DOI:
10.1039/C8FO02067A
(Paper)
Food Funct., 2019,
10, 432-447
Pharmacodynamic and urinary metabolomics studies on the mechanism of Schisandra polysaccharide in the treatment of Alzheimer's disease†
Received
22nd October 2018
, Accepted 20th December 2018
First published on 24th December 2018
Abstract
Schisandra chinensis (Turcz.) Baill is produced mainly in northeast China, Korea and Japan. Its fruit has been used in food as a nutritional and functional ingredient for centuries. Polysaccharide is an important chemical component in Schisandra. Previous studies have shown that Schisandra polysaccharide (SCP) could be used to improve cognitive function clinically and treat age-related neurodegenerative disorders. In this study, a urinary metabolomics method based on ultra-high-performance liquid chromatography combined with quadrupole-time-of-flight mass spectrometry (UHPLC-Q-TOF-MS) was established to investigate the change of endogenous metabolites in an amyloid β-protein (Aβ) 25–35-induced Alzheimer's disease (AD) rat model. Meanwhile, levels of 9 neurotransmitters were evaluated with ultrahigh-performance liquid chromatography-triple-quadrupole mass spectrometry (UHPLC-TQ-MS) to explore the therapeutic mechanisms of SCP on the AD rat model. Additionally, the synthesis of phosphorylated tau protein (p-tau), acetylcholinesterase (AchE) activity and oxidative damage in the brain of the AD rats were assessed using glycogen synthase kinase-3β (GSK3β), AchE and antioxidant assays, NOS (nitric oxide synthase) and SOD (superoxide dismutase), respectively. The results indicated that the AD model was established successfully and the inducement of Aβ25–35 caused the phosphorylation of tau protein and the deposition of Aβ. In the AD model rats, the levels of AchE, GSK-3β and NOS were significantly elevated and SOD activity was reduced. In the hippocampus of the model rats, the contents of γ-aminobutyric acid, acetylcholine, glycine, norepinephrine, taurine, serotonin and dopamine were significantly decreased and the contents of glutamate and aspartic acid were increased significantly. However, SCP could reduce the degree of phosphorylation of tau protein, the deposition of Aβ and oxidative damage and reverse these changes of neurotransmitters in the AD rats. In a metabolomics study, a total of 38 metabolites were finally identified as potential biomarkers of AD and all of them had a significant recovery compared with the AD model after SCP administration. Metabolomics studies have shown that SCP plays a role in protecting the central nervous system, regulating intestinal microbial metabolism, regulating energy metabolism, and promoting antioxidant effects by regulating the levels of endogenous metabolites in related pathways. This is first report of the use of urine metabolomics combined with the evaluation of 9 neurotransmitter levels to investigate the mechanism of SCP on the treatment of AD.
Introduction
Alzheimer's disease (AD) is a neurodegenerative disease, and there is no clear and effective treatment for it.1 AD can lead to the death of a large number of nerve cells, thereby destroying people's normal memory and thinking ability. Eventually, the patient loses basic self-care ability and physiological function until death.2 The number of people affected by AD is increasing rapidly worldwide and it has become one of the most damaging diseases in society.3 There are many hypotheses about the pathogenesis of AD, including β-amyloid deposition, tau protein phosphorylation, gene mutation, chronic inflammation, oxidative stress, and free radical damage.4 There is currently no drug or clinical method that can completely cure AD. The main therapeutic targets of existing drugs are to improve learning and memory ability and behavioral disorders, slow the progression of the disease, and prevent or delay the occurrence of the disease.
Medicinal plants have been recognized for their various chemical components, synergistic effect by multi-pathway and multi-target mechanisms and their therapeutic advantages in chronic, multi-gene complex diseases.5 In recent years, a large number of studies have reported that medicinal plants have shown their unique advantages in the treatment of AD.6–8 Some polysaccharides, lignans, flavonoids, triterpenes, saponins and alkaloids isolated from medicinal plants have been shown to have potential anti-AD efficacy.9–13Schisandra chinensis (Turcz.) Baill as a medicinal plant has the functions of improving learning and memory, sedation, anti-aging and analgesia on the central nervous system.14–17 Its fruit has been used in food as a nutritional and functional ingredient for centuries. In recent years, there have been many kinds of Schisandra products on the market, such as drinks, fruit vinegar, flavoring agents, food additives and others. The beverages produced from Schisandra berry as a raw material have a high nutritional value, medical function and health care function. More importantly, the fruit itself contains antioxidants, and the product can be stored for a long time without adding preservatives. In addition, the fruit of Schisandra contains anthocyanin, which has a bright carmine color, and it does not require any coloring. Previous studies indicated that the Schisandra polysaccharide (SCP) could improve the learning and memory ability of mice with brain aging.18,19 However, the molecular mechanisms of this effect have not been fully investigated.
Metabolomics aims to study biomarkers associated with specific biochemical events and specific mechanisms of action in order to predict the development mechanism of a disease or the mechanism of action of a drug.20,21 Medicinal plants with complex active ingredients have the characteristics of multi-target holistic action. The metabolomics method is particularly suitable for the study of the holistic mechanism of action of medicinal plants. In AD research, metabolomics is becoming a powerful tool for identifying biomarkers for early diagnosis, exploring disease-related processes, discovering novel therapeutic targets, and monitoring therapeutic responses. Therefore, metabolomics studies for clarifying the mechanism of SCP on AD are necessary. AD pathology is also characterized by the abnormal functioning of different excitatory neurotransmitters and inhibitory neurotransmitters, such as γ-aminobutyric acid (GABA), acetylcholine (Ach), glycine (Gly), adrenaline (E), norepinephrine (NE), taurine (Tau), serotonin (5-HT), dopamine (DA), glutamate (Glu), and aspartic acid (Asp).22,23 It has been reported that cholinergic, GABAergic, serotonergic, glutamatergic, and dopaminergic neurons are disturbed in AD.24–27 Therefore, restoration of neurotransmitter content in the brain should be a feasible treatment strategy in pharmacotherapy of AD.
In this study, rather than searching for pharmacological indicators, an ultra-high-performance liquid chromatography combined with quadrupole-time-of-flight mass spectrometry (UHPLC-Q-TOF-MS)-based metabolomics study combined with multivariate statistical analysis was applied to identify potential biomarkers and estimate the changes in important metabolic networks in urine in AD model rats after treatment with SCP. Ultra-high-performance liquid chromatography-triple-quadrupole mass spectrometry (UHPLC-TQ-MS) using multiple reaction monitoring (MRM) detection allows a rapid, sensitive and specific quantification of multi-analytes in a short chromatographic separation. Therefore, it was used to investigate the influence of SCP on the neurotransmitters of the hippocampus in the rat model of AD.
Materials and methods
Chemicals and reagents
Water was generated by a Milli-Q academic water purification system (Milford, MA, USA). N,N-Dimethylphenylalanine, Aβ25–35, Tau, Glu, Asp, GABA, Gly, DA, 5-HT, NE, Ach, L-phenylalanine, citric acid, uric acid, tyrosol, hippuric acid, p-cresol sulfate, indoxyl sulfate and xanthurenic acid were purchased from Sigma-Aldrich (St Louis, MO, USA). Histostain™-Pluskits, anti-beta amyloid and anti-phospho-tau (Ser235) were bought from Cell Signaling Technology (Boston, USA). HPLC-grade methanol, formic acid and acetonitrile were obtained from Tedia Company (Fairfield, OH, USA). Rat NOS ELISA kits, rat SOD ELISA kits, rat AChE ELISA kits, rat β-secretase ELISA kits, rat GSK-3β ELISA kits and rat γ-secretase ELISA kits were obtained from Nanjing Jiancheng Bioengineering Institute (Nanjing, China).
Preparation of the crude polysaccharide extract
Schisandra chinensis (Turcz.) Baill was purchased from Hebei Kaida Pharmaceutical Co., Ltd. The herb was identified by Prof. Zhiqiang Liu (Changchun Institute of Applied Chemistry, Chinese Academy of Sciences, Changchun, China). It complied with the standards of Chinese Pharmacopoeia 2015. The fruits of the plant were dried at 60 °C for 24 h, then crushed into powder and sieved with a 40-mesh sieve. The powder was extracted twice with 10 °C water in a ratio of 1
:
30 (w/v) for 1.5 h. After centrifugation, the supernatant was concentrated at 50 °C. Then, 95% ethanol was added to the concentrate to yield a final ethanol concentration of 70% and the solution was left to stand for one night. The crude polysaccharide was obtained by precipitation filtration and its yield was 7.0%.
Using D-glucose as the reference substance, the total sugar content was determined with the phenol-sulfuric acid method. The total sugar content of SCP was 58.65%. The protein content of SCP was determined using the Bradford assay and was found to be 2.65%. Additionally, SCP was graded by the membrane filter method, and 6 kinds of polysaccharides with different molecular weights were obtained: <5 × 103, 5 × 103–1 × 104, 1 × 104–1 × 105, 1 × 105–5 × 105, 5 × 105–1 × 106 and >1 × 106. The purity of the polysaccharides in these six grades was 26.94%, 32.53%, 28.70%, 65.95%, 71.84% and 79.60%, respectively. The yields were 34%, 10.02%, 7.11%, 13.22%, 8.83%, and 9.02%, respectively. The monosaccharide composition of SCP was investigated using GC-MS following derivatization with a pyridine and silanization reagent (BSTFA + TMCS). Analysis of the derivatives was carried out by the Thermo Scientific TSQ 8000 triple quadrupole GC-MS/MS system. Separation was achieved using reverse phase elution on a 0.25 μm capillary column TG-SQC (15 m × 0.25 mm, 0.25 μm, Thermo Scientific, USA). The injection volume was 2.0 μL with a 20
:
1 split ratio with an injector temperature of 250 °C, using helium as a carrier gas at a constant flow of 1.0 mL min−1. The following gradient was used: initial oven temperature 60 °C; 120 °C at 10 °C min−1, 5 min; 155 °C at 1.5 °C min−1, 5 min; 171 °C at 0.9 °C min−1, 5 min; 180 °C at 9 °C min−1, 5 min and 300 °C at 10 °C min−1, 10 min. A transfer line temperature of 250 °C was used. The MS conditions in the positive mode were as follows: ion source, 280 °C; mass range 30–600 Da; scan time of 0.35 s. Through comparison with the monosaccharide standards (Fig. S1A†), SCP was found to contain arabinose (Ara), glucose (Glu), galactose (Gal), mannose (Man), ribose (Rib), xylose (Xyl), glucuronic acid (GlcA), rhamnose (Rha) and fucose (Fuc) (Fig. S1B†).
Animals
Forty male Sprague-Dawley rats (6 weeks old, 200–220 g) were purchased from Changsheng Biotechnology Co., Ltd (Dalian, China), with the license number SCXK-liao-2010-0001. The animals were kept in standard cages under a light/dark cycle of 12 h per day (lights on at 7 a.m.), with free access to water and food throughout the study, at a regulated temperature (23 °C ± 2 °C) and relative humidity (50% ± 10%). All animal treatments and experiments were carried out in strict accordance with the recommendations of “Laboratory animals-guideline for ethical review of animal welfare” in the national standards of the People's Republic of China (GB/T 35892-2018). All procedures related to animal experiments were approved by the Institutional Animal Care and Use Committee of Jilin University.
Grouping and treatment
In our experiments, the animals were randomly divided into 4 groups, namely sham operation group (SHO), Alzheimer's disease model group (ADM), positive drug donepezil group (PDD) and Schisandra crude polysaccharide group (SCP). All the rats of the ADM, PDD and SCP groups were anesthetized with 1% pentobarbital sodium and fixed on brain stereotaxic apparatus. Then, the rats were individually injected with 10 μg Aβ25–35 (2 μg μL−1) into each bilateral hippocampus according to the rat brain in stereotaxic coordinates (3.0 mm behind the bregma and 2.0 mm beside the midline; vertically insert the needle to a depth of 2.6 mm below the dura mater). The SHO group was administrated with saline using the same method. All the rats were intramuscularly administrated with penicillin (106 U mL−1) every other day three consecutive times after the intracerebroventricular administration. A week after the animal model establishment, the rats were orally administrated as follows: ADM and SHO were treated with distilled water, PDD treated with 0.91 mg kg−1 donepezil and SCP was treated with 38.15 mg kg−1 day−1 doses (amounting to 545 mg Schisandra chinensis (Turcz.) Baill/kg day−1). All the rats were treated once daily for 56 consecutive days.
Morris water maze test
The Morris water maze (MWM) test was performed to evaluate the learning and memory ability of the rats according to a previously reported method on the 52nd day of SCP administration.22 Thirty minutes after daily administration of SCP, the MWM test was carried out for a total of 5 days. The place navigation test was conducted in the first 4 days and the spatial probe test was performed on the last day.
Sample collection and preparation
The collected 24 h urine samples of the SHO, ADM and SCP groups were diluted 10 times with water and filtered through a 0.22 μm filter membrane before UHPLC-Q-TOF-MS analysis. A 100 μL urine sample of each rat was included as a quality control (QC) sample to validate the system stability.
The rats were sacrificed after the last administration. The brain samples were rapidly removed and placed on dry ice to quickly freeze. The brain was divided into two parts along the midline. One part was stored at −80 °C for the determination of the neurotransmitters and ELISA, and the other part was stored in 4% paraformaldehyde for fixation in pathological and immunohistochemical experiments.
Prior to quantitative analysis of neurotransmitters in the brain using UHPLC-TQ-MS, the brain tissues were homogenized in cold H2O–HCOOH (100
:
0.12, v/v; 1
:
8, w/v) and centrifuged at 12
000 rpm for 20 min.
ELISA of rat NOS, SOD, AChE and GSK-3β was performed after the brain tissues were ground by using a homogenizer in cold physiological saline (1
:
10, w/v) and centrifuged at 3500 rpm (4 °C, 10 min).
To evaluate the cellular abnormalities in the hippocampus, hematoxylin and eosin (H–E) staining was performed as per a previous method.28 Briefly, the sections were soaked in distilled water, and stained for 10 min with hematoxylin. After differentiation with 1% ethanolic hydrochloric acid for 20 seconds, eosin staining was performed for 10 minutes. Dehydration and clearing were performed using ethanol (90%, 95%, 100%) and xylene solutions, respectively. Finally the sections were sealed using neutral gum seal. The brain sections of each group were observed under a light microscope, focusing particularly on the brain pathological morphology of the hippocampal CA1 region.
In this study, streptavidin-peroxidase immunohistochemistry was used to detect the expression of Aβ and p-tau in the hippocampus.29 Brown granular deposits were interpreted as positive areas. A BI2000 Image Analysis System was used to measure the average optical density of protein expression, and statistically analyze the differences in the expression of various proteins in each group.
UHPLC-TQ-MS conditions
Quantitative analysis of neurotransmitters was performed using a Waters ACQUITY UPLC system coupled with a Xevo TQ MS Spectrometer equipped with an ESI ion source in the positive mode operating in multiple-reaction monitoring (MRM) mode. Water containing 0.12% formic acid (A) and methanol containing 70% water (B) were used as the mobile phase. A 10 μL of sample solution was injected into an ASB C18 HPLC column (4.6 mm × 250 mm, 5 mm) at a flow rate of 0.5 mL min−1. The following gradient program was used: 0–3.7 min, 0% B; 3.7–4.2 min, 0–21% B; 4.2–8.2 min, 21–23% B; 8.2–8.7 min, 23–100% B; 8.7–15.7 min, 100% B; 15.7–16 min, 100–0% B and 16–20 min, 0% B.
The parameters in MS analysis were as follows: ion source block temperature, 110 °C; desolvation temperature, 350 °C; nebulizer gas flow rate, 50 L h−1; desolvation gas flow rate, 800 L h−1 and capillary voltage, 2.5 kV.
UHPLC-Q-TOF-MS conditions
The mass spectrometry system was a Q-TOF Mass Spectrometer (SYNAPT G2 High Definition, Waters). Sodium formate and leucine enkephalin were used to establish the mass spectrometer calibration and perform mass correction, respectively. The ESI ion source was used in positive and negative ionization mode. The voltages of capillary and sampling cone were 2.5 kV and 40 V, respectively. The temperature of desolvation and source were 350 °C and 110 °C, respectively. The flow rates of cone and desolvation gas were 50 L h−1 and 600 L h−1, respectively.
An ACQUITY UHPLC BEH C18 column (1.7 μm, 2.1 mm × 50 m; Waters) kept at 35 °C was used for the urine analysis. A 10 μL diluted urine sample was injected into the column with a flow rate of 0.3 mL min−1 for elution. The elution gradient of the mobile phase A (water containing 0.1% formic acid) and B (acetonitrile) was as follows: 5–30% B at 0–5 min, 30–50% B at 5–7 min, 50–100% B at 7–9 min, 100–5% B at 9–9.1 min and then kept at 5% B for 4 min.
Data analysis
The UHPLC-Q-TOF-MS and UHPLC-TQ-MS raw data files were acquired using MassLynx V4.1 for mass spectrometric ion information (retention time, mass-to-charge ratio and peak area normalization). The UHPLC-Q-TOF-MS data was further analyzed by unsupervised PCA analysis, supervised PLS-DA analysis and OPLS-DA analysis with EZinfo software to obtain target statistics. The calibration and quantification of 9 neurotransmitters were performed using TargetLynx V4.1 software. SPSS16.0 was used for statistical analysis between the three study groups.
Results
Effect of Schisandra polysaccharide on the performance of MWM test
The MWM test was used to evaluate the spatial learning and memory ability of the four groups.30 The results of the place navigation test showed that the trajectories of the SHO, PDD and SCP groups were mostly in a toward-type, spending shorter distance to find the platform compared with that of the ADM group, which swam around the wall (Fig. S2A–C†). The escape latency time to find the submerged platform gradually declined as training times increased in the three groups. Compared with the SHO group, the escape latency time of the rats in the model group increased significantly within 4 days (p < 0.01), indicating that the ability of learning and memory of the ADM rats was significantly impaired. After the treatment with SCP the escape latency time was shortened significantly in 4 days (p < 0.01) (Fig. 1).
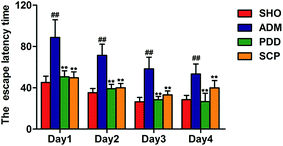 |
| Fig. 1 The escape latency time over four days in the place navigation test. Data are presented as mean ± SEM (n = 10). ## p < 0.01 versus the SHO group. * p < 0.05 and ** p < 0.01 versus the ADM group. | |
In the spatial probe test, the platform was placed in the second quadrant. As shown in Fig. S2D–S2F,† the SHO, PDD and SCP groups swam in the second quadrant of the platform. However, the ADM group swam in all four quadrants randomly. As seen in Fig. 2, compared with the SHO group, the ADM rats had worse performance in crossing time, distance and time spent in the correct regions (p < 0.01). After treatment with SCP and donepezil for 8 weeks, the performances of the SCP and PDD rats in terms of these parameters were significantly improved (p < 0.05 or p < 0.01).
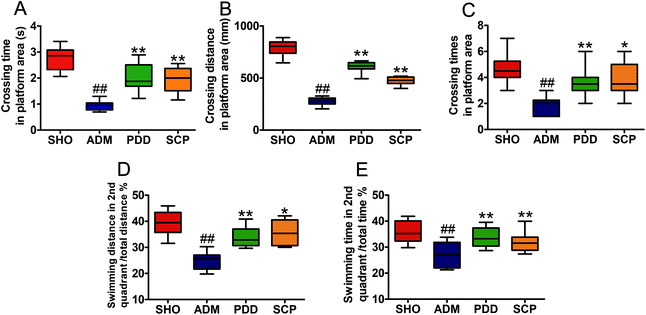 |
| Fig. 2 The effect of SCP on the behavior in the spatial probe test of AD rats. Data are presented as mean ± SEM (n = 10). # p < 0.05 and ## p < 0.01 versus the SHO group. * p < 0.05 and ** p < 0.01 versus the ADM group. | |
The place navigation test was conducted to examine the spatial learning ability of the rats, while the spatial probe test examined the spatial memory ability of the rats. These results suggested that SCPs could modulate the impairment of learning and memory induced by Aβ25–35 in AD rats.
Effect of Schisandra polysaccharide on hippocampal neuronal morphology
Fig. 3A shows that the hippocampal neurons in the CA1 region were plump and well-arranged with clear cell structure, clear nuclei and cytoplasm in the SHO group, and no obvious abnormalities were found. By contrast, as shown in Fig. 3B, in the ADM group, the cells in the hippocampus were sparse and disorganized, the pyramidal cell layer was incomplete, the cell bodies were reduced in number, the cytoplasm was reddish, and the nucleus was pyknotic and deeply stained. Compared with the model group, the structure of the hippocampal neurons in the PDD and SCP group changed significantly (Fig. 3C and D). The number of normal nerve cells (/HP) in the CA1 region of the AD rats was obviously (p < 0.01) less than that in SHO. However, the number increased significantly (p < 0.05) in PDD and SCP compared with ADM (Fig. 3E). These results revealed that the damage to hippocampal neurons was repaired in the AD rats after treatment with SCP.
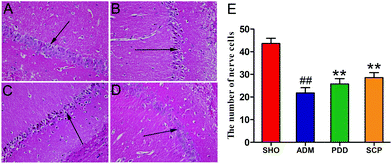 |
| Fig. 3 The number of nerve cells (e) and H–E staining of pathologic changes in the CA1 region of rat hippocampi in the SHO (a), ADM (b), PDD (c) and SCP (d) groups (magnification: 400×, n = 10 in each group, ## p < 0.01 versus the SHO group, ** p < 0.01 versus the ADM group). | |
Effect of Schisandra polysaccharide on Aβ and p-tau expressions
The two major pathological features of AD are the senile plaques and neurofibrillary tangles formed by the extracellular Aβ deposition and the hyperphosphorylation of tau protein in the cells, respectively.31 The deposition of Aβ is a major cause of neuronal loss and brain death. After hyperphosphorylation of tau protein in the brain of AD patients, polymerization occurs to form double-stranded spiral filaments, which in turn form insoluble neurofibrillary tangles.32 These neurofibrillary tangles are neurotoxic. They can deplete soluble tau protein and block neuronal trafficking pathways, further aggravating the disease.
The expressions of Aβ and p-tau in the hippocampus were determined by immunohistochemistry. As seen in Fig. 4, the accumulation and the optical density of Aβ in the ADM group was significant higher than that in the SHO group (p < 0.01). However, the SCP and PDD group showed obviously decreased Aβ expression (p < 0.01). No significant expression of p-tau was observed in the SHO group, and the average optical density was low. P-tau was strongly expressed in the ADM group, as indicated by a dark brown color. Moreover, the quantity of p-tau was significantly increased in the ADM group, and the average optical density was greater than that of the SHO group (p < 0.01). In the SCP and PDD group, the positive expression of p-tau was decreased, and the average optical density was significantly reduced compared with the ADM group (p < 0.01).
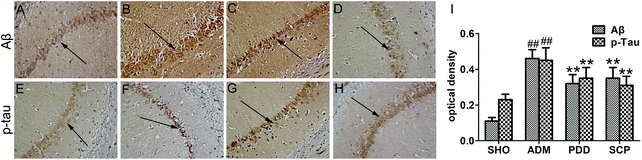 |
| Fig. 4 The expression of aβ and p-tau in the hippocampi of rats. a–d: The aβ expression of the SHO, ADM, PDD and SCP groups; e–h: the p-tau expression of SHO, ADM, PDD and SCP groups; and i: the average optical density of the expression of aβ and p-tau. ## p < 0.01 versus the SHO group. ** p < 0.01 versus the ADM group. | |
The pathological studies herein showed that Aβ deposition and p-tau were present in the brain of Aβ25–35 induced AD rats, indicating that the AD model established by this method was successful. After treatment with SCPs, the Aβ formation and tau protein phosphorylation of the AD model were significantly reduced, suggesting that SCP has a measurable therapeutic effect on AD.
Effect of Schisandra polysaccharide on the level of NOS, SOD, GSK-3β and AChE in the hippocampus
In this study, the activities of SOD, GSK-3β, NOS and AchE in the hippocampus were evaluated to elucidate the effect of Aβ25–35 injection on the antioxidant status, p-tau and activity of AchE within the brain and the therapeutic effect of SCP. As shown in Table 1, compared with the SHO group, the model group exhibited increased NOS, GSK-3β and AChE levels and decreased SOD activity in the hippocampus (p < 0.01). Compared with the model rats, after treatment, SCP reduced the level of hippocampal NOS, GSK-3β, AChE and increased SOD activity (p < 0.01 or p < 0.05). All these findings suggested that SCP might relieve oxidative stress and inhibit the activity of AChE and GSK-3β in AD rats and thus play a potential prevention and treatment role in AD.
Table 1 Effect of SCP on NOS, SOD, GSK-3β and AChE in hippocampus of AD rats. Data are presented as mean ± SEM (n = 10). ##P < 0.01 versus the sham operation group. **P < 0.01 versus the model group
Group |
NOS (nmol g protein−1) |
SOD (U g protein−1) |
GSK-3β (ng g protein−1) |
AChE (nmol g protein−1) |
SHO |
65.72 ± 4.51** |
293.38 ± 24.11** |
10.68 ± 0.89** |
0.254 ± 0.012** |
ADM |
76.88 ± 3.45## |
179.45 ± 19.14## |
15.12 ± 0.99## |
0.309 ± 0.029## |
SCP |
68.00 ± 3.52** |
269.90 ± 14.18** |
12.48 ± 1.97* |
0.263 ± 0.013** |
Effect of Schisandra polysaccharide on neurotransmitter levels
In this study, the contents of 9 neuroactive substances in the rat hippocampus were determined (Fig. 5). The results showed that the contents of Glu and Asp in the hippocampus of the ADM animals were significantly increased (p < 0.05 or p < 0.01), and the contents of 5-HT, NE, DA, Ach, Tau, GABA and Gly were significantly decreased (p < 0.01). This suggested that excitatory amino acids (Glu and Asp), inhibitory amino acids (GABA and Gly) and related neurotransmitters (5-HT, NE, DA, Ach and Tau) all participate in the pathogenesis of AD. After treatment with SCP, the levels of the 9 neuroactive substances in the hippocampus of the AD rats were adjusted to normal levels (p < 0.01 or p < 0.05). This indicated that SCP may protect the central nervous system function by regulating the content of neuroactive substances in the brain of AD rats, thus improving the learning and memory ability of AD rats.
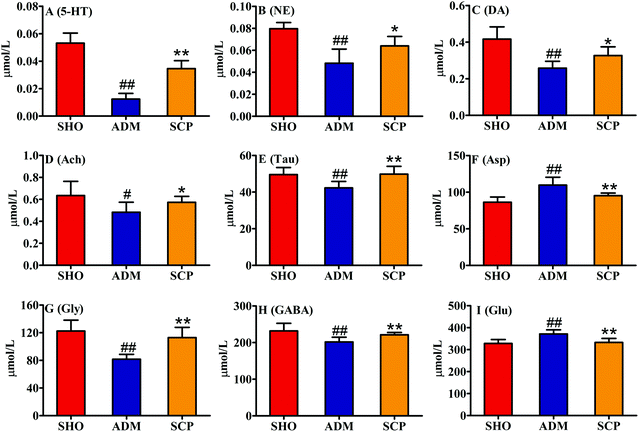 |
| Fig. 5 The levels of 9 neurotransmitters in the rat hippocampi. # p < 0.05 and ## p < 0.01 versus the SHO group. * p < 0.05 and ** p < 0.01 versus the ADM group. | |
UHPLC-Q-TOF-MS method of validation
The information of 16 ions in the QC group were selected to verify the stability of the system, as follows: 0.82–229.1554, 1.29–297.1450, 2.31–338.0876, 3.26–130.0661, 5.19–475.2473, 6.36–375.1189, 6.36–375.1189, 8.32–357.2794 in positive mode and 0.95–227.968, 1.53–172.9915, 2.175–178.0504, 3.10–283.0821, 4.17–297.0977, 4.88–201.0223, 5.77–329.1603, 7.14–212.0025 in negative mode, respectively. The relative standard deviations of retention time and peak area of these 16 ions are 0.3–1.6% and 1.1–7.5% respectively. The results showed that chromatographic separation and mass spectrometry were stable and reproducible during the analysis.
Identification of potential biomarkers
The collected UHPLC-Q-TOF-MS data was imported into MarkerLynx software for processing. Potential biomarkers were analyzed using the multivariate analysis methods of PCA, PLS-DA and OPLS-DA. As shown in Fig. 6, each point on the PCA score map represented a sample, and the position of each sample was determined by the type and content of its metabolites. Samples in a similar location tend to have similar metabolite compositions. The urine samples of the ADM, SHO and SCP rats had clearer boundaries in the Score plots with no crossover or overlap. The distances between SHO and SCP were short. This result showed that the metabolic profile in the urine of the ADM rats significantly changed compared with the SHO animals. After SCP treatment, the urine metabolic profile of the AD rats shifted toward the SHO group. The OPLS-DA analysis was performed in the ADM and SCP groups to identify potential markers of SCP for AD. Compounds with a VIP > 1 and an independent t-test with p < 0.05 were initially screened as potential biomarkers. Finally, the biomarkers were determined by comparing their molecular weight and tandem mass spectra with databases (KEGG, HMDB and METLIN) or standard products. 38 potential biomarkers identified in the SCP treatment group compared to the AD model group are listed in Table S1.† To compare the relative amounts of potential biomarkers in each group, the MetaboAnalyst online metabolomics tool was used to convert the urine sample biomarker content into a visualized heatmap (Fig. 7). From the heatmap, it can be seen that compared with the ADM group, 23 endogenous metabolites were up-regulated and 15 endogenous metabolites were down-regulated after administrating SCP. Additionally, clustering analysis grouped SCP and SHO together, which indicated that their metabolic profiles were similar. The metabolic pathways affected by SCP were obtained through MetPA (http://metpa.metabolomics.ca/), and included the metabolic pathways of tryptophan, tyrosine, phenylalanine, taurine and hypotaurine, arginine and proline, purine and pyrimidine, nicotinate and nicotinamide and lysine (Fig. 8). The changes of neurotransmitters and their metabolites in the AD rats are shown in Fig. S3.†
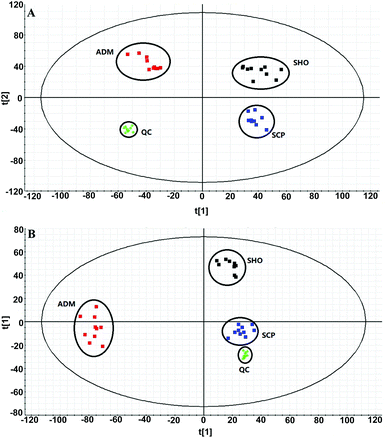 |
| Fig. 6 PCA score plots derived from the urinary metabolic profiles of the SHO group (black), ADM group (red), and SCP group (blue) and QC samples (green) in positive mode (a) and negative mode (b). | |
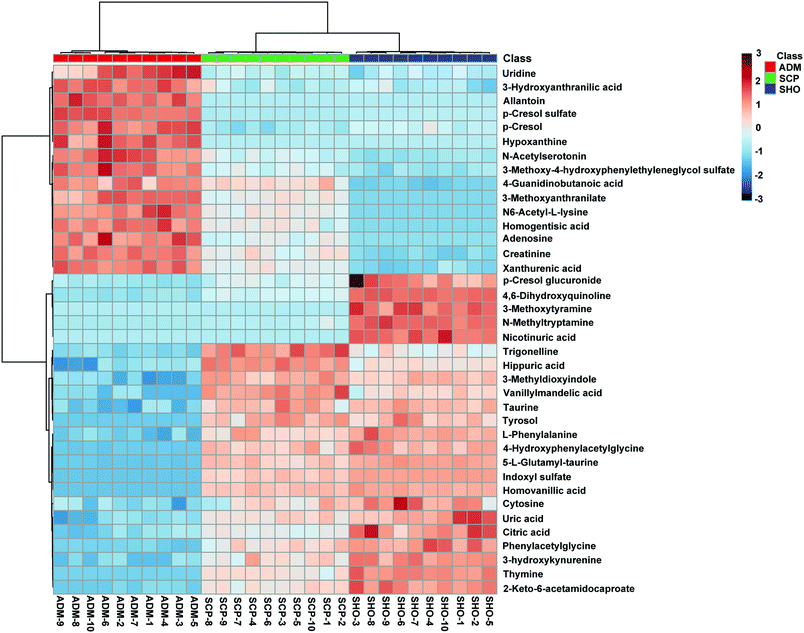 |
| Fig. 7 A heatmap visualizing the intensities of potential biomarkers in the ADM group, SCP group and SHO group. Increasing expression values are coded with blue to red colors. Rows indicate potential biomarkers and columns indicate samples. | |
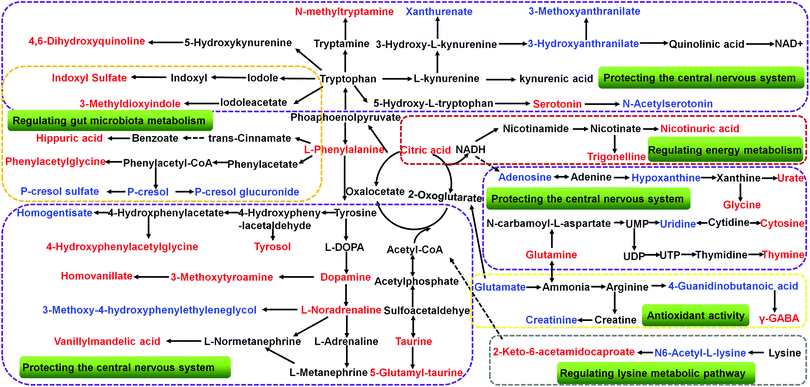 |
| Fig. 8 The regulation of SCP and endogenous metabolite disturbance in the urine of AD rats; the metabolites marked in red denote increased potential biomarkers, and those in blue denote decreased ones. The related mechanisms are shown in the green boxes. | |
Discussion
In this study, an AD model induced by Aβ25–35 injection in the rat hippocampal CA1 region was selected. The results of behavioral experiments (MWM test) showed that SCP could improve the symptoms of learning and memory impairment in AD rats induced by Aβ25–35. The pathological experimental results of HE staining and immunohistochemistry showed that SCP could reduce deposition of Aβ and expression of p-tau in the hippocampus of Aβ25–35-induced AD rats. In order to further explore the mechanism of SCP on AD, the activity of AchE, GSK-3β, NOS and SOD in the brain of the AD rats was determined. Biochemical analysis showed that SCP is able to attenuate hippocampal AChE and GSK-3β activity and exhibit antioxidant activity. In addition, the UHPLC-TQ-MS method was used to determine the content of 9 neuroactive substances in the hippocampus. The contents of Glu and Asp in the hippocampus of the SCP animals were significantly decreased, and the contents of 5-HT, NE, DA, Ach, Tau, GABA and Gly were significantly increased. Finally, UHPLC-Q-TOF-MS combined with PCA analysis was performed to study the urine metabolomics of AD rats treated with SCP. 38 endogenous metabolites in rat urine were identified as biomarkers for the treatment of AD by SCP. The above results were analyzed comprehensively and the mechanism of SCP in the treatment of AD is as follows.
SCP treats AD by protecting the central nervous system
The differences in levels of neurotransmitters and their metabolites between a sham-operated group and AD model group have been studied in some earlier works.33,34 Neurotransmitters, consisting of amino acid neurotransmitters and monoamine neurotransmitters, play important roles in various activities of the peripheral and central nervous systems.
Acetylcholine is a signal transmitter of cholinergic neurons. The signals it conducts are related to learning, cognition and memory, and its metabolic processes are closely related to AD, so it has been widely studied. The cholinergic theory of AD states that the number of cholinergic neurons in the brain is reduced, and the synthesis and release of Ach are reduced, leading to impaired memory and recognition in patients.35 Studies have shown that 90% of AD patients lack Ach during the transmission of nerve impulses, so one way to alleviate the condition is to take a drug that inhibits the breakdown of Ach by AchE, restoring normal levels of Ach.36 In this study, the content of Ach in the brain of the ADM rats was significantly reduced (p < 0.05) and the level of AchE was significantly increased (p < 0.01). SCP significantly corrected the content of Ach (p < 0.05) and AchE (p < 0.01) in the hippocampus, indicating that SCP can promote the release of Ach by inhibiting the activity of AchE.
The mechanism of learning and memory is not only related to the central cholinergic nervous system, but also closely related to the monoamine neurotransmitters NE, DA, and 5-HT. Tyrosine is a nonessential amino acid produced by hydroxylation of phenylalanine in humans and animals. It is a precursor of the monoamine neurotransmitters such as adrenaline, NE and DA. DA indirectly affects memory by regulating mental activity, emotion, recognition, thinking and reasoning through mesolimbic, mesocortical, nigrostriatal and tuberoinfundibular pathways.37,38 There is a close relationship between the progression of AD and the locus coeruleus, which is the main site for the synthesis of NE in the brain.39 Recent studies have found that NE is not only a classical neurotransmitter, but also an endogenous anti-inflammatory factor that has immunomodulatory and anti-inflammatory effects that can inhibit the activation of inflammatory mediators in astrocytes and microglia.40 Decreased levels of NE can cause neurotoxicity and pro-inflammatory symptoms, reduce Aβ clearance and negatively affect cognitive and memory function in AD patients.41 5-HT is also an important monoamine neurotransmitter closely related to learning and cognitive ability, and has a close relationship with degenerative changes in the brain. These monoamine neurotransmitters were also highlighted as molecular targets involved in the treatments of AD.42–44 In the 5-HT metabolic pathway, 5-HT is converted to N-acetylserotonin by N-acetyltransferase. N-Acetylserotonin has been found to exhibit antiaging and antioxidant activities, as well as to protect against Aβ-induced neurotoxicity and improve cognition.45,46 3-Methoxy-4-hydroxyphenylethyleneglycol sulfate, homovanillic acid, vanillylmandelic acid and 3-methoxytyramine are all metabolites of the dopamine metabolic pathway system. In this study, the levels of NE, DA, and 5-HT in the ADM group were decreased significantly (p < 0.01), indicating that learning and memory impairment in the MWM test of AD rats may be related to the decrease of monoamine neurotransmitters. It was also shown (Fig. 7) that the uric contents of 3-methoxy-4-hydroxyphenylethyleneglycol sulfate and N-acetylserotonin in the ADM group were significantly higher than that in the SHO group, whereas the urine level of homovanillic acid, vanillylmandelic acid and 3-methoxytyramine in the ADM group appeared to be lower than in the SHO group. SCP revised the levels of these endogenous metabolites in the AD rats (p < 0.05), which indicates that SCP may enhance the learning and memory ability of AD rats by regulating the level of monoamine neurotransmitters and their metabolites.
The main inhibitory amino acid neurotransmitter in the nervous system, GABA, is also thought to be involved in cognitive function.47 GABA plays an important role in maintaining the balance of brain excitement and inhibiting nerve conduction. Autopsy and animal model studies have shown that GABAergic system dysfunction exists in the pathological process of AD.48 4-Guanidinobutanoate is the precursor of GABA. In patients with arginase deficiency, elevated levels of 4-guanidinobutanoate caused a variety of clinical syndromes such as mental retardation, neurodevelopmental arrest and epilepsy.49 In the present study, SCP reduced the content of 4-guanidinobutanoate (Fig. 7) in the urine of the AD rats and increased the GABA content in the hippocampus (Fig. 5), indicating that SCP can promote the synthesis of GABA and reduce the damage to the nervous system caused by hyperammonemia, thereby exerting neuroprotective effects.
Gly, like GABA, is also an inhibitory neurotransmitter. Studies have shown that long-term exposure to aluminum can cause learning and memory disorder, cognitive dysfunction and other dementia symptoms. The contents of inhibitory amino acid neurotransmitters (Gly and GABA) in the brains of rats exposed to aluminum were decreased.50 The results in Fig. 5 showed that the SCP treatment significantly increased the contents of Gly and GABA in the hippocampus of the AD rats (p < 0.01), indicating that SCP has a significant protective effect on the central nervous system.
Glu and Asp are two major excitatory amino acid neurotransmitters that play an important role in the transmission of excitatory information in the nervous system. They are associated with the survival, synapse formation and plasticity of neurons.51 Glu and Asp, the excitatory amino acids released from the presynaptic membrane, selectively bind to G protein-mediated glutamate receptors and N-methyl-D-aspartate (NMDA) receptors, causing Ca2+ channels to open. This increases the influx of Ca2+ in the postsynaptic membrane and participates in the generation and maintenance of long-term potentiation (LTP) of synaptic transmission. LTP has been recognized as an indicator of physiological activity of neurons during memory formation and consolidation. The contents of Glu and Asp in the hippocampus of the ADM group were significantly increased (p < 0.01), while SCP significantly decreased their content (p < 0.01). The results showed that SCP could inhibit the synthesis of excitatory amino acids in the brain, thereby exerting a protective effect on nerve cells.
Taurine is a conditionally essential amino acid that is specifically distributed in the cerebral cortex, hippocampus and cerebellum.52 In the central nervous system, taurine as the second most abundant endogenous amino acid plays a role in neuromodulation,53 osmoregulation,54 the maintenance of calcium homeostasis,55 membrane stabilization,56 anti-oxidant function,57 anti-inflammatory processes58 and neuroprotection.59 Taurine has also shown neuroprotective effects in many pathological conditions such as Alzheimer's and Huntington's diseases.60,61 During the progression of AD, Aβ monomers aggregate into neurotoxic soluble oligomeric Aβ, which causes cognitive impairments.62 It has been reported that the content of taurine in the brain of AD patients was reduced, and taurine could inhibit the accumulation of Aβ.63 Previous studies reported that oral administration of taurine recovered cognitive deficits in the APPswe/PS1dE9 transgenic AD mouse model by directly binding to oligomeric Aβ.64,65 Moreover, taurine has a significant effect on the intelligence development of rats.66 Injecting taurine in vivo can increase the content of acetylcholine in the brain, and the long-term use of taurine has no toxic or other side effects.67 In this study, the content of taurine in the hippocampus of the AD rats was significantly decreased, and the content of taurine was increased to normal levels after administration of SCP (Fig. 5). 5-L-Glutamyl-taurine is produced from taurine via the enzyme γ-glutamyltranspeptidase. The urinary concentrations of 5-L-glutamyl-taurine and taurine were decreased in the AD rats and significantly increased after SCP treatment. This result indicated that SCP could inhibit the accumulation of Aβ protein by regulating taurine metabolism.
Adenosine is responsible for regulating, integrating and fine-tuning neuronal activity, and affecting related brain functions, including sleep and arousal, cognition and memory, and neuronal damage and degeneration.68 Uridine as a precursor for membrane phospholipid biosynthesis69 has been shown to increase the number of brain synapses and improve learning and memory in the long term by reducing apoptotic cell death in the early newborn period.70 The levels of adenosine and uridine were significantly increased in ADM compared with SHO, and their levels were much lower in SCP than ADM. The restoration to healthy levels of adenosine and uridine may imply that SCP could improve the learning and memory of AD rats by affecting the structure and function of neurons.
Tryptophan is an essential amino acid in the human body and is involved in the synthesis of proteins and some important active substances (serotonin, nicotinamide adenine dinucleotide and nicotinic acid).71 99% of tryptophan not used for protein synthesis is metabolized by the kynurenine pathway. Imbalances in the kynurenine pathway have been related with AD.72 The kynurenine pathway consists of two branches that result in the formation of xanthurenic acid and kynurenic acid or the production of 3-hydroxyanthranilate and 3-methoxyanthranilate. Kynurenic acid as the N-methyl-D-aspartate receptor antagonist is thought to be neuroprotective,73 while metabolites of the other branch, including 3-hydroxyanthranilic acid74 and 3-methoxyanthranilate,75 are considered to be neurotoxic. The accumulation of 3-hydroxyanthranilic acid and 3-methoxyanthranilate may lead to apoptosis of astrocytes and certain nerve cells, thereby weakening the work of microglia-neural networks, reducing the synthesis of neurotrophic factors, and making the entire nervous system suffer from damage.76 SCP reduced the content of 3-hydroxyanthranilate and 3-methoxyanthranilate in the urine of the AD rats, suggesting that SCP can protect nerve cells by reducing neurotoxic substances.
SCP treats AD by regulating gut microbiota metabolism
p-Cresol is a metabolite of aromatic amino acid metabolism produced by intestinal microflora. It has corrosive effects on the eyes, skin and respiratory tract and has toxic side effects on the central nervous system, cardiovascular system, lungs and liver. When it passes through the liver and the intestinal mucosa, p-cresol is detoxified by glucuronidation and sulfation generating p-cresol glucuronide and p-cresol sulfate, respectively.77 Clinical studies show that p-cresol sulfate is strongly associated with cardiovascular risk and mortality.78,79 There is growing evidence that cardiovascular risk factors affect not only heart and cerebrovascular disease, but cognition as well.80–82 Cardiovascular risk factors play a pivotal role in the etiology of AD. They may affect the AD process though direct effects on β-amyloid deposition.83 In this study, the levels of p-cresol sulfate increased significantly in ADM compared to SHO and were down-regulated after treatment with SCP. This reveals that SCP can effectively reduce cardiovascular risk to inhibit the occurrence of AD.
Tryptophan is metabolized through two major pathways, the kynurenine pathway and the methoxyindole pathway. As can be seen from the metabolic disturbance map (Fig. 8), 3-methyldioxyindole and indole sulfate are the metabolites of tryptophan via the methoxystilbene pathway. Indoles are the main fermentation product of tryptophan by intestinal bacteria. It has been reported that indole metabolites are able to modify neuronal function and animal behavior by interacting with voltage-dependent Na+ channels.84 Hippuric acid and phenylacetylglycine are also the products of amino acids transformed by intestinal microorganisms. As a series of products of intestinal flora metabolism, the contents of hippuric acid, phenylacetylglycine, 3-methyldioxyindole, indole sulfate, p-cresol, p-cresol sulfate and p-cresol glucuronide were changed in the urine of the ADM rats. After SCP treatment, their content was corrected, indicating that SCP has a therapeutic effect on AD-induced intestinal metabolic disorders.
SCP treats AD by regulating energy metabolism
Energy metabolism plays an important role in the pathogenesis of cognitive disorders. Improving energy metabolism in humans and animals with AD can effectively prevent or reverse cognitive impairment and reduce brain atrophy.85–87 The brain is the most active and vigorous organ of energy metabolism in the body. It is also the organ with the highest blood supply in the body, accounting for 15% to 20% of the cardiac output, and brain energy accounts for about 20% of the body's energy consumption.88 Since there is neither a reserve of energy nor a reserve of raw material for energy production, almost all of the energy usage in the brain depends on the supply of blood. The biochemical metabolism of the brain determines that it must rely on continuous aerobic oxidation of glucose to maintain normal metabolic activity. Modern research has shown that the occurrence and development of AD is associated with glucose metabolism disorders.89
The Krebs cycle refers to the process of oxidative decarboxylation of pyruvic acid produced by glycolysis to form acetyl-CoA in the presence of oxygen, and the acetyl-CoA undergoes a series of oxidation and decarboxylation to finally form CO2, H2O and adenosine triphosphate (ATP). The Krebs cycle promotes the further metabolism of sugar, fatty acid and partial amino acid metabolism substrates, and it is the main way for the body to obtain energy and the center of energy metabolism. The change in the amount of intermediate products can intuitively reflect the changing trend of energy metabolism. SCP significantly up-regulated the citric acid level in AD rats, suggesting that SCP may increase the body's energy metabolism by promoting the Krebs cycle.
Nicotinate is a water-soluble vitamin that belongs to the B vitamins and plays a very important role in the metabolism of carbohydrates, fats and proteins. Nicotinamide as an aminated derivative of nicotinate is the precursor of coenzyme I (NAD) and coenzyme II (NADP), which are the coenzymes of hundreds of enzymes and play an important role in maintaining the normal life activities of cells, including participation in energy metabolism, DNA repair, aging and oxidative stress reactions.90 In the nicotinate and nicotinamide metabolic pathway, nicotinuric acid and trigonelline were the related metabolites that significantly differentiated between the ADM group and SCP group. Nicotinuric acid is the major catabolic product of nicotinic acid and a minor metabolite of fatty acid beta-oxidation. It is involved in nicotinate and nicotinamide metabolism as an endogenous end product, participating in glycolysis, gluconeogenesis and the citric acid cycle.91 Trigonelline, also known as N-methylnicotinate, is a byproduct of niacin metabolism.92 It helps to regenerate glutathione that has been depleted by oxidative stress in trans-sulfuration pathways. From our results, the increase of nicotinuric acid and trigonelline in the urine of SCP rats suggests that SCP is able to improve the nicotinate and nicotinamide metabolism and indirectly promote the biological synthesis of NAD+.
SCP treats AD by exhibiting significant antioxidant activity
Mitochondrial damage and oxidative stress have attracted increasing attention in the occurrence and development of AD. Oxidative stress has been shown to be an important cause of nerve cell damage. The combination of reactive oxygen species (ROS) with lipid and protein on the biofilm destroys the stability and fluidity of the membrane, leading to tissue and cell necrosis or apoptosis. ROS binding to DNA and RNA changes protein translation, transcription and destroys neuron metabolism.93,94 In addition, ROS can also inhibit enzyme complexes in the mitochondrial electron transport chain, leading to mitochondrial dysfunction. In vitro studies have shown that ROS can open calcium ion channels, causing a large number of calcium ions to stimulate over-activation of the calcium-dependent and calmodulin-dependent protein kinase, further unbalancing the ratio of protein kinase to protein phosphatase, thus promoting the abnormal phosphorylation of tau protein.95 In the brain of AD patients, excessive Aβ deposition stimulates the secretion of ROS and other influential factors in glial cells, which leads to the oxidative stress response, while 4-hydroxynonenal, a representative of oxidative stress, can stimulate the production of Aβ and form feedback to further accelerate the pathogenesis of AD.96
Oxidative stress is one of the important mechanisms of Aβ-induced neuronal toxicity.97 SOD is an important enzyme that protects the body against damage and destruction of oxygen free radicals and plays a crucial role in the balance between oxidation and antioxidants. The normal concentration of nitric oxide (NO) in the brain plays a physiological role in the transmission of information as a neurotransmitter. However, high concentrations of NO are mainly manifested as cytotoxic effects leading to nerve cell damage. Because of the short half-life of NO, direct detection is difficult. One of the key enzymes for NO synthesis is NOS, so the NO content is usually analyzed experimentally by detecting NOS activity. The level of NOS in the hippocampus of the ADM rats was significantly increased compared with the SHO rats, and significantly decreased after administration of SCP, while SOD showed the opposite trend. These changes indicated that SCP exhibited significant antioxidant activity.
The arginine and proline metabolism is an important metabolic pathway associated with neurodegenerative diseases,98–100 and arginine and proline have been proposed as potential biomarkers of AD recently.101 Creatinine and 4-guanidinobutanoic acid are the downstream metabolites of the arginine and proline metabolic pathways. In general, the excretion of urinary creatinine is associated with the change of total muscle mass, nutritional status and glomerular filtration rate.102,103 4-Guanidinobutanoic acid is formed by the transfer of the guanidine group from arginine to GABA via transamination.104 The creatinine and 4-guanidinobutanoic acid contents in urine were both increased, indicating that excessive activation of arginine and proline metabolism was caused by AD. Arginine is an indispensable component for synthesis of nitric oxide (NO),105 and NO signaling improves synaptic plasticity in AD.106 It has been reported that excessive NO produced by inducible nitric oxide synthase (iNOS) leads to increased expression levels of Aβ production-associated proteins, including APP and BACE1 genes.107 In our study (Table 1), compared with the SHO group, the ADM group exhibited increased NOS activity in the hippocampus (p < 0.01), indicating that excessive activation of arginine and proline metabolism produced excessive NO in AD rats. The level of creatinine, 4-guanidinobutanoic acid and NOS of the AD rats significantly changed after SCP treatment, indicating that SCP could correct the production of NO by regulating arginine and proline metabolic pathways in Aβ25–35 induced AD rats.
Others
Children with disorders of lysine metabolism display dysplasia of the central nervous system, retarded mental development, slow reactions, low intelligence, poor awareness, slow growth, short stature, light weight, slow and unstable walking, and sometimes the inability to walk even after the age of 1 year.108 Lysine catabolism was clearly altered in the AD rats as demonstrated by the increase of N6-acetyl-L-lysine and decrease of 2-keto-6-acetamidocaproate. N6-Acetyl-L-lysine is an acetylated amino acid that has critical roles in regulating gene transcription, cell-cycle progression, apoptosis, DNA repair and cytoskeletal organization. 2-Keto-6-acetamidocaproate is an intermediate in lysine degradation that inhibits inflammation via the NF-κB signaling pathway.109 After treatment with SCP in the AD rats, the levels of N6-acetyl-L-lysine and 2-keto-6-acetamidocaproate in urine were reversed, indicating that SCP can improve the disturbance of the lysine metabolic pathway in AD rats.
Conclusions
In this study, an AD rat model was established by injection of Aβ25–35 into the bilateral hippocampus. The results of the Morris water maze behavior experiment showed that SCP significantly improved the memory acquisition ability and reversed the memory consolidation disorder of the AD rats. Pharmacodynamic and pathology studies showed that SCP enhanced the learning and memory ability of the AD rats by inhibiting Aβ formation, tau protein phosphorylation and anti-oxidative damage. UHPLC-TQ-MS was used to determine the content of 9 neurotransmitters in the hippocampus and UHPLC-Q-TOF-MS combined with PCA analysis was used to study the urine metabolomics of the AD rats. The changes of neurotransmitters and metabolomic indicators showed that SCP plays a role in treating AD by regulating the central nervous system, gut microbiota metabolism, and energy metabolism and promoting anti-oxidants.
Conflicts of interest
The authors declare no conflicts of interest.
Acknowledgements
This research was supported by the National Natural Science Foundation of China (No. 31670356 and 81873193).
References
- W. W. Barker, C. A. Luis, A. Kashuba, M. Luis, D. G. Harwood, D. Loewenstein, C. Waters, P. Jimison, E. Shepherd and S. Sevush, Relative frequencies of Alzheimer disease, Lewy body, vascular and frontotemporal dementia, and hippocampal sclerosis in the State of Florida Brain Bank, Alzheimer Dis. Assoc. Disord., 2002, 16, 203–212 CrossRef PubMed.
- R. S. Wilson, E. Segawa, P. A. Boyle, S. E. Anagnos, L. P. Hizel and D. A. Bennett, The Natural History of Cognitive Decline in Alzheimer's Disease, Psychol. Aging, 2012, 27, 1008–1017 Search PubMed.
- Y. Huang and L. Mucke, Alzheimer Mechanisms and Therapeutic Strategies, Cell, 2012, 148, 1204–1222 CrossRef CAS PubMed.
- B. J. Hock, K. M. Lattal, L. S. Kulnane, T. Abel and B. T. Lamb, Pathology associated memory deficits in Swedish mutant genome-based amyloid precursor protein transgenic mice, Curr. Aging Sci., 2009, 2, 205–213 CrossRef CAS PubMed.
- L. Wang, G. B. Zhou, P. Liu, J. H. Song, Y. Liang, X. J. Yan, F. Xu, B. S. Wang, J. H. Mao and Z. X. Shen, Dissection of mechanisms of Chinese medicinal formula Realgar-Indigo naturalis as an effective treatment for promyelocytic leukemia, Proc. Natl. Acad. Sci. U. S. A., 2008, 105, 4826–4831 CrossRef CAS PubMed.
- E. K. Perry, A. T. Pickering, W. W. Wang, P. J. Houghton and N. S. L. Perry, Medicinal plants and alzheimer's disease: from ethnobotany to phytotherapy, J. Pharm. Pharmacol., 2010, 51, 527–534 CrossRef.
- R. V. Rao, O. Descamps, V. John and D. E. Bredesen, Ayurvedic medicinal plants for Alzheimer's disease: a review, Alzheimers Res. Ther., 2012, 4, 1–9 Search PubMed.
- N. Chomchalow, Curing Incurable Alzheimer's disease with Medicinal Plants, Assumption University Institutional Repository, 2013, 16, 215–220 Search PubMed.
- S. Huang, J. Mao, K. Ding, Y. Zhou, X. Zeng, W. Yang, P. Wang, C. Zhao, J. Yao and P. Xia, Polysaccharides fromGanoderma lucidumPromote Cognitive Function and Neural Progenitor Proliferation in Mouse Model of Alzheimer's Disease, Stem Cell Rep., 2017, 8, 84–94 CrossRef CAS PubMed.
- Q. Ruan, J. Ruan, W. Zhang, F. Qian and Z. Yu, Targeting NAD+ degradation: The therapeutic potential of flavonoids for Alzheimer's disease and cognitive frailty, Pharmacol. Res., 2017, 128, 345–358 CrossRef PubMed.
- Y. J. Kang, D. G. Seo and S. Y. Park, Phenylpropanoids from cinnamon bark reduced β-amyloid production by the inhibition of β-secretase in Chinese hamster ovarian cells stably expressing amyloid precursor protein, Nutr. Res., 2016, 36, 1277–1284 CrossRef CAS PubMed.
- X. F. Ji, T. Y. Chi, P. Liu, L. Y. Li, J. K. Xu, Q. Xu, L. B. Zou and D. L. Meng, The total triterpenoid saponins of Xanthoceras sorbifolia improve learning and memory impairments through against oxidative stress and synaptic damage, Phytomedicine, 2017, 25, 15–24 CrossRef CAS PubMed.
- S. Dall, Acqua, Plant-derived acetylcholinesterase inhibitory alkaloids for the treatment of Alzheimer's disease, Bot.: Targets Ther., 2012, 2013, 19–28 Search PubMed.
- D. S. Kim, J. Y. Kim and Y. S. Han, Alzheimer's disease drug discovery from herbs: neuroprotectivity from beta-amyloid (1–42) insult, J. Altern. Complement. Med., 2007, 13, 333–340 CrossRef PubMed.
- T. Yan, M. Xu, B. Wu, Z. Liao, Z. Liu, X. Zhao, K. Bi and Y. Jia, The effect of Schisandra chinensis extracts on depression by noradrenergic, dopaminergic, GABAergic and glutamatergic systems in the forced swim test in mice, Food Funct., 2016, 7, 2811–2819 RSC.
- S. Y. Kang, K. Y. Lee, K. A. Koo, J. S. Yoon, S. W. Lim, Y.
C. Kim and S. H. Sung, ESP-102, a standardized combined extract of Angelica gigas, Saururus chinensis and Schizandra chinensis, significantly improved scopolamine-induced memory impairment in mice, Life Sci., 2005, 76, 1691–1705 CrossRef CAS PubMed.
- B. Y. Yang, J. Y. Tan, Y. Liu, B. Liu, S. Jin, H. W. Guo and H. X. Kuang, A UPLC-TOF/MS-based metabolomics study of rattan stems of Schisandra. chinensis effects on Alzheimer's disease rats model, Biomed. Chromatogr., 2018, 32, 1–12 Search PubMed.
- W. J. Sun, S. Jing, C. M. Wang, C. Y. Zhang, H. X. Sun, J. H. Li, J. H. Sun, J. G. Chen and H. Li, Effects of Schisandra Polysaccharides on the Learning and Memory Ability in Brain Aging Mice, Appl. Mech. Mater., 2014, 675–677, 1595–1599 Search PubMed.
- M. Miao, J. Gao, G. Zhang, X. Ma and Y. Zhang, Effect of Schisandra chinensis polysaccharide on intracerebral acetylcholinesterase and monoamine neurotransmitters in a D-galactose-induced aging brain mouse model, Neural Regener. Res., 2009, 4, 687–693 Search PubMed.
- R. D. Beger, J. Sun and L. K. Schnackenberg, Metabolomics approaches for discovering biomarkers of drug-induced hepatotoxicity and nephrotoxicity, Toxicol. Appl. Pharmacol., 2010, 243, 154–166 CrossRef CAS PubMed.
- F. Dieterle, A. Ross, A. Götz Schlotterbeck and H. Senn, Probabilistic Quotient Normalization as Robust Method to Account for Dilution of Complex Biological Mixtures. Application in 1H NMR Metabonomics, Anal. Chem., 2006, 78, 4281–4290 CrossRef CAS PubMed.
- Y. Zhang, Z. Pi, F. Song and Z. Liu, Ginsenosides attenuate d-galactose- and AlCl3-inducedspatial memory impairment by restoring the dysfunction of the neurotransmitter systems in the rat model of Alzheimer's disease, J. Ethnopharmacol., 2016, 194, 188–195 CrossRef CAS PubMed.
- X. E. Zhao, Y. He, M. Li, G. Chen, N. Wei, X. Wang, J. Sun, S. Zhu and J. You, Analysis of amino acid and monoamine neurotransmitters and their metabolites in rat urine of Alzheimer's disease using in situ ultrasound-assisted derivatization dispersive liquid-liquid microextraction with UHPLC-MS/MS, J. Pharm. Biomed. Anal., 2017, 135, 186–198 CrossRef CAS PubMed.
- Y. Y. Lim, P. Maruff, R. Schindler, B. R. Ott, S. Salloway, D. C. Yoo, R. B. Noto, C. Y. Santos and P. J. Snyder, Disruption of cholinergic neurotransmission exacerbates Aβ-related cognitive impairment in preclinical Alzheimer's disease, Neurobiol. Aging, 2015, 36, 2709–2715 CrossRef CAS PubMed.
- S. Reeves, M. Mehta, R. Howard, P. Grasby and R. Brown, The dopaminergic basis of cognitive and motor performance in Alzheimer's disease, Neurobiol. Dis., 2010, 37, 477–482 CrossRef CAS PubMed.
- Y. Li, S. Hao, Z. Chen, H. Xu, G. Bu and Z. Hui, Implications of GABAergic Neurotransmission in Alzheimer's Disease, Front. Aging Neurosci., 2016, 8, 1–12 CAS.
- A. P. Lin, F. Shic, C. Enriquez and B. D. Ross, Reduced glutamate neurotransmission in patients with Alzheimer's disease – an in vivo (13)C magnetic resonance spectroscopy study, Magn. Reson. Mater. Phys., Biol. Med., 2003, 16, 29–42 CrossRef CAS PubMed.
- R. D. Cardiff, C. H. Miller and R. J. Munn, Manual hematoxylin and eosin staining of mouse tissue sections, Cold Spring Harb. Protoc., 2014, 2014, 655–658 Search PubMed.
- L. Huang, A. M. Xu and Q. Peng, CD147 and MMP-9 expressions in type II/III adenocarcinoma of esophagogastric junction and their clinicopathological significances, Int. J. Clin. Exp. Pathol., 2014, 8, 1929–1937 Search PubMed.
-
M. A. Mehta, Morris Water Maze, Springer Berlin Heidelberg, 2010, vol. 46, pp. 799–799 Search PubMed.
- D. Prvulovic and H. Hampel, Amyloid β (Aβ) and phospho-tau (p-tau) as diagnostic biomarkers in Alzheimer's disease, Clin. Chem. Lab. Med., 2011, 49, 367–374 CAS.
- M. Baksalerskapazera and G. Niewiadomska, Structure and role of the tau protein, Postepy Biochem., 2002, 48, 287–295 CAS.
- C. Lv, Q. Li, X. Liu, B. He, Z. Sui, H. Xu, Y. Yin, R. Liu and K. Bi, Determination of catecholamines and their metabolites in rat urine by ultra-performance liquid chromatography-tandem mass spectrometry for the study of identifying potential markers for Alzheimer's disease, J. Mass Spectrom., 2015, 50, 354–363 CrossRef CAS PubMed.
- X. E. Zhao, Y. He, M. Li, G. Chen, N. Wei, X. Wang, J. Sun, S. Zhu and J. You, Analysis of amino acid and monoamine neurotransmitters and their metabolites in rat urine of Alzheimer's disease using in situ ultrasound-assisted derivatization dispersive liquid-liquid microextraction with UHPLC-MS/MS, J. Pharm. Biomed. Anal., 2017, 135, 186–198 CrossRef CAS PubMed.
- P. Francis, A. Palmer, M. Snape and G. Wilcock, The cholinergic hypothesis of Alzheimer's disease: a review of progress, J. Neurol., Neurosurg. Psychiatry, 1999, 66, 137–147 CrossRef CAS.
- B. Wei, Q. Li, R. Fan, D. Su, X. Ou, K. Chen, X. Chen, Y. Jia and K. Bi, UFLC-MS/MS method for simultaneous determination of six lignans of Schisandra chinensis (Turcz.) Baill. in normal and insomniac rats brain microdialysates and homogenate samples: towards an in-depth study for its sedative-hypnotic activity, J. Mass Spectrom., 2013, 48, 448–458 CrossRef CAS PubMed.
- R. A. Mitchell, N. Herrmann and K. L. L. T, The Role of Dopamine in Symptoms and Treatment of Apathy in Alzheimer's Disease, CNS Neurosci. Ther., 2010, 17, 411–427 CrossRef PubMed.
- S. Reeves, C. Bench and R. Howard, Ageing and the nigrostriatal dopaminergic system, Int. J. Geriatr. Psychopharmacol., 2010, 17, 359–370 CrossRef PubMed.
- M. T. Heneka, F. Nadrigny, T. Regen, A. Martinez-Hernandez, L. Dumitrescu-Ozimek, D. Terwel, D. Jardanhazi-Kurutz, J. Walter, F. Kirchhoff and U. K. Hanisch, Locus ceruleus controls Alzheimer's disease pathology by modulating microglial functions through norepinephrine, Proc. Natl. Acad. Sci. U. S. A., 2010, 107, 6058–6063 CrossRef CAS PubMed.
- J. H. Yang, E. O. Lee, S. E. Kim, Y. H. Suh and Y. H. Chong, Norepinephrine differentially modulates the innate inflammatory response provoked by amyloid-β peptide via action at β-adrenoceptors and activation of cAMP/PKA pathway in human THP-1 macrophages, Exp. Neurol., 2012, 236, 199–206 CrossRef CAS PubMed.
- T. Chalermpalanupap, B. Kinkead, W. T. Hu, M. P. Kummer, T. Hammerschmidt, M. T. Heneka, D. Weinshenker and A. I. Levey, Targeting norepinephrine in mild cognitive impairment and Alzheimer's disease, Alzheimers Res. Ther., 2013, 5, 21 CrossRef CAS PubMed.
- W. H. de Jong, E. G. de Vries and I. P. Kema, Current status and future developments of LC-MS/MS in clinical chemistry for quantification of biogenic amines, Clin. Biochem., 2011, 44, 95–103 CrossRef CAS PubMed.
- D. T. Marc, J. W. Ailts, D. C. Campeau, M. J. Bull and K. L. Olson, Neurotransmitters excreted in the urine as biomarkers of nervous system activity: validity and clinical applicability, Neurosci. Biobehav. Rev., 2011, 35, 635–644 CrossRef CAS PubMed.
- S. Shi, B. Zhao, G. Yagnik and F. Zhou, An interface for sensitive analysis of monoamine neurotransmitters by ion-pair chromatography-electrospray ionization-mass spectrometry with continuous online elimination of ion-pair reagents, Anal. Chem., 2013, 85, 6598–6602 CrossRef CAS PubMed.
- G. Oxenkrug, P. Requintina and S. Bachurin, Antioxidant and antiaging activity of N-acetylserotonin and melatonin in the in vivo models, Ann. N. Y. Acad. Sci., 2010, 939, 190–199 CrossRef.
- S. Bachurin, G. Oxenkrug, N. Lermontova, A. Afanasiev, B. Beznosko, G. Vankin, E. Shevtzova, T. Mukhina and T. Serkova, N-acetylserotonin, melatonin and their derivatives improve cognition and protect against beta-amyloid-induced neurotoxicity, Ann. N. Y. Acad. Sci., 2010, 890, 155–166 CrossRef.
- K. L. Lanctôt, N. Herrmann, P. Mazzotta, L. R. Khan and N. Ingber, GABAergic function in Alzheimer's disease: evidence for dysfunction and potential as a therapeutic target for the treatment of behavioural and psychological symptoms of dementia, Can. J. Psychiatry, 2004, 49, 439–453 CrossRef PubMed.
- R. Seidl, N. Cairns, N. Singewald, S. T. Kaehler and G. Lubec, Differences between GABA levels in Alzheimer's disease and Down syndrome with Alzheimer-like neuropathology, Naunyn-Schmiedeberg's Arch. Pharmacol., 2001, 363, 139–145 CrossRef CAS PubMed.
- B. Marescau, P. P. De Deyn, J. Holvoet, I. Possemiers, G. Nagels, V. Saxena and C. Mahler, Guanidino compounds in serum and urine of cirrhotic patients, Metabolism, 1995, 44, 584–588 CrossRef CAS PubMed.
- E. Matyja, Aluminum enhances glutamate-mediated neurotoxicity in organotypic cultures of rat hippocampus, Folia Neuropathol., 2000, 38, 47–53 CAS.
- G. Richterlevin, L. Canevari and T. V. P. Bliss, Long-term potentiation and glutamate release in the dentate gyrus: Links to spatial learning, Behav. Brain Res., 1995, 66, 37–40 CrossRef CAS.
- S. Ida, K. Kuriyama, Y. Tomida and H. Kimura, Antisera against taurine: quantitative characterization of the antibody specificity and its application to immunohistochemical study in the rat brain, J. Neurosci. Res., 1987, 18, 626 CrossRef CAS PubMed.
- R. Banerjee, V. Vitvitsky and S. K. Garg, The undertow of sulfur metabolism on glutamatergic neurotransmission, Trends Biochem. Sci., 2008, 33, 413–419 CrossRef CAS PubMed.
- S. S. Oja and P. Saransaari, Pharmacology of taurine, Proc. West. Pharmacol. Soc., 2007, 50, 8–15 CAS.
- I. A. El, Taurine increases mitochondrial buffering of calcium: role in neuroprotection, Amino Acids, 2008, 34, 321–328 CrossRef PubMed.
- S. W. Schaffer, J. Azuma and J. D. Madura, Mechanisms underlying taurine-mediated alterations in membrane function, Amino Acids, 1995, 8, 231–246 CrossRef CAS PubMed.
- C. J. Jong, J. Azuma and S. Schaffer, Mechanism underlying the antioxidant activity of taurine: prevention of mitochondrial oxidant production, Amino Acids, 2012, 42, 2223–2232 CrossRef CAS PubMed.
- M. Sun, Y. Zhao, Y. Gu and C. Xu, Anti-inflammatory mechanism of taurine against ischemic stroke is related to down-regulation of PARP and NF-κB, Amino Acids, 2012, 42, 1735–1747 CrossRef CAS PubMed.
- M. Sun, Y. Gu, Y. Zhao and C. Xu, Protective functions of taurine against experimental stroke through depressing mitochondria-mediated cell death in rats, Amino Acids, 2011, 40, 1419–1429 CrossRef CAS PubMed.
- P. R. Louzada, A. C. Paula Lima, D. L. Mendoncasilva, F. Noël, F. G. De Mello and S. T. Ferreira, Taurine prevents the neurotoxicity of beta-amyloid and glutamate receptor agonists: activation of GABA receptors and possible implications for Alzheimer's disease and other neurological disorders, FASEB J., 2004, 18, 511–518 CrossRef CAS PubMed.
- M. G. Tadros, A. E. Khalifa, A. B. Abdelnaim and H. M. Arafa, Neuroprotective effect of taurine in 3-nitropropionic acid-induced experimental animal model of Huntington's disease phenotype, Pharmacol., Biochem. Behav., 2005, 82, 574–582 CrossRef CAS PubMed.
- S. T. Ferreira, M. V. Lourenco, M. M. Oliveira and F. G. De Felice, Soluble amyloid-β oligomers as synaptotoxins leading to cognitive impairment in Alzheimer's disease, Front. Cell. Neurosci., 2015, 91, 91 Search PubMed.
- H. Y. Kim, S. H. Yang, J. Yoon, H. Jang, S. Baek, J. Shin, S. H. Kim and Y. Kim, Taurine-Carbohydrate Derivative Stimulates Fibrillogenesis of Amyloid-β and Reduce Alzheimer-Like Behaviors, Adv. Exp. Med. Biol., 2017, 975, 225–232 CrossRef CAS PubMed.
- H. Y. Kim, H. V. Kim, J. H. Yoon, B. R. Kang, S. M. Cho, S. Lee, J. Y. Kim, J. W. Kim, Y. Cho and J. Woo, Taurine in drinking water recovers learning and memory in the adult APP/PS1 mouse model of Alzheimer's disease, Alzheimers Dement., 2015, 11, P839–P840 CrossRef.
-
H. C. Jang, S. Lee, S. L. Choi, H. Y. Kim, S. Baek and Y. S. Kim, Taurine Directly Binds to Oligomeric Amyloid-β and Recovers Cognitive Deficits in Alzheimer Model Mice, Oxygen Transport to Tissue XXXIII, 2017, vol. 975, pp. 233–241 Search PubMed.
- G. Novarino, C. Fabrizi, R. Tonini, M. A. Denti, F. Malchiodi-Albedi, G. M. Lauro, B. Sacchetti, S. Paradisi, A. Ferroni, P. M. Curmi, S. N. Breit and M. Mazzanti, Involvement of the Intracellular Ion Channel CLIC1 in Microglia-Mediated -Amyloid-Induced Neurotoxicity, J. Neurosci., 2004, 24, 5322–5330 CrossRef CAS PubMed.
- H. Zhang, S. Wu and X. Da, YAP accelerates Aβ 25–35 -induced apoptosis through upregulation of Bax expression by interaction with p73, Apoptosis, 2011, 16, 808–821 CrossRef CAS PubMed.
- R. Anisur, The Role of Adenosine in Alzheimer's Disease, Curr. Neuropharmacol., 2009, 7, 207–216 CrossRef PubMed.
- E. P. Kennedy and S. B. Weiss, The function of cytidine coenzymes in the biosynthesis of phospholipides, J. Biol. Chem., 1956, 222, 193–214 CAS.
- B. Goren, A. Cakir, B. Ocalan, K. S. Serter, T. Alkan, M. Cansev and N. Kahveci, Long-term cognitive effects of uridine treatment in a neonatal rat model of hypoxic-ischemic encephalopathy., Brain Res., 2017, 1659, 81–87 CrossRef CAS PubMed.
- G. F. Oxenkrug, Genetic and hormonal regulation of tryptophan kynurenine metabolism: implications for vascular cognitive impairment, major depressive disorder, and aging, Ann. N. Y. Acad. Sci., 2010, 1122, 35–49 CrossRef PubMed.
- H. Baran, K. Jellinger and L. Deecke, Kynurenine metabolism in Alzheimer's disease, J. Neural Transm., 1999, 106, 165–181 CrossRef CAS PubMed.
- C. Klein, C. Pattemensah, O. Taleb, J. J. Bourguignon, M. Schmitt, F. Bihel, M. Maitre and A. G. Mensahnyagan, The neuroprotector kynurenic acid increases neuronal cell survival through neprilysin induction, Neuropharmacology, 2013, 70, 254–260 CrossRef CAS PubMed.
- R. Schwarcz, W. Whetsell and R. Mangano, Quinolinic acid: an endogenous metabolite that produces axon-sparing lesions in rat brain, Science, 1983, 219, 316–318 CrossRef CAS PubMed.
- J. R. Ocampo, R. L. Huitrón, D. Gonzálezesquivel, P. Ugaldemuñiz, A. Jiménezanguiano, B. Pineda, J. Pedrazachaverri and C. Ríos, Kynurenines with Neuroactive and Redox Properties: Relevance to Aging and Brain Diseases, Oxid. Med. Cell. Longevity, 2014, 2014, 646909 Search PubMed.
- A. M. Myint and Y. K. Kim, Cytokine-serotonin interaction through IDO: a neurodegeneration hypothesis of depression, Med. Hypotheses, 2003, 61, 519–525 CrossRef CAS.
- R. Poesen, P. Evenepoel, L. H. De, D. Kuypers, P. Augustijns and B. Meijers, Metabolism, Protein Binding, and Renal Clearance of Microbiota-Derived p-Cresol in Patients with CKD, Clin. J. Am. Soc. Nephrol., 2016, 11, 1136 CrossRef CAS PubMed.
- S. Liabeuf, D. V. Barreto, F. C. Barreto, N. Meert, G. Glorieux, E. Schepers, M. Temmar, G. Choukroun, R. Vanholder and Z. A. Massy, Free p-cresylsulphate is a predictor of mortality in patients at different stages of chronic kidney disease, Nephrol., Dial., Transplant., 2010, 25, 1183–1191 CrossRef CAS PubMed.
- I. W. Wu, K. H. Hsu, H. J. Hsu, C. C. Lee, C. Y. Sun, C. J. Tsai and M. S. Wu, Serum free p-cresyl sulfate levels predict cardiovascular and all-cause mortality in elderly hemodialysis patients–a prospective cohort study, Nephrol., Dial., Transplant., 2012, 27, 1169–1175 CrossRef CAS PubMed.
- L. K. Ferreira, J. H. Tamashiroduran, P. Squarzoni, F. L. Duran, T. C. Alves, C. A. Buchpiguel and G. F. Busatto, The link between cardiovascular risk, Alzheimer's disease, and mild cognitive impairment: support from recent functional neuroimaging studies, Rev. Bras. Psiquiatr., 2014, 36, 344–357 CrossRef PubMed.
- B. Imtiaz, A. M. Tolppanen, M. Kivipelto and H. Soininen, Future directions in Alzheimer's disease from risk factors to prevention, Biochem. Pharmacol., 2014, 88, 661–670 CrossRef CAS PubMed.
- R. F. D. Bruijn and M. A. Ikram, Cardiovascular risk factors and future Alzheimer's disease risk, BMC Med., 2014, 12, 130 CrossRef PubMed.
- D. E. Barnes and K. Yaffe, The projected effect of risk factor reduction on Alzheimer's disease prevalence, Lancet Neurol., 2011, 7, S511 Search PubMed.
- F. Moroni, Tryptophan metabolism and brain function: focus on kynurenine and other indole metabolites, Eur. J. Pharmacol., 1999, 375, 87 CrossRef CAS PubMed.
- Y. E. Geda, R. O. Roberts, D. S. Knopman, T. J. H. Christianson, V. S. Pankratz, R. J. Ivnik, B. F. Boeve, E. G. Tangalos, R. C. Petersen and W. A. Rocca, Physical Exercise, Aging, and Mild Cognitive Impairment: A Population-Based Study, Mayo Clin. Proc., 2012, 87, 437–442 CrossRef PubMed.
- L. D. Baker, L. L. Frank, K. Fosterschubert, P. S. Green, C. W. Wilkinson, A. Mctiernan, S. R. Plymate, M. A. Fishel, G. S. Watson and B. A. Cholerton, Effects of Aerobic Exercise on Mild Cognitive Impairment: A Controlled Trial, Arch. Neurol., 2010, 67, 71–79 Search PubMed.
- K. Nichol, S. P. Deeny, J. Seif, K. Camaclang and C. W. Cotman, Exercise improves cognition and hippocampal plasticity in APOE ε4 mice, Alzheimers Dement., 2009, 5, 287–294 CrossRef CAS PubMed.
- S. Rossi, E. R. Zanier, I. Mauri, A. Columbo and N. Stocchetti, Brain temperature, body core temperature, and intracranial pressure in acute cerebral damage, J. Neurol., Neurosurg. Psychiatry, 2001, 71, 448 CrossRef CAS.
- L. Mosconi, W. H. Tsui, K. Herholz, A. Pupi, A. Drzezga, G. Lucignani, E. M. Reiman, V. Holthoff, E. Kalbe and S. Sorbi, Multicenter Standardized 18F-FDG PET Diagnosis of Mild Cognitive Impairment, Alzheimer's Disease, and Other Dementias, J. Nucl. Med., 2008, 49, 390–398 CrossRef PubMed.
- B. Yu, Z. Huang, X. Y. Wang and G. Z. Zhao, Study on THz Spectra of Nicotinic Acid, Nicotinamide and Nicotine, Spectrosc. Spect. Anal., 2009, 29, 2334–2337 CAS.
- S. Chong, Z. Sun, D. Chen, X. Su, J. Jing, G. Li, B. Lin and J. Yan, Developing Urinary Metabolomic Signatures as Early Bladder Cancer Diagnostic Markers, Omics, 2015, 19, 1–11 CrossRef PubMed.
- R. D. Holland, Metabonomics evaluation of urine from rats given acute and chronic doses of acetaminophen using NMR and UPLC/MS, J. Chromatogr. B: Anal. Technol. Biomed. Life Sci., 2008, 871, 328–340 CrossRef PubMed.
- R. Castellani, A. Nunomura, R. Rolston, P. Moreira, A. Takeda, G. Perry and M. Smith, Sublethal RNA Oxidation as a Mechanism for Neurodegenerative Disease, Int. J. Mol. Sci., 2008, 9, 789–806 CrossRef CAS PubMed.
- D. F. Di, G. Pupo, A. Tramutola, A. Giorgi, M. E. Schininà, R. Coccia, E. Head, D. A. Butterfield and M. Perluigi, Redox proteomics analysis of HNE-modified proteins in Down syndrome brain: clues for understanding the development of Alzheimer disease, Free Radicals Bio. Med., 2014, 71, 270–280 CrossRef PubMed.
- A. Gómez-Ramos, J. Díaz-Nido, M. A. Smith, G. Perry and J. Avila, Effect of the lipid peroxidation product acrolein on tau phosphorylation in neural cells, J. Neurosci.
Res., 2003, 71, 863–870 CrossRef PubMed.
- J. Wang, W. R. Markesbery and M. A. Lovell, Increased oxidative damage in nuclear and mitochondrial DNA in mild cognitive impairment, J. Neurochem., 2010, 96, 825–832 CrossRef PubMed.
- S. Varadarajan, S. Yatin, M. Aksenova and D. A. Butterfield, Review: Alzheimer's Amyloid β-Peptide-Associated Free Radical Oxidative Stress and Neurotoxicity, J. Struct Biol., 2000, 130, 184–208 CrossRef CAS PubMed.
- G. Wu, F. W. Bazer, T. A. Davis, S. W. Kim, P. Li, R. J. Marc, S. M. Carey, S. B. Smith, T. E. Spencer and Y. Yin, Arginine metabolism and nutrition in growth, health and disease, Amino Acids, 2009, 37, 153–168 CrossRef CAS PubMed.
- M. Basso and M. Pennuto, Serine phosphorylation and arginine methylation at the crossroads to neurodegeneration, Exp. Neurol., 2015, 271, 77–83 CrossRef CAS PubMed.
- G. Paglia, M. Stocchero, S. Cacciatore, S. Lai, P. M. Angel, M. T. Alam, M. Keller, M. Ralser and G. Astarita, An unbiased metabolomic investigation of the Alzheimer's disease brain points to a dysregulation of the mitochondrial aspartate metabolism, J. Proteome Res., 2015, 15, 1334–1341 Search PubMed.
- C. Ibáñez, C. Simó, P. J. Martín-Álvarez, M. Kivipelto, B. Winblad, A. Cedazo-Mínguez and A. Cifuentes, Toward a predictive model of Alzheimer's disease progression using capillary electrophoresis-mass spectrometry metabolomics, Anal. Chem., 2012, 84, 8532–8540 CrossRef PubMed.
- S. Beddhu, M. H. Samore, M. S. Roberts, G. J. Stoddard, L. M. Pappas and A. K. Cheung, Creatinine production, nutrition, and glomerular filtration rate estimation, J. Am. Soc. Nephrol., 2003, 14, 1000–1005 CrossRef PubMed.
- S. B. Heymsfield, C. Arteaga, C. Mcmanus, J. Smith and S. Moffitt, Measurement of muscle mass in humans: validity of the 24-hour urinary creatinine method, Am. J. Clin. Nutr., 1983, 37, 478–494 CrossRef CAS PubMed.
- E. E. Jansen, N. M. Verhoeven, C. Jakobs, A. Schulze, H. Senephansiri, M. Gupta, O. C. Snead and K. M. Gibson, Increased guanidino species in murine and human succinate semialdehyde dehydrogenase (SSADH) deficiency, Biochim. Biophys. Acta, Mol. Basis Dis., 2006, 1762, 494–498 CrossRef CAS PubMed.
- O. Felicity, S. Louis, E. Gazit and M. A. De, Is Nitric Oxide Assuming a Janus-Face in The Central Nervous System?, Curr. Med. Chem., 2016, 23, 1625–1637 CrossRef.
- S. Chakroborty, J. Kim, C. Schneider, A. R. West and G. E. Stutzmann, Nitric Oxide Signaling Is Recruited As a Compensatory Mechanism for Sustaining Synaptic Plasticity in Alzheimer's Disease Mice, J. Neurosci., 2015, 35, 6893–6902 CrossRef CAS PubMed.
- N. Nagai, Y. Ito, T. Shibata, E. Kubo and H. Sasaki, A positive feedback loop between nitric oxide and amyloid β (1–42) accelerates mitochondrial damage in human lens epithelial cells, Toxicology, 2017, 381, 19–30 CrossRef CAS PubMed.
- P. Guo, What should children do with lysine metabolism disorder?, J. Chronic Dis., 2000, 5, 33 Search PubMed.
- M. Bohgaki, T. Tsukiyama, A. Nakajima, S. Maruyama, M. Watanabe, T. Koike and S. Hatakeyama, Involvement of Ymer in suppression of NF-kappaB activation by regulated interaction with lysine-63-linked polyubiquitin chain, Biochim. Biophys. Acta, Mol. Cell Res., 2008, 1783, 826–837 CrossRef CAS PubMed.
Footnote |
† Electronic supplementary information (ESI) available. See DOI: 10.1039/C8FO02067A |
|
This journal is © The Royal Society of Chemistry 2019 |