticles (MSNs) for thrombolytic drug delivery. BP can induce hyperthermia under the irradiation of a near-infrared laser, resulting in the accelerated release of the drug (uPA) and the generation of bubbles (the vaporization of perfluoro-n-pentane loaded into the MSNs). Thrombolytic assessment of this platform in vitro and in vivo reveals that the platform presents effective and safe thrombolytic drug delivery with accelerated and enhanced thrombolysis.
|
Introduction
Thrombus diseases such as pulmonary embolism, myocardial infarction and stroke are the leading cause of mortalities and morbidities around the world.1–3 The clinical presentation of these diseases is a thrombosis, namely an occlusive thrombi that hinders blood flow, resulting in ischemic organ necrosis.4,5 Rapid clot lysis is a critical treatment modality for these diseases using intravascular administration of thrombolytic drugs clinically. However, most thrombolytic drugs exhibit a short circulation time, the drug dosage required therefore forces clinicians to administer high doses to compensate for this limitation, which can cause hemorrhagic side effects.6,7 Therefore, achieving effective and accelerated thrombolysis with a reduced dose of thrombolytic drugs is the primary purpose for thrombolysis.
Champagne, a sparkling wine, can be opened by the generation of carbon dioxide through shaking or heating, which can push out the cork immediately. In a blocked vessel, a thrombus is just like a cork. Therefore, we hypothesize that the thrombolytic effect could be accelerated and enhanced if some bubbles, formed by a gas, could be generated around thrombi. Inspired by this wild assumption, we planned to design a novel thrombolytic drug delivery platform combined with physical properties (hyperthermia and bubbles) to enhance thrombolysis.
Hyperthermia, an induced photothermal effect, has been reported in many biomedical applications. Recently, novel nanomaterials with excellent photothermal effects have been introduced for use in bioimaging, phototherapy, delivery of therapeutic agents and combination treatment, such as black phosphorus,8–10 antimonene,11,12 and semiconducting polymer nanoparticles.13–15 However, as far as we know, no research on the use of these nanomaterials for thrombolysis has been reported. Herein, owing to the good biocompatibility and biodegradation of black phosphorus, a near infrared (NIR)-activatable and chain-responsive nano-platform based on black phosphorus nanosheets (BPNs) and mesoporous silica nanoparticles (MSNs) was designed for the first time to deliver uPA and liquid perfluoropentane (PFP) for combined thrombolysis. PFP is a thermally responsive material, which vaporizes at environmental temperatures exceeding 29 °C. However, the boiling point is elevated to 50 °C after encapsulation into nanoparticles and is also affected by blood pressure.16,17 As shown in Fig. 1, uPA and PFP were separately loaded into the BPNs and MSNs, then, the uPA@black phosphorus nanosheets-perfluoro-n-pentane@mesoporous silica nanoparticles (uPA@BPNs–PFP@MSNs) were assembled via electrostatic adsorption owing to the opposite electrical charge of the BPNs and MSNs. Under NIR irradiation (808 nm, 0.2 W cm−2, beam size: 10 cm2), BPNs can induce localized hyperthermia, which can accelerate uPA release and associatively transform PFP to bubbles, resulting in accelerated and combined thrombolysis (uPA, hyperthermia and bubbles). In this work we selected these particular laser parameters owing to the vulnerability of the blood vessels, which would be irradiated directly by the laser. We propose that the present platform could serve as a multifunctional thrombolytic drug delivery system for accelerated thrombolysis.
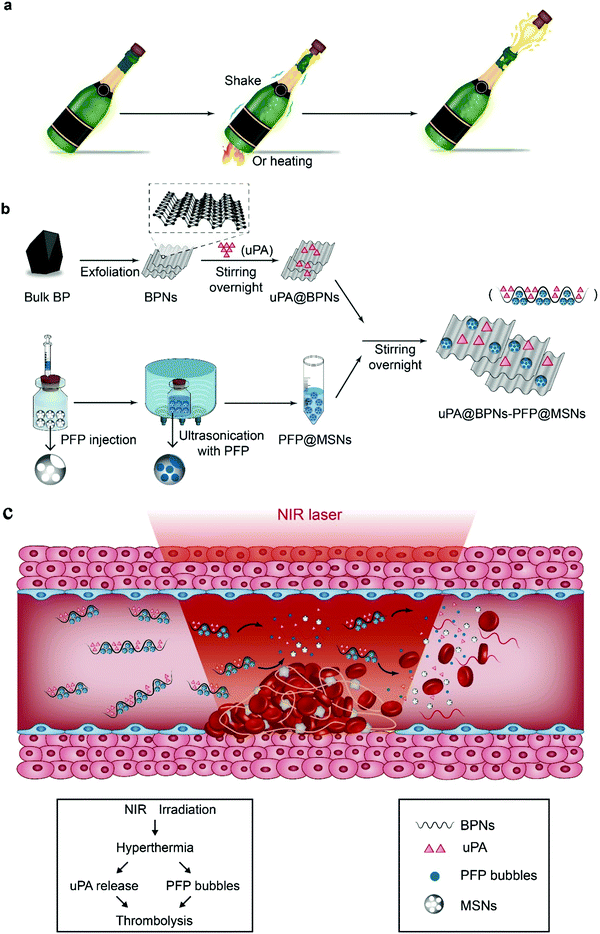 |
| Fig. 1 (a) Champagne can be opened through shaking or heating owing to the generation of bubbles, which inspired us to design the platform. (b) A schematic illustration of the fabrication of the uPA@BPNs–PFP@MSNs platform. (c) A schematic illustration of the thrombolytic mechanism of the uPA@BPNs–PFP@MSNs platform. | |
Results and discussion
Characterization of the designed platform
To fabricate the platform, we first synthesized the BPNs and MSNs respectively according to previously published reports.18,19 The morphology of the BPNs was characterized using scanning electron microscopy (SEM) and transmission electron microscopy (TEM). The SEM image shows the independent sheet structure of the BPNs (Fig. 2a), which was in line with the previously published reports.20,21 The TEM image showed that the lateral size of the BPNs was about 300 nm (Fig. 2b), which was in line with the results of dynamic light scattering (DLS) (Fig. S1, ESI†). BPNs with a smaller lateral size can be obtained by changing the solvents used in the ultrasound sonication.22 Furthermore, the MSNs presented a spherical morphology with a uniform size of about 100 nm (Fig. S2, ESI†). The BPNs and MSNs were then mixed and stirred in water overnight, we observed that the MSNs could attach onto the surface of the BPNs using SEM (Fig. 2c), and the energy dispersive spectrometry (EDS) results also indicated that the platform was indeed composed of BPNs and MSNs (Fig. 2d). In addition, as shown in Fig. 2e, new peaks at 470, 800, 960 and 1100 cm−1 observed in the spectra of the BPNs–MSNs, were ascribed to the absorption peak of the MSNs which appear in the Fourier transform infrared spectroscopy (FTIR) results.23,24 The broad peak at 1100 cm−1 was attributed to the Si–O–Si anti-symmetric stretching mode and the peak at 960 cm−1 was assigned to the Si–OH bending mode. The peak at 800 cm−1 was assigned to the Si–O symmetric stretching mode and the peak at 470 cm−1 was assigned to the Si–O bending mode. Moreover, the BP nanosheets also showed a set of peaks at 3449, 1600 and 1400 cm−1 in the FTIR spectrum. The peak at 3449 cm−1 was attributed to the O−H stretching mode, and the peak at 1600 cm−1 was assigned to the P
O stretching mode. The broad peak centered at 1400 cm−1 was ascribed to the P−O stretching mode. These results suggested that the MSNs were attached onto the surface of the BPNs successfully, which can be ascribed to the opposite charges of the BPNs (negative) and MSNs (positive) (Fig. 2f). The zeta potential of the BPNs was about −24.3 mV, which approached the value reported previously.21,22 Additionally, the platform also presented a robust stability. After placing the BPNs–MSNs in saline for 7 d, the MSNs were still attached onto the surface of the BPNs (Fig. S3, ESI†). Meanwhile, the size distribution of the BPNs–MSNs placed in saline for 7 d changed slightly compared with fresh BPNs–MSNs (at 1 d) (Fig. 2g and h). Furthermore, after placing the BPNs–MSNs in saline for 14 d, no obvious change in the morphology and lateral size of the BPNs–MSNs was observed, indicating their robust stability (Fig. S4, ESI†). In addition, we compared the photothermal effects and the photothermal conversion efficiency of the BPNs and BPNs–MSNs and the results indicated that the attached MSNs would not slow down the rate of temperature elevation of the BPNs–MSNs under NIR irradiation (Fig. 2i and Fig. S5, ESI†). Using eqn (S1) and (S2) (ESI†), the photothermal conversion efficiency of the BPNs was found to be 30.34%, which was in line with the previously reported value.22,25,26 After the MSNs were attached onto the surface of the BPNs, the photothermal conversion efficiency of the BPNs@MSNs was about 28.26%. These results indicated that the platform could be fabricated successfully as a stable and effective drug delivery system.
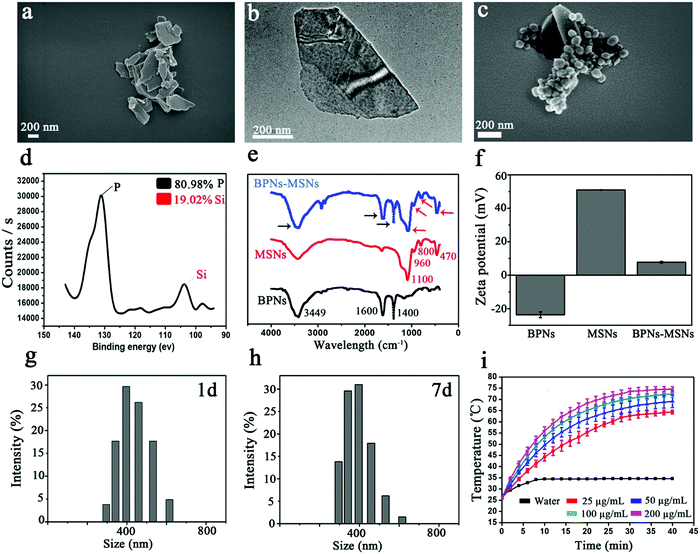 |
| Fig. 2 (a) SEM image of the BPNs; (b) TEM image of the BPNs; (c) SEM image of the BPNs–MSNs; (d) EDS curve for the BPNs–MSNs; (e) FTIR spectra of the BPNs, MSNs and BPNs–MSNs; (f) zeta potentials of the BPNs, MSNs and BPNs–MSNs, (error bars represent the standard deviation of three separate measurements); (g) and (h) size distribution of the BPNs–MSNs placed in saline for 1 d and 7 d; (i) heating curve of the BPNs–MSNs with different concentrations under NIR laser irradiation of 0.2 W cm−2 (error bars represent the standard deviation of three separate measurements). | |
PFP and uPA loading and release
After verifying the feasibility of the BPNs–MSNs, we attempted to load PFP and uPA into the MSNs and BPNs respectively. PFP was encapsulated into the MSNs via a vacuum combined ultrasound perfusion method. As shown in Fig. 3a, the fragment ions of the PFP@MSNs detected using mass spectrometry were matched with the standard substance of PFP (inset in Fig. 3a). Additionally, compared with the MSNs, the absorbed curve and pore volume of the PFP@MSNs also indicated that PFP had been loaded into the MSNs successfully (Fig. 3b and c). Then, after the PFP@MSNs were attached onto the BPNs (BPNs–PFP@MSNs), the vaporization of PFP was observed under different environmental conditions (Fig. S6, ESI†). When the BPNs–PFP@MSNs were placed in saline, abundant bubbles were generated under NIR irradiation for 5 min, while only a few bubbles were observed with treatment at 37 °C for 3 h (Fig. S6a, ESI†). Similar results were observed after the BPNs–PFP@MSNs were cultured with B16 cells (Fig. S6b, ESI†). These results indicated that PFP could be transformed into a gas in this platform under NIR irradiation.
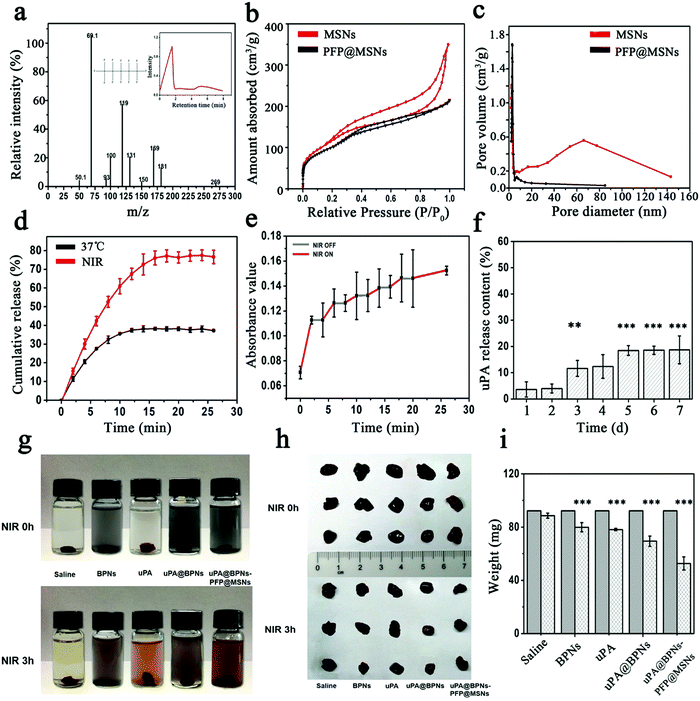 |
| Fig. 3 (a) GC-MS chromatogram of PFP extracted from the prepared PFP@MSNs; (b) BET surface areas of the MSNs and PFP@MSNs; (c) change in pore volume for the MSNs and PFP@MSNs; (d) FITC-uPA release curve for the FITC-uPA@BPNs at 37 °C and under NIR at 0.2 W cm−2 (error bars represent the standard deviation of three separate measurements); (e) pulsed FITC-uPA release from FITC-uPA@BPNs with/without NIR at 0.2 W cm−2 (error bars represent the standard deviation of three separate measurements); (f) uPA release from uPA@MSNs after being placed in saline over 7 d (error bars represent standard deviation of three separate measurements) **P < 0.01, ***P < 0.001; (g) photos of thrombolysis in saline with blood clots treated with BPNs, uPA, uPA@BPNs and uPA@BPNs–PFP@MSNs; (h) photos of thrombi before and after different treatments; (i) change in weight of the thrombi before and after different treatments (error bars represent standard deviation of three separate measurements) ***P < 0.001. | |
To detect the tendency of the thrombolytic drug release, uPA was labelled using fluorescein isothiocyanate (FITC) to give FITC-uPA and monitored using an enzyme-linked immunosorbent assay. After the FITC-uPA@BPNs were formed, the drug loading efficiency of the platform was calculated to be about 92.78% using eqn (3) (ESI†) and the drug loading capacity was calculated to be about 92.78% using eqn (4) (ESI†) (the ratio of W0/WNP was 1), which was similar to that reported previously.21,27 Then, the drug loaded platform was treated at 37 °C or under NIR respectively. As shown in Fig. 3d, the amount of FITC-uPA released approached 80% in 30 min under NIR irradiation, while only 30% FITC-uPA was released in 30 min at 37 °C. The accelerated release was attributed to the hyperthermia triggered by NIR irradiation. Additionally, we performed an additional experiment to evaluate the morphology of the uPA-BPNs@PFP-MSNs before and after irradiation. As shown in Fig. S7 (ESI†), after irradiation for 10 min, partial MSNs were separated from the BPNs, this could explain the accelerated drug release under NIR irradiation. However, the morphology of the BPNs and MSNs did not change. Furthermore, the FITC-uPA@BPNs also presented pulsed FITC-uPA release profiles with/without NIR (Fig. 3e). These results suggested that uPA could be released in a controlled manner from this platform by switching the irradiation of NIR on and off. Additionally, we observed the leakage of FITC-uPA after keeping FITC-uPA@BPNs in saline at 4 °C for 7 d. As shown in Fig. 3f, only about 18% of the FITC-uPA was released over 7 d, indicating that the platform possesses a robust stability in saline.
Thrombolysis evaluation in vitro
Next, the thrombolytic efficacy of the platform was evaluated through the change in weight of blood clots after different treatments in vitro. As shown in Fig. 3g, after being treated with NIR irradiation for 3 h, blood clots lysis could be observed in all groups. However, the images of blood clots indicated that the clot size reduced sharply in the group treated with uPA@BPNs–PFP@MSNs (Fig. 3h). By comparing the weight of the clots before and after the treatment, the clots treated with uPA@BPNs–PFP@MSNs were observed to have the biggest weight loss (Fig. 3i). These results indicated that the uPA@BPNs–PFP@MSNs were endowed with an enhanced thrombolytic efficacy compared with the single components (saline, BPNs, uPA, uPA@BPNs), which was ascribed to the hyperthermia and the induced dual chain reaction (accelerated uPA release and the generation of bubbles).
Biocompatibility of the platform assessment
It is well known that a desirable drug delivery system should possess a good biocompatibility. Therefore, the hemolysis, lactate dehydrogenase (LDH) release and cytotoxicity of the BPNs–MSNs were assessed at different concentrations. As shown in Fig. 4a, no significant hemolysis (<5%) was observed in the red blood cells after treatment with different concentrations of BPNs–MSNs for 6 h at 37 °C. In addition, the BPNs–MSNs presented an excellent cytocompatibility when incubated with human umbilical vein endothelial cells (HUVECs) for 3 d (Fig. 4b). In order to confirm the further synergistic cell viability, HUVECs were co-stained with Calcein-AM and propidium iodide (PI) after the different BPNs–MSNs treatment procedures. As shown in Fig. 4d, the results of the live/dead staining assay also indicated that the BPNs–MSNs presented a limited cytotoxicity to HUVECs. Furthermore, LDH is an injury indicator for the cellular and plasma membrane. To evaluate cellular injury by BPNs–MSNs, we examined the effect of BPNs–MSNs on the endothelial LDH release profile. As shown in Fig. 4c, the release of LDH decreased with the increased concentration of BPNs–MSNs, which is in line with the results found in the cell viability assay. Taken together, these results suggest that BPNs–MSNs possess a favorable cytocompatibility.
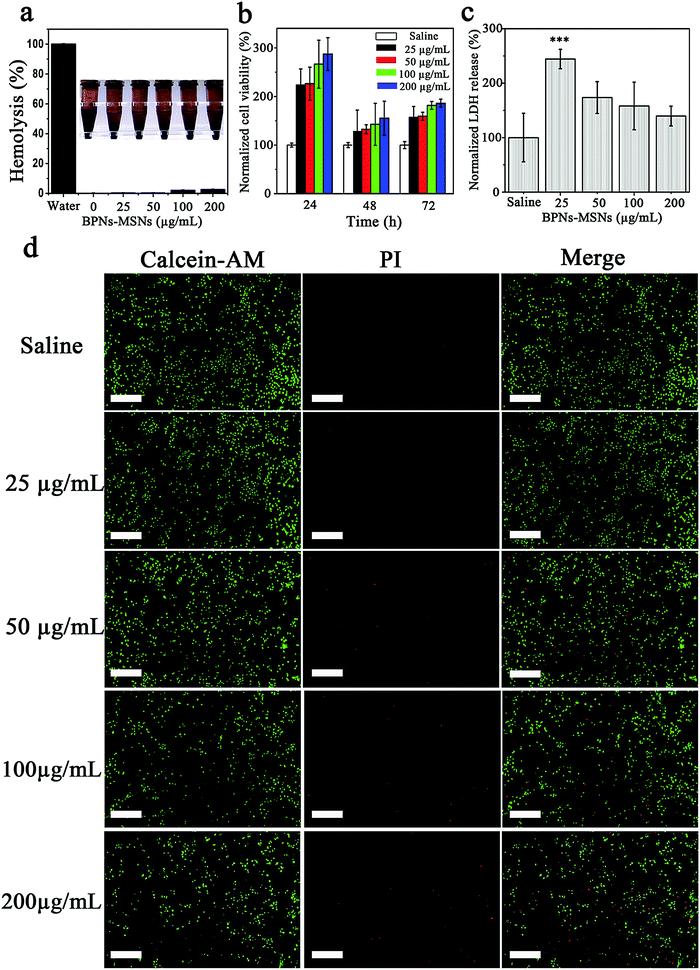 |
| Fig. 4 (a) Hemolysis assays for different concentrations of BPNs–MSNs; (b) cell viability of HUVECs treated with different concentrations of BPNs–MSNs (error bars represent the standard deviation of six separate measurements); (c) LDH release profiles of HUVECs treated with different concentrations of BPNs–MSNs (error bars represent the standard deviation of six separate measurements) ***P < 0.001; (d) live/dead cell assays of HUVECs treated with different concentrations of BPNs–MSNs, the images were photographed using fluorescence microscopy (scale bar: 20 μm). | |
Thrombolysis evaluation in vivo
To evaluate the thrombolytic efficacy of uPA@BPNs–PFP@MSNs in vivo, a murine acute jugular vein thrombosis model was built.28,29 Then, the mice were divided into five groups and were intravenously injected with saline, BPNs, uPA, uPA@BPNs or uPA@BPNs–PFP@MSNs respectively. At 10 min after the injection, the jugular vein was irradiated using NIR for another 10 min. As shown in Fig. 5a, we found that clots still remained in the saline, BPNs, and uPA (white circles) groups, a third of the blood clots had disappeared in the uPA@BPNs (two in six mice) group, and all of the blood clots had disappeared in the uPA@BPNs–PFP@MSNs group after treatment for 24 h. Furthermore, the results of H&E staining also revealed that the clots had been dissolved in the uPA@BPNs–PFP@MSNs group. We speculated that clots could be dissolved in a shorter time after treatment. Therefore, for further comparison of the lysis time, another nine mice exhibiting the acute jugular vein thrombosis model were divided into three groups and treated with uPA, uPA@BPNs or uPA@BPNs–PFP@MSNs separately, each group was then treated with NIR for 10 min. After treatment for 12 h, the images of the jugular veins showed that the clots had been dissolved in the uPA@BPNs–PFP@MSNs group (one in three mice), while the clots could still be observed in the uPA and uPA@BPNs groups (Fig. 5b). In light of these results, we proposed that the uPA@BPNs–PFP@MSNs can enhance clot lysis owing to the combined therapy of hyperthermia, uPA and the generation of bubbles in vivo. Then, we measured the area of the clots in the H&E staining images (blue boundary), these results also revealed that the uPA@BPNs–PFP@MSNs treatment was the best approach for clots lysis in these groups (Fig. S8a and b, ESI†). After treatment for 24 and 12 h respectively, the blood clots were taken out and compared. The results showed that only the clots in the uPA@BPNs–PFP@MSNs group decreased sharply in both the 24 and 12 h treatment, and one of clots was totally dissolved within 24 h (Fig. S8c, ESI†). Furthermore, analysis of the levels of D-dimer and fibrin degradation products (FDP) is an effective method for the noninvasive monitoring of clot dissolution during thrombolytic therapy. Therefore, the D-dimer and FDP level were measured to evaluate the degree of thrombolysis. As shown in Fig. 5c and e, after treatment for 24 h and compared with the other groups, the D-dimer and FDP level presented the highest elevation in the uPA@BPNs–PFP@MSNs group, which indicated that the thrombolytic effect of the uPA@BPNs–PFP@MSNs were the most significant in these treatments. In addition, a similar tendency for the D-dimer and FDP level was also observed after treatment for 12 h (Fig. 5d and f). In light of these results, it can be seen that the uPA@BPNs–PFP@MSNs presented a superior thrombolytic therapy in vivo.
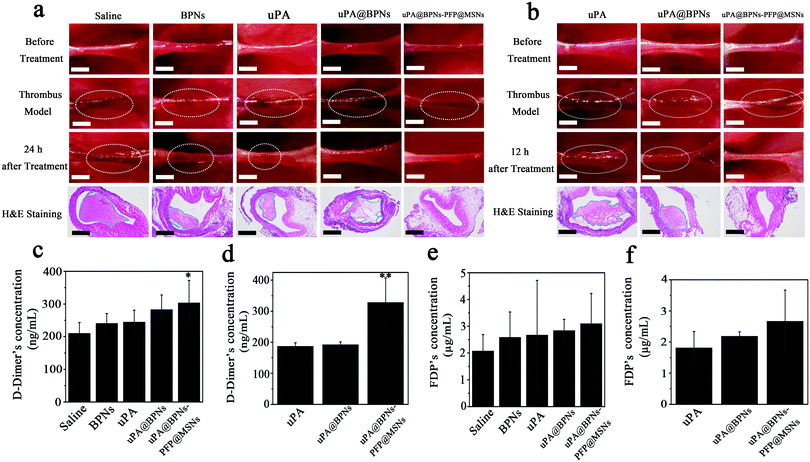 |
| Fig. 5 (a) Photos (clots are marked in white circles, scale bar: 1 cm) and H&E staining (clots are marked with a blue boundary, scale bar: 20 μm) of clots treated with saline, BPNs, uPA, uPA@BPNs and uPA@BPNs–PFP@MSNs before and after NIR irradiation for 24 h (error bars represent the standard deviation of six separate measurements); (b) photos (clots are marked in white circles, scale bar: 1 cm) and H&E staining (clots are marked with a blue boundary, scale bar: 20 μm) of clots treated with uPA, uPA@BPNs and uPA@BPNs–PFP@MSNs before and after NIR irradiation for 12 h (error bars represent the standard deviation of six separate measurements); (c) and (d) concentration of D-dimer in murine blood after treatment for 24 and 12 h (error bars represent the standard deviation of six separate measurements) *P < 0.05, **P < 0.01; (e) and (f) concentration of FDP in murine blood after treatment for 24 and 12 h (error bars represent the standard deviation of six separate measurements). | |
Biodistribution and biosafety assessment in vivo
To evaluate the distribution of the platform in vivo, the organs, blood and urine were collected and the amount of P and Si was determined using an inductively coupled plasma optical emission spectrometer (ICP-MS). As shown in Fig. 6a, P could be detected in all organs after treatment for 24 h, especially in the liver.8 Meanwhile, most of the Si was found in the urine, which indicated that the MSNs could be metabolized through urine (Fig. 6b). As P is an essential element which is beneficial to humans, we suggest that the BPNs could be distributed within the main organs with limited biotoxicity. Furthermore, the biodegradation of this platform was evaluated in vivo by determining the amount of P and Si in the main organs of mice after treatment for 7 d. As shown in Fig. S9 (ESI†), compared with 24 h, the amount of P and Si decreased in the organs of all mice after feeding for 7 d, which suggested that this system (uPA@BPNs–PFP@MSNs) possesses an ideal biodegradation. In addition, the organs of mice treated with saline, BPNs, uPA, uPA@BPNs or uPA@BPNs–PFP@MSNs were separated and treated with H&E staining (Fig. 6c). The results also indicated that the platform could be safely employed for drug delivery in vivo.
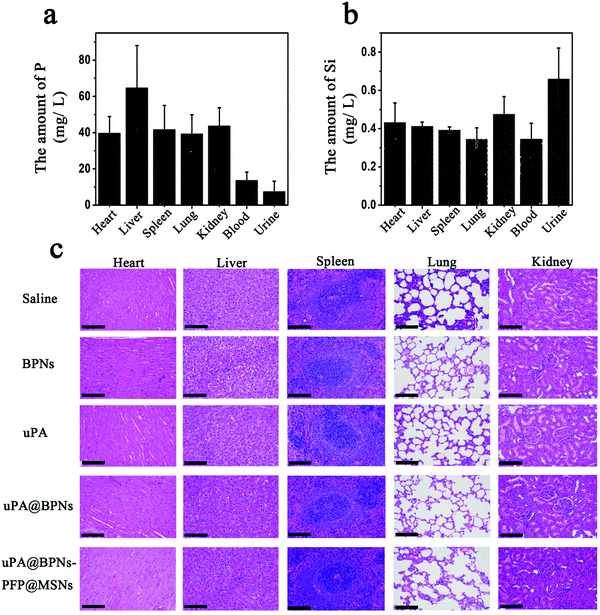 |
| Fig. 6 (a) The amount of P was determined in the major organs, blood and urine after treatment for 24 h (error bars represent the standard deviation of three separate measurements); (b) the amount of Si was determined in the major organs, blood and urine after treatment for 24 h (error bars represent the standard deviation of three separate measurements); (c) H&E staining of major organs after treatment (error bars represent the standard deviation of three separate measurements, scale bar: 20 μm). | |
Conclusions
In summary, for the treatment of many emergency thrombosis diseases, the speed of the drug response time directly determines the quality of the patient's recovery and even the difference between life and death; thus an effective and accelerated thrombolytic drug release nano-system is urgently needed. Inspired by champagne, a biocompatible drug delivery platform based on BPNs and MSNs, encapsulating uPA and PFP, respectively, was prepared as a laser activatable and chain-responsive system for thrombolytic therapy. Owing to the accelerated uPA release and the generation of bubbles, the thrombolytic effect of uPA@BPNs–PFP@MSNs was confirmed to be enhanced and accelerated by hyperthermia in vitro and in vivo. Moreover, future investigations should focus on three subjects: firstly, to optimize the system to achieve more effective thrombolysis with limited thrombolytic drugs; secondly, the targeting performance of this platform in large animal models; and thirdly, applying this platform to other blockage diseases, such as lacrimal duct obstruction. We hope to provide new perspectives for the design of multifunctional nanomedicines and their delivery platforms.
Author contributions
C. H. prepared the experiments and analyzed the data. X. W. D. developed the concept and wrote the manuscript. G. L. Z. and J. Q. L. helped to synthesize the nano-platform. H. OY. and W. Z. helped to build the animal model. M. Y. W. and L. D. helped to accomplish the thrombolytic assessment in vitro. X. W. D., H. B. X. and X. L. W. coordinated the project and supplied the funds.
Conflicts of interest
The authors declare no competing financial interests.
Acknowledgements
This work was supported by the National Natural Science Foundation of China (81760641, 31860263, 21461015, 81270202 and 91339113), the Natural Science Foundation of Jiangxi Province (20161BAB215203 and GJJ150194), the National Key Basic Research Program of China (2013CB531103) and the Nanchang University Seed Grant for Biomedicine.
Notes and references
- S. S. Jolly, J. A. Caims, S. Yusuf, M. J. Rokoss, P. Gao, B. Meeks, S. Kedev, G. Stankovic, R. Moreno, A. Gershlick, S. Chowdhary, S. Lavi, K. Niemela, I. Bernat, W. J. Cantor, A. N. Cheema, P. G. Steg, R. C. Welsh, T. Sheth, O. F. Bertrand, A. Avezum, R. Bhindi, M. K. Natarajan, D. Horak, R. C. M. Leung, S. Kassam, S. V. Rao, M. El-Omar, S. R. Mehta, J. L. Velianou, S. Pancholy, V. Dzavik and T. Investigators, Lancet, 2016, 387, 127–135 CrossRef.
- M. Di Nisio, N. van Es and H. R. Bueller, Lancet, 2016, 388, 3060–3073 CrossRef.
- I. Tabas, Nature, 2016, 536, 33–34 CrossRef.
- H. K. Eltzschig and T. Eckle, Nat. Med., 2011, 17, 1391–1401 CrossRef CAS.
- G. Karthikeyan, Lancet, 2018, 391, 938 CrossRef.
- X.-C. Jiang, J.-J. Xiang, H.-H. Wu, T.-Y. Zhang, D.-P. Zhang, Q.-H. Xu, X.-L. Huang, X.-L. Kong, J.-H. Sun, Y.-L. Hu, K. Li, Y. Tabata, Y.-Q. Shen and J.-Q. Gao, Adv. Mater., 2019, 29, e1807591 CrossRef.
- Y. Zhang, J. Yu, J. Wang, N. J. Hanne, Z. Cui, C. Qian, C. Wang, H. Xin, J. H. Cole, C. M. Gallippi, Y. Zhu and Z. Gu, Adv. Mater., 2017, 29, 1704077 Search PubMed.
- W. Tao, X. Zhu, X. Yu, X. Zeng, Q. Xiao, X. Zhang, X. Ji, X. Wang, J. Shi, H. Zhang and L. Mei, Adv. Mater., 2017, 29, 1603276 CrossRef.
- W. Chen, J. Ouyang, H. Liu, M. Chen, K. Zeng, J. Sheng, Z. Liu, Y. Han, L. Wang, J. Li, L. Deng, Y.-N. Liu and S. Guo, Adv. Mater., 2017, 29, 1603864 CrossRef.
- J. Shao, C. Ruan, H. Xie, Z. Li, H. Wang, P. K. Chu and X.-F. Yu, Adv. Sci., 2018, 5, 1700848 CrossRef.
- V. Lloret, M. A. Rivero-Crespo, J. A. Vidal-Moya, S. Wild, A. Domenech-Carbo, B. S. J. Heller, S. Shin, H. P. Steinruck, F. Maier, F. Hauke, M. Varela, A. Hirsch, A. Leyva-Perez and G. Abellan, Nat. Commun., 2019, 10, 509 CrossRef PubMed.
- W. Tao, X. Ji, X. Zhu, L. Li, J. Wang, Y. Zhang, P. E. Saw, W. Li, N. Kong, M. A. Islam, T. Gan, X. Zeng, H. Zhang, M. Mahmoudi, G. J. Tearney and O. C. Farokhzad, Adv. Mater., 2018, 30, 201806021 Search PubMed.
- Y. Jiang, P. K. Upputuri, C. Xie, Z. Zeng, A. Sharma, X. Zhen, J. Li, J. Huang, M. Pramanik and K. Pu, Adv. Mater., 2019, 31, 1808166 CrossRef PubMed.
- J. Li and K. Pu, Chem. Soc. Rev., 2019, 48, 38–71 RSC.
- Y. Lyu, D. Cui, H. Sun, Y. Miao, H. Duan and K. Pu, Angew. Chem., Int. Ed., 2017, 56, 9155–9159 CrossRef CAS.
- C. Li, Y. Zhang, Z. Li, E. Mei, J. Lin, F. Li, C. Chen, X. Qing, L. Hou, L. Xiong, H. Hao, Y. Yang and P. Huang, Adv. Mater., 2018, 30, 1706150 CrossRef.
- K. Hagisawa, T. Nishioka, R. Suzuki, K. Maruyama, B. Takase, M. Ishihara, A. Kurita, N. Yoshimoto, Y. Nishida, K. Iida, H. Luo and R. J. Siegel, J. Thromb. Haemostasis, 2013, 11, 1565–1573 CrossRef CAS.
- B. Yang, J. Yin, Y. Chen, S. Pan, H. Yao, Y. Gao and J. Shi, Adv. Mater., 2018, 30, 1705611 CrossRef.
- F. Tang, L. Li and D. Chen, Adv. Mater., 2012, 24, 1504–1534 CrossRef CAS.
- H. Wang, X. Yang, W. Shao, S. Chen, J. Xie, X. Zhang, J. Wang and Y. Xie, J. Am. Chem. Soc., 2015, 137, 11376–11382 CrossRef CAS.
- M. Qiu, W. X. Ren, T. Jeong, M. Won, G. Y. Park, D. K. Sang, L.-P. Liu, H. Zhang and J. S. Kim, Chem. Soc. Rev., 2018, 47, 5588–5601 RSC.
- M. Qiu, D. Wang, W. Liang, L. Liu, Y. Zhang, X. Chen, D. K. Sang, C. Xing, Z. Li, B. Dong, F. Xing, D. Fan, S. Bao, H. Zhang and Y. Cao, Proc. Natl. Acad. Sci. U. S. A., 2018, 115, 501–506 CrossRef CAS.
- M. Lee, Y. H. Park, E. B. Kang, A. Chae, Y. Choi, S. Jo, Y. J. Kim, S.-J. Park, B. Min, T. K. An and J. Lee, S.-I. In, S. Y. Kim, S. Y. Park and I. In, ACS Omega, 2017, 2, 7096–7105 CrossRef CAS PubMed.
- F. Chen, H. Hong, Y. Zhang, H. F. Valdovinos, S. Shi, G. S. Kwon, C. P. Theuer, T. E. Barnhart and W. Cai, ACS Nano, 2013, 7, 9027–9039 CrossRef CAS PubMed.
- H. Wang, L. Zhong, Y. Liu, X. Xu, C. Xing, M. Wang, S.-M. Bai, C.-H. Lu and H.-H. Yang, Chem. Commun., 2018, 54, 3142–3145 RSC.
- Y. Lyu, C. Xie, S. A. Chechetka, E. Miyako and K. Pu, J. Am. Chem. Soc., 2016, 138, 9049–9052 CrossRef CAS PubMed.
- J. Shao, H. Xie, H. Huang, Z. Li, Z. Sun, Y. Xu, Q. Xiao, X.-F. Yu, Y. Zhao, H. Zhang, H. Wang and P. K. Chu, Nat. Commun., 2016, 7, 9049–9052 Search PubMed.
- C. Cui, Z. Yang, X. Hu, J. Wu, K. Shou, H. Ma, C. Jian, Y. Zhao, B. Qi, X. Hu, A. Yu and Q. Fan, ACS Nano, 2017, 11, 3298–3310 CrossRef CAS.
- R. Cheng, W. Huang, L. Huang, B. Yang, L. Mao, K. Jin, Q. ZhuGe and Y. Zhao, ACS Nano, 2014, 8, 7746–7754 CrossRef CAS.
Footnotes |
† Electronic supplementary information (ESI) available. See DOI: 10.1039/c9nh00344d |
‡ These authors contributed equally and should be considered co-first authors. |
|
This journal is © The Royal Society of Chemistry 2019 |
Click here to see how this site uses Cookies. View our privacy policy here.