DOI:
10.1039/C9NH00021F
(Communication)
Nanoscale Horiz., 2019,
4, 1185-1193
Porphyrin–palladium hydride MOF nanoparticles for tumor-targeting photoacoustic imaging-guided hydrogenothermal cancer therapy†
Received
13th January 2019
, Accepted 3rd May 2019
First published on 6th May 2019
Abstract
Hydrogen gas, which is an important energy resource, was recently discovered to have high advantage in the treatment of many diseases, but the current constraint is the low efficacy of stable hydrogen storage and delivery. By the virtue of the strong hydrogen bonding and catalytic hydrogenation capacity of Pd, we developed a nanoscale porphyrin–palladium metal–organic framework (Pd-MOF) with highly dispersed Pd atoms as hydrogen carriers to efficiently load highly reductive hydrogen for the tumor-targeted photoacoustic imaging (PAI)-guided hydrogenothermal therapy of cancer. The hydrogenated Pd-MOF (PdH-MOF) exhibited a high hydrogen loading capacity, sustained hydrogen release profile, high tumor targeting ability, high photothermal effect and excellent PAI performance. Utilizing these unique advantages of PdH-MOF nanoparticles, high-efficacy hydrogenothermal therapy was achieved by tumor-targeted delivery, PAI-guided therapy, and the sustained release of high payload of highly reductive hydrogen. This study proposes a new strategy for hydrogen storage, activation, delivery and combined therapy using MOFs as a versatile platform.
New concepts
The targeted delivery and controlled release of hydrogen is urgently needed for cancer hydrogen therapy. Current hydrogen nanomedicine is expected to provide a radical solution. Herein, a nanoscale porphyrin palladium metal–organic framework (Pd-MOF) with highly dispersed Pd atoms was developed. The Pd-MOF nanoparticle exhibited a high hydrogen loading capacity and NIR photothermal conversion efficiency due to the high affinity of hydrogen to palladium and its strong NIR absorbance. A proof-of-concept investigation was performed using this novel hydrogen nanomedicine and was proved to be effective on a mouse breast cancer model. In addition, combined with the NIR-irradiated photothermal effect of the PdH-MOF, a novel hydrogenothermal therapy was proposed. Compared to the existing hydrogen administration methods, PdH-MOF serving as a hydrogen delivery system has the advantages of high efficiency, controlled release, tumor targeted delivery and high biocompatibility. The design and synthesis of PdH-MOF was motivated by the excellent performance of nanomaterials in precision cancer therapy. PdH-MOF is expected to serve as a candidate hydrogen drug of clinical translation potential and to guide the discovery of more hydrogen nanomedicine.
|
Introduction
Hydrogen gas has received much attention as a clean energy resource,1,2 and recently, it has emerged in the forefront of the medical field for its curative effect in several major diseases, such as cardiovascular disease,3–5 neurodegenerative disease6–8 and cancer.9–11 Owing to its high safety to human health, hydrogen has been used as an additive breathing gas for divers for several decades, which was proved to be non-toxic and was once considered to be biologically inert that did not participate in biochemical reactions in vivo. Therefore, it was relatively late when hydrogen was found to possess remarkable potential in medicine. In 2007, Ohsawa et al. reported the cytoprotective function of hydrogen gas against reperfusion oxidative injury and stroke by selectively reducing cytotoxic oxygen radicals.3 Different from the common antioxidants, hydrogen specifically could react with ·OH and ONOO− without any influence on other ROS species that are necessary for maintaining normal cellular physiological activities; therefore, hydrogen is considered to be a green therapeutic gas that does not cause harm to normal tissues and cells.
Importantly, prior to the clinical application of hydrogen, it is necessary to develop a method for the safe and effective hydrogen administration. However, limited by its low solubility in water and strong tissue penetration capability, it is hard to achieve highly efficient and sustainable hydrogen delivery. Current methods of hydrogen administration mainly include the inhalation of hydrogen gas,9,12,13 oral uptake of hydrogen-rich water6 and injection of hydrogen saturated saline,4 but all of these methods have obvious disadvantages such as a low hydrogen utilization efficiency and the inability to realize controlled and targeted delivery. Therefore, new hydrogen delivery methods are urgently desired. Inspired by the remarkable performance of nanomaterials in drug delivery systems, especially for tumor therapy with passive and active targeting, a nanoscale hydrogen gas donor is expected to effectively deliver hydrogen to the tumor sites by using nanomaterials to absorb or produce hydrogen. Palladium nanocrystals, an extraordinary photothermal therapy (PTT) agent with a high photothermal conversion efficiency,14–17 can be a candidate carrier for hydrogen delivery due to their excellent hydrogen adsorption capability.18 From this perspective, herein, we chose single-atom Pd as the metal unit for the construction of porphyrinic metal–organic frameworks (MOFs), given the specific coordination ability of Pd to enhance the loading of amounts of hydrogen,19,20 and designed MOF nanoparticles to achieve targeted hydrogen delivery to cancer cells. In this study, palladium and porphyrin were also chosen as basic building units to prepare hydrogen delivery nanomedicine based on their biocompatibility, as demonstrated by relevant biomedical researches. Recently, Pd-based nanomaterials acting as phothothermal agents have been proved to have excellent biocompatibility in bigger animal models.14–17 Moreover, porphyrin has been demonstrated by the FDA to act as an important photosensitizer for photodynamic therapy (PDT).21–23 Therefore, it was hypothesized that a porphyrin–palladium Pd-MOF could have high biosafety, which has been confirmed in this study.
Photothermal therapy (PTT) is a minimally invasive technique, which uses hyperthermia generated by photothermal agents to kill cancer cells.24 However, accurate control over the PTT temperature remains challenging, and higher temperatures can not only kill cancer cells more easily but can also trigger inflammatory responses, which consequently stimulates tumor regeneration and hinders subsequent therapy.25,26 Therefore, the combined implementation of PTT with anti-inflammatory therapy is a reasonable approach and has been proved to be more efficient.26,27 In view of this, based on the intrinsic anti-tumor properties of hydrogen, the combination of PTT and hydrogen therapy will be of great potential in overcoming cancer.
In this study, taking into consideration this synergistic strategy for cancer treatment, a nanoscale porphyrin–palladium metal–organic framework (Pd-MOF) was rationally designed to realize high efficiency hydrogen delivery and photothermal conversion and finally, hydrogen therapy and thermal therapy were combined (defined as hydrogenothermal therapy) based on the special NIR-absorption and hydrogen affinity to metal palladium (PdH-MOF). The novel Pd hydride-MOF (PdH-MOF) nanomaterials with excellent biocompatibility could achieve a highly effective loading of therapeutic hydrogen using single-atom Pd and served as the redox homeostasis regulator for cancer therapy. In addition, the near-infrared photothermal conversion ability of PdH-MOF enabled PTT and photoacoustic imaging (PAI). Finally, PdH-MOF was designed to realize the photoacoustic imaging-guided hydrogenothermal therapy of cancer.
Results and discussion
Design, synthesis, and characterization of porphyrin–palladium MOF nanoparticles
Pd-MOF nanoparticles were readily prepared by a coordination reaction between sodium tetrachloride palladate (Na2PdCl4) and 5,10,15,20-tetrakis(4-pyridyl)-21H,23H-porphine (TPyP), and then they were hydrogenated into PdH-MOF. First, a flash reaction between Na2PdCl4 and TPyP (a molar ratio of 2
:
1) was completed in the presence of PVP to produce PdCl2-MOF nanoparticles; then, the PdCl2-MOF nanoparticles were reduced by hydrazine to obtain Pd-MOF nanoparticles, which were purged with hydrogen gas in solution to generate PdH-MOF nanoparticles (Fig. 1a). The X-ray diffraction (XRD) spectrum was obtained to analyze the structure of the porphyrin–palladium MOF. The Bragg diffraction peaks of the PdCl2-MOF nanoparticles could be divided into three groups (Fig. S1, ESI†), which corresponded to the three interlayer stacking distances. The porphyrin–palladium MOF in this study exhibited a similar XRD feature as the Cd-TPyP coordination material reported by Qian et al., implying that PdCl2-MOF might also be a multiporphyrin nanocrystal.28,29 The morphology and size of the as-prepared porphyrin–palladium MOF nanoparticles were characterized using transmission electron microscopy (TEM), scanning electron microscopy (SEM) and dynamic light scattering (DLS). The TEM (Fig. 1b) and SEM (Fig. S2, ESI†) images of PdCl2-MOF nanoparticles clearly show uniform spherical morphology. The size that was measured by DLS was found to be 93 nm (DPI = 0.186, Fig. 1c). There was no obvious difference in the morphologies and sizes between PdCl2-MOF, Pd-MOF and PdH-MOF nanoparticles, thus demonstrating that the reduction and hydrogenation processes had almost no influence on the structure and morphology of porphyrin–palladium MOF nanoparticles, indicating their excellent stability. In addition, the PVP surface ligands endowed the nanoparticles with good colloidal dispersion.
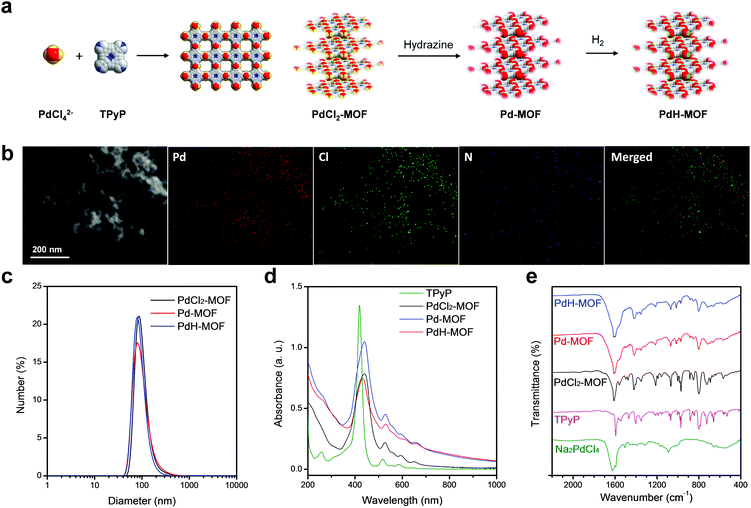 |
| Fig. 1 Synthesis and characterization of PdH-MOF nanoparticles. (a) Schematic of the synthesis process of PdH-MOF nanoparticles. (b) HAADF and elemental mapping images of PdCl2-MOF. (c) DLS size distribution of the aqueous solutions of PdCl2-MOF, Pd-MOF and PdH-MOF nanoparticles. (d) UV-VIS-NIR absorption spectra of free TPyP hydrochloric solution and aqueous solutions of PdCl2-MOF, Pd-MOF and PdH-MOF nanoparticles at the same Pd concentration. (e) FT-IR spectra of TPyP, Na2PdCl4, PdCl2-MOF, Pd-MOF and PdH-MOF. | |
The coordination reaction between Na2PdCl4 and TPyP in a molar ratio of 2
:
1 resulted in porphyrin–palladium MOF nanoparticles, whose structure is shown in Fig. 1a. Specifically, a palladium atom was coordinated with two pyridine groups and two chlorine atoms. At the initial stage of reaction, a planar Pd-TPyP structure was formed and quickly stacked into spherical PdCl2-MOF nanoparticles. After reduction by hydrazine, the chlorine atoms were eliminated, producing homogeneously dispersed zero-valent palladium that could coordinate with hydrogen when hydrogen gas was further introduced. The oxidation state of palladium was characterized using X-ray photoelectron spectroscopy (XPS). The binding energies of the Pd2+ 3d3/2 and 3d5/2 orbitals are 343.0 eV and 337.7 eV for PdCl2-MOF, respectively, and 342.9 eV and 338.0 eV for Pd-MOF, respectively, and for Pd-MOF, the Pd0 3d3/2 and 3d5/2 orbitals are found at 341.1 eV and 335.8 eV, respectively (Fig. S3b, ESI†). When PdCl2-MOF was reduced to Pd-MOF, the Pd/Cl ratio decreased from 0.41 to 0.02 (Fig. S3a, ESI†), meaning that most of the chlorine atoms were eliminated.
The UV-VIS-NIR spectra of porphyrin–palladium MOF nanoparticles and TPyP are shown in Fig. 1d, where the red-shift of the Soret band of porphyrin from 418 nm to 440 nm after coordination into the MOF was ascribed to strong electronic coupling between porphyrin rings. The coordination of Pd to the N atoms of pyridyl groups of TPyP was also supported by FT-IR spectra (Fig. 1e), with the shift in the characteristic peak of pyridine from 1593 cm−1 to 1609 cm−1, while the peak at 970 cm−1 that was attributed to N–H deformation vibrations on pyrrole remained unchanged, indicating that Pd2+ was not coordinated within the porphyrin ring. After reduction by hydrazine, the color of PdCl2-MOF changed from brownish-red to brownish-black. As shown in Fig. 1d, the Pd-MOF nanoparticles exhibited a broad absorption band in the NIR region between 800 nm and 1000 nm, which could be ascribed to the formation of zero-valent palladium. In Fig. S4 (ESI†), the absorbance of Pd-MOF nanoparticles at 808 nm was linearly proportional to the solution concentration within a certain range, and the extinction coefficient (ε) of the Pd-MOF nanoparticles at 808 nm was determined to be 4.7 L g−1 cm−1 according to the absorbance–concentration curve. When the aqueous suspension of the Pd-MOF nanoparticles was bubbled with high purity hydrogen gas, the color of the aqueous suspension turned darker and resulted in PdH-MOF. The absorption intensity of PdH-MOF (Fig. 1d) in the NIR region was a little stronger than that of Pd-MOF, which was consistent in the case of the hydrogenation of palladium nanoparticles.30 It should be noted that the PdCl2-MOF nanoparticles have negligible absorbance at 808 nm, which means that the photothermal effect of Pd-MOF was contributed to by the zero-valent palladium alone instead of porphyrin or PdCl2. The main function of porphyrin is to serve as a framework unit of the MOF materials to coordinate with single-atom Pd. Therefore, PdH-MOF has a higher hydrogen loading capacity (Pd:H = 1), which is much higher than that of the PdH0.2 nanocrystals reported previously by us,30 thus making a stronger contribution to hydrogen therapy. Furthermore, compared with other MOFs based on Pd, PdCl2-MOF nanoparticles have excellent fluorescence (Fig. S5, ESI†) owing to the incorporation of porphyrin, which could serve as a fluorescence probe for the in vivo tracing of nanoparticles. PdCl2-MOF nanoparticles exhibited a similar fluorescence feature to free TPyP (Fig. S5, ESI†), while after reduction, the fluorescence of Pd-MOF and PdH-MOF nanoparticles completely vanished. Since PdH-MOF nanoparticles have the same morphology and size as PdCl2-MOF nanoparticles, PdCl2-MOF nanoparticles were used for in vitro and in vivo tracing by fluorescence imaging.
Reductive hydrogen release
Hydrogen can reduce methylene blue to colorless leucomethylene blue (leucoMB) through the catalysis of colloidal platinum.31 In this study, the reaction was employed for the determination of the hydrogen content in PdH-MOF that was catalyzed by PdH-MOF nanoparticles without colloidal platinum. The reaction was conducted in a sealed cuvette to maintain air-free conditions, and the decreasing intensity of the MB absorbance at 664 nm was used to calculate the amount of released hydrogen from the PdH-MOF nanoparticles. As shown in Fig. 2a and Fig. S6 (ESI†), at the initial stage, MB molecules reacted quickly with reductive hydrogen on the surface of the PdH-MOF nanoparticles, and the reaction rate was slowed down gradually over time. It was worth noting that the complete release of hydrogen from PdH-MOF took about 5 days, exhibiting a sustained-release behavior. This meant that PdH-MOF had a high reductive and self-catalytic capability, greatly favoring the quick and long-acting scavenging of intracellular overexpressed ˙OH. According to the equilibrium concentration of MB, the yield of reductive hydrogen production was obtained, and the ratio of H to Pd in PdH-MOF was consequently calculated to be about 1, which is 5 times higher compared with that of the PdH0.2 (H
:
Pd = 0.2) nanoparticles reported in our previous study. Compared with hydrogen ultrasonic microbubbles,32 the PdH-MOF possesses a higher hydrogen loading capacity and sustained hydrogen release profile, which will greatly contribute to its therapeutic effect. In comparison, the reaction between MB and hydrogen gas saturated water quickly reached equilibrium at 5 h, and only a tiny fraction of hydrogen (0.08%) was consumed by MB in the absence of a catalyst, such as Pt, which suggested the stronger reductive property of hydrogen in PdH-MOF nanoparticles as compared to that of the molecular hydrogen in water. The release process proved that the hydrogen in the PdH-MOF nanoparticles was reductive and showed a sustained-release behavior. In addition, the thermal gravity analyses (TGA) of Pd-MOF and PdH-MOF without PVP were carried out. It was found that the TGA curves of Pd-MOF and PdH-MOF had quick weight losses of 63.4% and 68.6% over 120–320 °C, respectively (Fig. S7, ESI†). The higher weight loss of PdH-MOF was an unambiguous evidence for the efficient hydrogen loading of the particles.
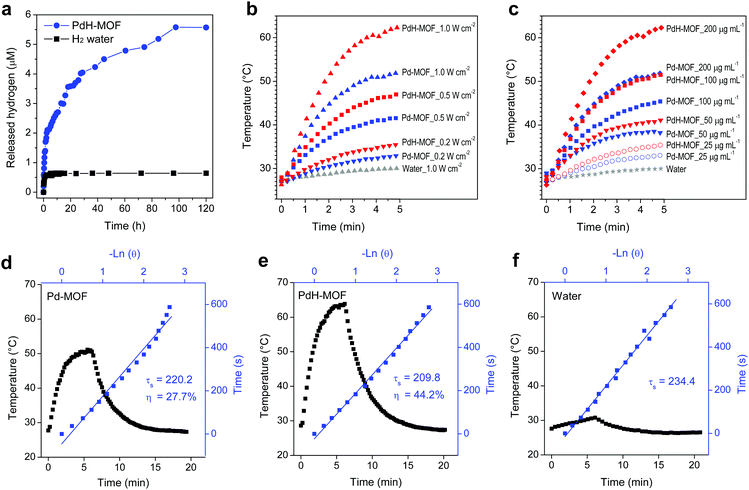 |
| Fig. 2 Hydrogen release behavior and photothermal conversion effect of PdH-MOF. (a) Reactive hydrogen release behavior of aqueous suspensions of PdH-MOF and hydrogen gas saturated water measured using MB probe. (b and c) Photothermal curves of aqueous suspensions of Pd-MOF and PdH-MOF after 808 nm laser irradiation at different power densities (0.2, 0.5 and 1 W cm−2, at the same concentration of 200 μg mL−1) or different nanoparticle concentrations (25, 50, 100, 200 μg mL−1, at the same power density of 1 W cm−2), pure water irradiated at 1 W cm−2 was taken as the control. (d–f) Calculation of the photothermal-conversion efficiency (η) of Pd-MOF and PdH-MOF at 808 nm. The photothermal effect of an aqueous dispersion of Pd-MOF and PdH-MOF under irradiation with 808 nm laser for 6 min, and then the laser was switched off. | |
Photothermal effect
The photothermal conversion effect of Pd-MOF and PdH-MOF nanoparticles was measured under irradiation with an 808 nm laser using a thermal imaging camera. First, three power densities of 0.2, 0.5 and 1.0 W cm−2 were used at the same particle concentration of 200 μg mL−1 and pure water was used as the control. The solution temperature increased with the increase in the power density, with the maximum temperature elevation of 24.9 °C and 36.3 °C for Pd-MOF and PdH-MOF at 1 W cm−2, respectively (Fig. 2b). When the power density was fixed at 1 W cm−2, the photothermal effect was enhanced by the increase in nanoparticle concentration. When the concentration increased from 25 to 200 μg mL−1, the solution temperature rapidly increased by 6.4–24.9 °C for Pd-MOF and by 8.3–36.3 °C for PdH-MOF after irradiation for 5 min (Fig. 2c). In contrast, pure water had a weak temperature elevation (3 °C) at the highest power density (1.0 W cm−2). In Fig. 2d–f, the photothermal conversion efficiencies (η) at 808 nm were calculated to be 27.7% for Pd-MOF and 44.2% for PdH-MOF. We concluded that PdH-MOF exhibited better photothermal performance than Pd-MOF, suggesting it could serve as an ideal photothermal therapy agent.
In vitro hydrogenothermal therapy
At first, the uptake of nanoparticles was investigated using fluorescent PdCl2-MOF nanoparticles. As shown in Fig. 3a, the HeLa cells were stained by DAPI after incubation with PdCl2-MOF nanoparticles for 1 h, and the red fluorescence around the blue DAPI-stained nuclear region clearly showed the intracellular uptake of nanoparticles by the HeLa cells. On the basis of the effective uptake of nanoparticles, we evaluated the therapeutic effect of the hydrogen therapy and hydrogenothermal combination of PdH-MOF nanoparticles using the CCK-8 assay. Pd-MOF nanoparticles as a non-hydrogen control almost had no influence on the viability of HeLa cells at the nanoparticle concentration of 0–200 μg mL−1 (Fig. 3b), while PdH-MOF nanoparticles showed significant suppression on the growth of cancer cells, indicating the effect of hydrogen therapy. By contrast, MCF-10A cells (Fig. S8, ESI†) were chosen as a normal cell model to assess the biocompatibility of nanoparticles. After incubation with Pd-MOF or PdH-MOF nanoparticles, MCF-10A cell viabilities were not affected irrespective of the nanoparticle concentration (12.5–200 μg mL−1), indicating that the high cancer selectivity of PdH-MOF is similar to that of the PdH0.2 nanoparticles developed previously. For combined hydrogenothermal therapy investigations, HeLa cells were respectively incubated with Pd-MOF and PdH-MOF nanoparticles, and 3 hours later were irradiated by an 808 nm laser at power intensities of 0.5 and 1.0 W cm−2, respectively. As shown in Fig. 3b, the best therapeutic efficacy was acquired in the hydrogenothermal therapy group (PdH-MOF + laser), while the suppression on the cell growth in the individual PTT (Pd-MOF + laser) and hydrogen therapy (PdH-MOF, without laser) groups was obviously lower than in the combined hydrogenothermal therapy group, despite the cancer cells being irradiated at 0.5 W cm−2 or 1.0 W cm−2. This suggested that the hydrogenothermal combination had a greater suppression effect on the HeLa cells. A similar combination therapeutic effect was also obtained on 4T1 cells, as shown in Fig. S9 (ESI†). The synergistic treatment of cancer cells by PdH-MOF nanoparticles was ascribed to the elevated temperatures induced by the highly efficient photothermal conversion of the nanoparticles and the sustained hydrogen release in the cancer cells. For more direct evidence of cancer cell ablation, the CLSM images of the HeLa cells were acquired after treatment with the laser, Pd-MOF, Pd-MOF + laser, and PdH-MOF + laser, respectively, followed by the living and dead cell staining with calcein-AM (green) and PI (red). The partially red fluorescence of cells in the hydrogen therapy group and PTT group clearly proved their efficacies compared with the totally green fluorescence in the PBS, PBS + laser and Pd-MOF groups (Fig. 3c). The strong red fluorescence that represents dead cells in the PdH-MOF + laser treated group indicated the high efficiency of the combined hydrogenothermal therapy using PdH-MOF nanoparticles.
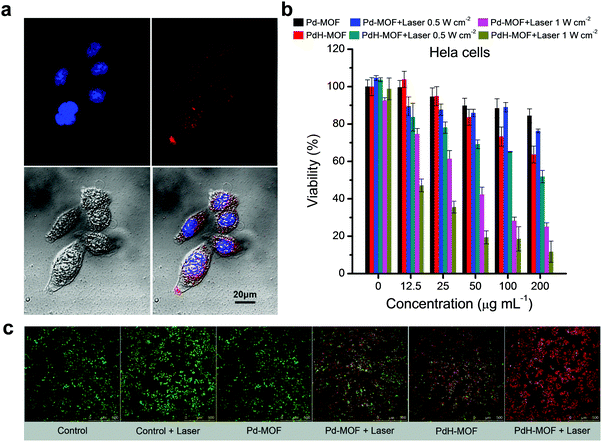 |
| Fig. 3
In vitro therapy efficacies and endocytosis of cancer cells. (a) CLSM images of HeLa cells incubated with PdCl2-MOF nanoparticles for 1 h. HeLa cell nuclei stained by 4′,6-diamidino-2-phenylindole (DAPI) (blue) and porphyrin fluorescence from PdCl2-MOF in cells (red). (b) Cell viability of HeLa cells treated with different concentrations of nanoparticles for 24 h with or without 808 nm laser irradiation (5 min, 0.5 and 1 W cm−2). (c) Confocal fluorescence imaging of combined hydrogenothermal therapy. HeLa cells were treated with Pd-MOF or PdH-MOF nanoparticles at the same concentration of 200 μg mL−1 with or without 1 W cm−2 of 808 nm laser irradiation (10 min). | |
Photoacoustic imaging (PAI) and fluorescence imaging of PdH-MOF
In order to implement PAI, the imageable performance of PdH-MOF nanoparticles was investigated using the Vevo 2100 LAZR system in vitro and in vivo. As expected, PdH-MOF nanoparticles with excellent photothermal conversion capabilities also showed significant PA signal in solution. The PA signal produced by the PdH-MOF nanoparticles were in linear relation with the nanoparticle concentration (Fig. S10, ESI,†y = 0.026x + 0.382, R2 = 0.99). The strong PA signal observed in the solution containing PdH-MOF nanoparticles suggested that PdH-MOF could be a desirable PAI probe in vivo. The in vivo PAI test was performed on 4T1 tumor-bearing mice. After the intravenous injection of PdH-MOF nanoparticles for 1 h, an apparent PA signal emerged within the region of the tumor, and the signal intensity (PA Avr) was maintained at a relatively high and stable level during 24 h without significant decline (Fig. 4b and c). The rapid increase in the PA signal intensity in tumors indicated the passive targeting performance of PdH-MOF nanoparticles. In brief, the in vitro and in vivo imaging results suggested that PdH-MOF nanoparticles showed great potential for the following PAI guidance of hydrogenothermal therapy.
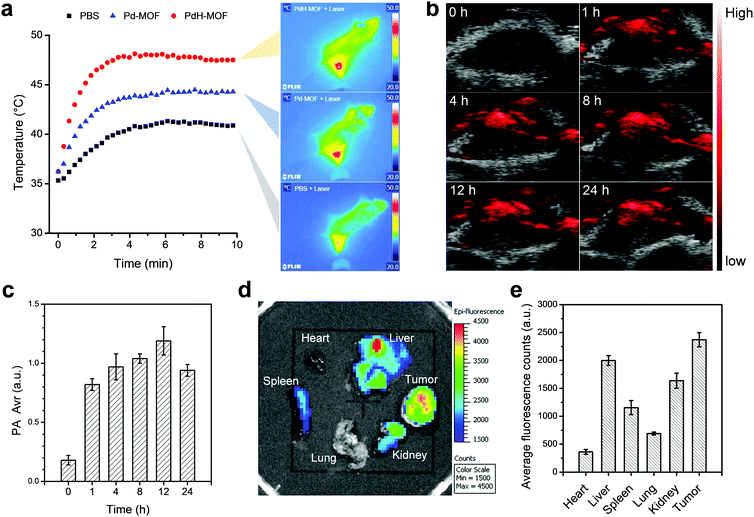 |
| Fig. 4
In vivo photothermal imaging, photoacoustic imaging and fluorescence imaging. (a) Photothermal imaging of tumors on 4T1 tumor-bearing mice. Mice were treated with PBS, aqueous suspensions of Pd-MOF and PdH-MOF nanoparticles prior to the 808 nm laser irradiation. (b) PAI images of the 4T1 tumor at different time points (0, 1, 4, 8, 12, 24 h) after the intravenous injection of an aqueous dispersion of PdH-MOF. (c) Quantitative analysis of intratumoral accumulation and retention of PdH-MOF nanoparticles from PAI data. (d) Ex vivo fluorescence imaging of main organs of 4T1 tumor-bearing mouse at 8 h after being treated with PdCl2-MOF. (e) Biodistribution of PdCl2-MOF nanoparticles represented by average fluorescence intensity in main organs. | |
PdCl2-MOF nanoparticles were intravenously injected into 4T1 tumor-bearing mice via the tail vein to evaluate the biodistribution of therapeutic agents using ex vivo fluorescence imaging. It should be noted that the absorbance of PdCl2-MOF at 605 nm is stronger than that of free porphyrin, and the fluorescence intensity excitated at 605 nm is still high enough for the ex vivo fluorescence imaging of mice. Therefore, 605 nm was chosen as the excitation wavelength rather than 430 nm for mice imaging. Major organs including the heart, liver, spleen, lung, kidney and tumor were collected from mice for fluorescence imaging 8 h after the intravenous injection of the PdCl2-MOF nanoparticles. As shown in Fig. 4d and e, the average fluorescence intensity of major organs was in the following order: tumor > liver > kidney > spleen > heart and lung. The strongest fluorescence signal in the tumor site indicated that the effective tumor targeting of the nanoparticles was aided by the enhanced permeability and retention (EPR) effect. Particle size is an important factor for passive tumor targeting; sub-100 nm particles with excellent performance in tumor accumulation appear to be ideally suited for tumor-targeted drug delivery. The high tumor targeting capacity of PdCl2-MOF should be attributed to its uniform size distribution in the nanoscale (93 nm, DPI = 0.186) and good dispersivity. In consideration of the potential of in vivo applications by intravenous injection, a safety assessment of PdH-MOF nanoparticles was conducted, and the haematology and blood biochemical analysis results (Fig. S11 and S12, ESI†) demonstrated that PdH-MOF possessed good biocompatibility at a high injection dosage of 100 mg kg−1 and was therefore appropriate for cancer theranostics.
In vivo hydrogenothermal therapy
Encouraged by the high photothermal conversion efficiency and sustained reductive hydrogen release as well as efficient in vitro combined hydrogenothermal therapy of cancer cells, the in vivo combined hydrogenothermal therapy performance of PdH-MOF nanoparticles was systematically evaluated by the intravenous administration of nanoparticles into 4T1 tumor-bearing mice, followed by 808 nm laser irradiation. The 4T1 tumor model was established by the subcutaneous injection of murine 4T1 breast cancer cells into the hind limb of female BALB/c (four weeks old) mice. When tumor volumes reached about 200 mm3, mice were randomly divided into five groups (n = 5), (a) blank control group (PBS), (b) NIR control group (intravenously injected with PBS followed by exposure to NIR irradiation), (c) Pd-MOF control group (only intravenously injected with Pd-MOF nanoparticles), (d) thermal therapy group (intravenously injected with Pd-MOF nanoparticles, followed by exposure to NIR irradiation), (e) hydrogen therapy group (only intravenously injected with PdH-MOF nanoparticles), and (f) hydrogenothermal therapy group (intravenously injected with PdH-MOF nanoparticles followed by exposure to NIR irradiation). For NIR irradiation-involved groups, the tumor sites of mice were exposed to the NIR laser at the same power density of 0.5 W cm−2 for 10 min each time at fixed time points (day 1, 2, and 4) after an 1 h post-injection of PBS, Pd-MOF, or PdH-MOF nanoparticles (100 μL, 10 mg kg−1). During the process of PTT, temperature variations of the tumors were monitored by a near infrared imaging camera. As shown in Fig. 4a and b, after four minutes of NIR irradiation, the temperature in the thermal therapy group and hydrogenothermal therapy group increased quickly up to 44.3 °C and 48.1 °C, respectively. By contrast, the temperature in the NIR control group only reached 40.8 °C. The distinctly different temperature rise confirmed the potential of the in vivo photothermal therapeutic applications of Pd-MOF and PdH-MOF. After a 17 day observation, the tumor growth curves are shown in Fig. 5b. Compared with the PBS group, both the NIR control group and the Pd-MOF control group had no effect on the tumor growth, while the hydrogen therapy group and the thermal therapy group caused a slight suppression on the tumor growth. Importantly, in the hydrogenothermal therapy group, the growth of tumors was totally suppressed during the whole process of treatment in 17 days, indicating a remarkable therapeutic efficacy by combination of the PTT and hydrogen therapy. In addition, the tumor growth curve of the hydrogenothermal therapy group in Fig. 5b showed a remarkable decrease in the tumor volume, even though no more NIR-irradiation was provided after 4 days post-injection, which meant that the tumors were still suppressed in the later period (day 5–day 17), possibly owing to the sustained hydrogen release profile of PdH-MOF nanoparticles. The combined hydrogenothermal therapy exhibited a synergetic anti-cancer effect in accordance with the in vitro cytotoxicity results. The enhanced therapeutic effect of the combined therapy was attributed to the enhanced photothermal effect of PdH-MOF nanoparticles, as compared to Pd-MOF nanoparticles, as well as the inherent anti-tumor effect of hydrogen. As we previously proved with PdH0.2 nanocrystals, the mechanism of hydrogen cancer therapy derives from the effect of hydrogen on the intratumoral ROS level and tumor energy metabolism.30 Photothermal therapy (PTT) using gold nanoshells or other photothermal agents can also yield high efficacy cancer therapy owing to the high photothermal conversion efficiency.33–39 Different from PTT, the hydrogenothermal therapy has a low heating requirement owing to the synergetic effect of hydrogen and heating. At a low laser power density of 0.5 W cm−1, Pd-MOF nanoparticles exhibited a poor PTT efficacy, but Pd-MOF nanoparticles displayed remarkably higher efficacy of tumor inhibition under the same conditions. Lastly, the weights of mice in each treatment group had no obvious change during the whole treatment process (Fig. 5a). The main organs of mice (heart, liver, spleen, lung, and kidney) were harvested after a 17 day treatment for histopathological evaluation by hematoxylin and eosin (H&E) staining. The results (Fig. S13, ESI†) showed that all the treatment groups did not cause obvious damage to the main organs, reflecting the high tissue compatibility of the PdH-MOF nanoparticles.
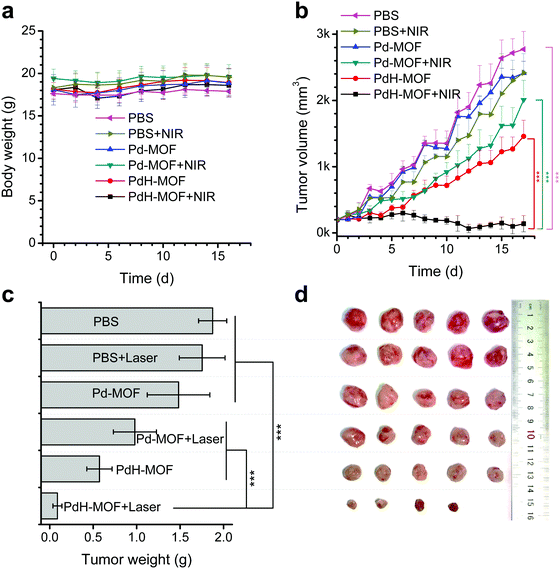 |
| Fig. 5
In vivo combined hydrogenothermal therapy efficacies of PdH-MOF. (a) Time-dependent body-weight curves of 4T1 tumor-bearing mice during treatment. (b) Time-dependent tumor growth curves (n = 5, mean ± SD) during different treatments (PBS, PBS + laser, Pd-MOF, Pd-MOF + laser, PdH-MOF, and PdH-MOF + laser). (c) Tumor weight comparison after 17 day treatment. (d) Photographs of extracted 4T1 tumors after 17 day treatment (n = 5). P values were calculated by the two-tailed Student's t-test (***P < 0.005, **P < 0.01, *P < 0.05). | |
Conclusion
A porphyrin–palladium MOF, based on the coordination between palladium and pyridine groups on the periphery of TPyP, was synthesized. After the reduction and hydrogenation of single-atom Pd in the Pd-MOF, the obtained PdH-MOF exhibited a uniform size distribution on the nanoscale, good aqueous dispersibility, excellent photothermal efficacy (photothermal conversion efficiency: 44.2%) and photoacoustic imaging properties, sustained reductive hydrogen release behavior, and high tumor targeting. Collectively, we showed a proof-of-concept study that PdH-MOF nanoparticles can be used for hydrogenothermal cancer therapy. PdH-MOF nanoparticles exhibited synergetic anti-cancer effects in vitro and in vivo.
Conflicts of interest
There are no conflicts to declare.
Acknowledgements
We greatly appreciate the help of Dr Heliang Yao (SIC, CAS) with TEM measurements and Feng Zhou (KeLiEn Hydrogen Molecular Biomedicine Institute) in support of the hydrogenation equipment (hydrogen gas generator). We thank the financial support from the National Natural Science Foundation of China (51872188, 81701827), Shenzhen Basic Research Program (JCYJ20170302151858466, JCYJ20170818093808351, GJHZ20180418190532315), Shenzhen Peacock Plan (KQTD2016053112051497), and Natural Science Foundation of SZU (827-000143).
References
- L. Peraldo Bicelli, Int. J. Hydrogen Energy, 1986, 11, 555–562 CrossRef CAS.
- I. P. Jain, Int. J. Hydrogen Energy, 2009, 34, 7368–7378 CrossRef CAS.
- K. C. Wood and M. T. Gladwin, Nat. Med., 2007, 13, 673–674 CrossRef CAS PubMed.
- X. Zheng, Y. Mao, J. Cai, Y. Li, W. Liu, P. Sun, J. H. Zhang, X. Sun and H. Yuan, Free Radical Res., 2009, 43, 478–484 CrossRef CAS PubMed.
- M. Tanaka, G. K. Mokhtari, R. D. Terry, F. Gunawan, L. B. Balsam, G. Hoyt, K. H. Lee, P. S. Tsao and R. C. Robbins, Neuropsychopharmacology, 2005, 24, 1906–1914 Search PubMed.
- K. Nagata, N. Nakashima-Kamimura, T. Mikami, I. Ohsawa and S. Ohta, Neuropsychopharmacology, 2008, 34, 501–508 CrossRef PubMed.
- K. Fujita, T. Seike, N. Yutsudo, M. Ohno, H. Yamada, H. Yamaguchi, K. Sakumi, Y. Yamakawa, M. A. Kido, A. Takaki, T. Katafuchi, Y. Tanaka, Y. Nakabeppu and M. Noda, PLoS One, 2009, 4, e7247 CrossRef PubMed.
- J. Li, C. Wang, J. Zhang, J. Cai, Y. Cao and X. Sun, Brain Res., 2010, 1328, 152–161 CrossRef CAS PubMed.
- M. Dole, F. R. Wilson and W. P. Fife, Science, 1975, 190, 152–154 CrossRef CAS PubMed.
- Y. Saitoh, Y. Yoshimura, K. Nakano and N. Miwa, Exp. Oncol., 2009, 31, 156–162 CAS.
- L. Zhao, C. Zhou, J. Zhang, F. Gao, B. Li, Y. Chuai, C. Liu and J. Cai, Int. J. Biol. Sci., 2011, 7, 297–300 CrossRef CAS.
- J. Qu, Y. Gan, K. Xie, W. Liu, Y. Wang, R. Hei, W. Mi and J. Qiu, Acta Pharmacol. Sin., 2012, 33, 445–451 CrossRef CAS PubMed.
- K. Xie, Y. Yu, Y. Pei, L. Hou, S. Chen, L. Xiong and G. Wang, Shock, 2010, 34, 90–97 CrossRef CAS PubMed.
- S. Tang, M. Chen and N. Zheng, Small, 2014, 10, 3139–3144 CrossRef CAS PubMed.
- S. Shi, Y. Huang, X. Chen, J. Weng and N. Zheng, ACS Appl. Mater. Interfaces, 2015, 7, 14369–14375 CrossRef CAS PubMed.
- S. Tang, M. Chen and N. Zheng, Nano Res., 2015, 8, 165–174 CrossRef CAS.
- J. Li, H. Liu, J. Ming, D. Sun, X. Chen, X. Liu and N. Zheng, Chem. Sci., 2019, 10, 1677–1686 RSC.
- R. Griessen, N. Strohfeldt and H. Giessen, Nat. Mater., 2016, 15, 311–317 CrossRef CAS PubMed.
- J. A. Navarro, E. Barea, J. M. Salas, N. Masciocchi, S. Galli, A. Sironi, C. O. Ania and J. B. Parra, Inorg. Chem., 2006, 45, 2397–2399 CrossRef CAS PubMed.
- J. A. R. Navarro, E. Barea, J. M. Salas, N. Masciocchi, S. Galli, A. Sironi, C. O. Ania and J. B. Parra, J. Mater. Chem., 2007, 17, 1939–1946 RSC.
- J. Zhang, Y. Liang, X. Lin, X. Zhu, L. Yan, S. Li, X. Yang, G. Zhu, A. L. Rogach, P. K. N. Yu, P. Shi, L. Tu, C. Chang, X. Zhang, X. Chen, W. Zhang and C. Lee, ACS Nano, 2015, 9, 9741–9756 CrossRef CAS PubMed.
- J. Wang, Y. Zhong, X. Wang, W. Yang, F. Bai, B. Zhang, L. Alarid, K. Bian and H. Fan, Nano Lett., 2017, 17, 6916–6921 CrossRef CAS PubMed.
- D. Wang, L. Niu, Z. Qiao, D. Cheng, J. Wang, Y. Zhong, F. Bai, H. Wang and H. Fan, ACS Nano, 2018, 12, 3796–3803 CrossRef CAS PubMed.
-
M. Norouzi, B. Nazari and D. W. Miller, in Electrospun Materials for Tissue Engineering and Biomedical Applications, ed. T. Uyar and E. Kny, Woodhead Publishing, 2017, 15, 337–356 Search PubMed.
- J. R. Melamed, R. S. Edelstein and E. S. Day, ACS Nano, 2015, 9, 6–11 CrossRef CAS PubMed.
- Q. Dong, X. Wang, X. Hu, L. Xiao, L. Zhang, L. Song, M. Xu, Y. Zou, L. Chen, Z. Chen and W. Tan, Angew. Chem., Int. Ed., 2018, 57, 177–181 CrossRef CAS PubMed.
- R. K. Pathak, S. Marrache, J. H. Choi, T. B. Berding and S. Dhar, Angew. Chem., Int. Ed., 2014, 53, 1963–1967 CrossRef CAS PubMed.
- B. Liu, D. Qian, H. Huang, T. Wakayama, S. Hara, W. Huang, C. Nakamura and J. Miyake, Langmuir, 2005, 21, 5079–5084 CrossRef CAS PubMed.
- D. Qian, T. Wakayama, C. Nakamura and J. Miyake, J. Phys. Chem. B, 2003, 107, 3333–3335 CrossRef CAS.
- P. Zhao, Z. Jin, Q. Chen, T. Yang, D. Chen, J. Meng, X. Lu, Z. Gu and Q. He, Nat. Commun., 2018, 9, 4241 CrossRef PubMed.
- T. Seo, R. Kurokawa and B. Sato, Med. Gas. Res., 2012, 2, 1–6 CrossRef CAS PubMed.
- Y. He, B. Zhang, Y. Chen, Q. Jin, J. Wu, F. Yan and H. Zheng, ACS Appl. Mater. Interfaces, 2017, 9, 21190–21199 CrossRef CAS PubMed.
- R. Bardhan, W. Chen, C. Perez-Torres, M. Bartels, R. M. Huschka, L. Zhao, E. Morosan, R. G. Pautler, A. Joshi and N. J. Halas, Adv. Funct. Mater., 2009, 19, 3901–3909 CrossRef CAS.
- C. Ayala-Orozco, C. Urban, M. W. Knight, A. S. Urban, O. Neumann, S. W. Bishnoi, S. Mukherjee, A. M. Goodman, H. Charron, T. Mitchell, M. Shea, R. Roy, S. Nanda, R. Schiff, N. J. Halas and A. Joshi, ACS Nano, 2014, 8, 6372–6381 CrossRef CAS PubMed.
- L. R. Hirsch, R. J. Stafford, J. A. Bankson, S. R. Sershen, B. Rivera, R. E. Price, J. D. Hazle, N. J. Halas and J. L. West, Proc. Natl. Acad. Sci. U. S. A., 2003, 100, 13549–13554 CrossRef CAS PubMed.
- C. Ayala-Orozco, C. Urban, S. Bishnoi, A. Urban, H. Charron, T. Mitchell, M. Shea, S. Nanda, R. Schiff, N. J. Halas and A. Joshi, J. Controlled Release, 2014, 191, 90–97 CrossRef CAS PubMed.
- J. R. Cole, N. A. Mirin, M. W. Knight, G. P. Goodrich and N. J. Halas, J. Phys. Chem. C, 2009, 113, 12090–12094 CrossRef CAS.
- S. Lal, S. E. Clare and N. J. Halas, Acc. Chem. Res., 2008, 41, 1842–1851 CrossRef CAS PubMed.
- D. P. O'Neal, L. R. Hirsch, N. J. Halas, J. D. Payne and J. L. West, Cancer Lett., 2004, 209, 171–176 CrossRef PubMed.
Footnote |
† Electronic supplementary information (ESI) available. See DOI: 10.1039/c9nh00021f |
|
This journal is © The Royal Society of Chemistry 2019 |