DOI:
10.1039/C8FO01609G
(Paper)
Food Funct., 2019,
10, 448-457
Polysaccharides from fermented Momordica charantia ameliorate obesity in high-fat induced obese rats†
Received
12th August 2018
, Accepted 24th October 2018
First published on 10th January 2019
Abstract
Momordica charantia (M. charantia) has been widely used to treat obesity due to its bioactive ingredients. This research aimed to investigate the anti-obesity effect of polysaccharides (FP) from fermented M. charantia with Lactobacillus plantarum NCU116 on high-fat induced obese rats. We found that FP could effectively lower the body weight gain, Lee's index, insulin resistance and cell sizes of epididymal adipose tissues in obese rats compared with polysaccharides from non-fermented M. charantia (NFP). FP treatments decreased the total cholesterol, triacylglycerols, and low-density lipoprotein cholesterol, leptin, whereas they elevated the high-density lipoprotein cholesterol, adiponectin, significantly in the serum of obese rats. Furthermore, administrations of FP notably improved oxidative balance in obese rats. Lipidomics results indicated that 24 potential biomarkers have been identified in serum. Additionally, 21 lipids were considerably altered by FP and NFP intakes, such as fatty acyls, glycerolipids, sphingolipids, sterol lipids and glycerophospholipids. The anti-obesity properties of FP were revealed via relieving insulin resistance and fat accumulation of obese rats, which was associated with the regulation of lipid metabolism. Overall, FP exerted more favourable impacts on the anti-obesity effect than NFP, which may be attributed to fermentation.
1. Introduction
Obesity is a disease of the endocrine system, which involves many tissues and metabolic processes.1 Consequently, it is essential to treat obesity to maintain good health. For this purpose, various medicines have been applied to treat obesity; however, these drugs generally cause side effects.2 Diets such as fruit and vegetables are considered as another main option for the management of the severity of overweight and related chronic diseases.3 The increase in high calorie-low fiber fast food consumption caused the prevalence of obesity in the urban population of developing countries when compared with Western counterparts.4 To this end, plant-based dietary fibers have been used to alleviate obesity.5
Momordica charantia L. (M. charantia) exhibits a broad range of bioactivities, such as anti-cancer,6 anti-diabetic,7 and anti-inflammatory.8M. charantia has prompted plenty of research activities for the anti-obesity effect.9 As a major component of M. charantia, polysaccharides also have anti-diabetic10 and hypoglycemic effects.11 Although these properties are related to ameliorating obesity, the anti-obesity effect of M. charantia polysaccharides has not yet been confirmed.
Considering the structure–function relationship, food processing methods could affect the functionalities of polysaccharides via altering their structures. For instance, microwave and ultrasonic irradiation triggered the degradation of polysaccharides from the seeds of Plantago asiatica L. and hence improved their bioactivities.12 By virtue of its health-related benefits, fermentation is considered as a beneficial methodology to manufacture functional food via the biotransformation of food components such as polysaccharides, and not just a traditional food preservation method.9 For example, by altering the structure of the polysaccharides derived from Ishige okamurae and ginseng, fermentation enhanced their functionalities including antioxidant, radioprotective, and immunomodulating activities,13 but data on the improvement of the anti-obesity effect have not yet been reported. As they possess many functions, probiotics are widely applied to prepare fermented fruit and vegetable juices.14 As a probiotic, Lactobacillus plantarum (L. plantarum) NCU116 achieved a large-scale production and has been successfully applied in fruit and vegetable fermentation.15 Although fermentation using this strain could enhance the functional properties of the fermented product, observations on the transformation of compounds are limited. A previous study has shown that this strain could release various carbohydrate-active enzymes (CAZy), which could catalyze the hydrolysis of plant-based polysaccharides in the food matrix during fermentation, leading to the enhancement of the bioactivity of polysaccharides.16 Physicochemical characterization of polysaccharides from fermented (FP) and non-fermented (NFP) M. charantia using L. plantarum NCU116 has been shown in a previously published paper, and the total sugar content in FP (57.48 ± 0.02%) was higher than that in NFP (53.13 ± 0.00%); the apparent viscosity in FP was less than that in NFP at the same concentration. The reduction in viscosity may be attributed to the change of the monosaccharide composition, which might enhance the bioactivities of polysaccharides. And our recent study presented the positive effects of FP on the blood glucose level in terms of hyperglycemia, hyperinsulinemia and hyperlipidemia in diabetic rats.10 Therefore, this manuscript aimed to investigate the anti-obesity effect of polysaccharides from Lactobacillus plantarum-fermented M. charantia on high-fat induced obese rats.
2. Materials and methods
2.1 Preparation of M. charantia juices
Fresh M. charantia was purchased from a local supermarket (Nanchang, China). And M. charantia was authenticated by Ce-ming Tan in Jiangxi province, China, in March 2016. A voucher specimen (JJF00015574) has been deposited at the Herbarium Jiujiang Forestry Institute (JJF). The pulp of M. Charantia (after detachment of seed, internal tissue and peel) was crushed and sterilized at 102 °C for 20 min with 1 Atm to obtain non-fermented juice (NFJ). Subsequently, NFJ was inoculated with 0.01% Lactobacillus plantarum NCU116 strain powder and fermented at 37 °C for 48 h to prepare fermented juice (FJ). After preparation, NFJ and FJ were stored at 4 °C until use.
2.2 Preparation of M. charantia polysaccharides
Polysaccharides were extracted from NFJ and FJ. Briefly, the juice with added 80% of distilled water (v/v) was extracted at 100 °C for 3 h and filtered. This process was repeated 3 times and the residues were collected and mixed. The mixture was concentrated by using a rotary evaporator to 20% of the original volume at 60 °C under vacuum. The concentrated liquid was precipitated with 80% of anhydrous ethanol (v/v) at 4 °C for 24 h. After that, distilled water was added to dissolve the precipitate. The protein was removed by the Sevag method.17 The resulting extract was dialyzed and precipitated with anhydrous ethanol at a final concentration of 80% (v/v) at 4 °C for 24 h and lyophilized to obtain the polysaccharides (NFP, from NFJ; FP, from FJ).
2.3 Experimental design
The study complied with the National Guidelines for Experimental Animal Welfare (MOST of PR China, 2006), and the protocol was approved by the Institutional Animal Care and Use Committee of Nanchang University (SYXK (Gan) 2015-0001). One hundred male Sprague-Dawley18 rats (160–200 g) were obtained from Hunan Silaike Jingda Laboratory Animal Co Ltd (China). Rats were housed at the constant temperature of 25 ± 2 °C, a relative humidity of 50 ± 5% and 12/12 h of day-night cycle. After one week adjustment, the rats were divided into eight groups as follows: (1) normal, fed a normal diet, (2) model, fed a high fat diet, 66.5% standard pellet diet, 20% sucrose, 10% lard, 2.5% cholesterol, and 1% sodium cholate,19 (3) N + FPM, fed a normal diet with FP at middle dose (100 mg per kg of body weight, b.w.), (4) N + NFP, fed a normal diet with NFP at 100 mg kg−1, (5) FPL, fed a high fat diet with low dose FP at 50 mg kg−1, (6) FPM, fed a high fat diet with middle dose FP, (7) FPH, fed a high fat diet with high dose FP at 200 mg kg−1 and (8) NFP, fed a high fat diet with NFP. Oral administrations were conducted with 10 mL per kg of b.w. over the 8-week experimental period. Body weight was recorded daily, and the body length was finally measured to calculate Lee's index. At the end of this experiment, rats were executed after fasting for 12 h, and the blood, epididymal fats and livers were quickly removed. Blood samples were centrifuged at 1000gfor 10 min and the serum was collected for the following analysis. All samples were stored at −80 °C before use. Lee's index was calculated according to the formula:
Lee's index = body weight (g) 1/3 × 1000/body length (cm). |
2.4 Oral glucose tolerance test (OGTT)
The rats used for the oral glucose tolerance test fasted overnight. Rats were given glucose by oral gavage (2 g kg−1). Blood glucose levels were determined by using Accu-Chek Performa (Roche Diagnostics, Mannheim, Germany) from the tail vein blood, and then the blood glucose level was evaluated at 0, 30, 60, 90, 120, and 180 min, respectively.
2.5 Serum lipids
Levels of serum lipids including total cholesterol (TC), triacylglycerols (TG), high-density lipoprotein cholesterol (HDL-C), and low-density lipoprotein cholesterol (LDL-C) were determined by using an Automatic Biochemical Analyzer (Mindray BS-380, Shengzheng, China).
2.6 Serum hormones
Serum insulin, leptin, and adiponectin were estimated using the assay kits purchased from Nanjing Jiancheng Bioengineering Institute, Jiangsu, China. The homeostasis model assessment of insulin resistance (HOMA-IR) and the quantitative insulin sensitivity check index (QUICKI) were used to investigate the insulin resistance of rats in this experiment, and calculated according to the formula:
HOMA-IR = (fast insulin × fasting glucose)/22.5 |
QUICKI = 1/[(fast insulin) + log(fasting glucose)]. |
2.7 Histopathological examination
The epididymal fat and liver of rats were collected, immersed in 10% buffered formalin, dehydrated by using graded alcohol, and embedded in paraffin wax. Samples were cut into slices of about 5 μm and mounted on slides. Subsequently, the sample was stained with hematoxylin and eosin after deparaffinization. The stained slide was observed by using a Nikon Ti series inverted microscope equipped with bright-field high-quality objectives. Images were obtained by using a digital camera (Nikon Digital Sight DS-Filc) and image acquisition software.
2.8 Oxidative stress
Levels of malondialdehyde (MDA) and activities of superoxide dismutase (SOD) were determined following the instructions of the kits. MDA and SOD kits were obtained from Beyotime (Shanghai, China).
2.9 Lipidomics
2.9.1 Preparation of samples.
Serum samples (25.0 μL) with added distilled water (975.0 μL) were kept on ice for 10 min. After that, 2.0 mL MeOH and 0.9 mL CH2Cl2 were added and vortexed. If two clear phases existed in these mixtures, 50.0 μL MeOH was added and vortexed, again. If not, the above-mentioned step was repeated by adding 50.0 μL MeOH and vortexing. Solutions with a single phase were allowed to sit on ice for 30 min. Next, 1.0 mL distilled water and 0.9 mL CH2Cl2 were added and vortexed. Mixtures were centrifuged at 1200 rpm for 10 min, and then the lower layers were collected and transferred into a new tube. 2.0 mL CH2Cl2 was added to the remains, mixed, centrifuged, and collected, and combined with the lower layers. Combinations were evaporated by using nitrogen and the residues were dissolved in a mixture of MeOH and CH2Cl2 at a ratio of 1
:
1 (v/v), including 5 mM ammonium.
2.9.2 UPLC-Q-TOF-MS analysis.
In this study, liquid chromatographic separation was carried out on a Shimadzu Nexera X2 UHPLC system (Kyoto, Japan). Chromatographic separation was performed with a Shim-pack GIST C18 column (2.1 mm × 75 mm, 2 μm) at 30 °C. The flow rate was set at 0.35 mL min−1 and the injection volume was set at 3.5 μL. Mobile phases consisted of a mixture including distilled water, MeOH, ACN (3
:
1
:
1) and 5 mM ammonium (A) and a mixture of isopropanol modified with 5 mM ammonium for both positive and negative modes. Conditions of the gradient program were determined as follows: 0.5 min, 80% of A; 1.5 min, 60% of A; 3 min, 40% of A; 13 min, 2% of A; 13.1 min, 80% of A; 17 min, 80% of A.
The mass system was operated with the AB SCIEX Triple TOF 5600 in both positive and negative modes. In positive mode, the collision energy (CE) was set at 35 V and the collision energy spread (CES) was 15 V. The declustering potential was set at +80 V and the ion spray voltage was set to +5500 V. In negative mode, CE was −35 V and CSE was 15 V. The declustering potential was set at −80 V and the ion spray voltage was set to −5500 V. The mass range was from 50 to 1000 m/z. The other parameters were set as follows: the curtain gas, 35 psi; the ion source gas 1 and gas 2, 50 psi; drying temperature, 500 °C.
2.10 Statistical analysis
The data were analyzed by using SPSS 17.0 software (SPSS, Inc., Chicago, IL) with one-way analysis of variance (ANOVA). A significant difference between groups was examined with Tukey's tests for means with 95% confidence limit (p < 0.05).
The LC-MS/MS data were processed using PeakView and MarkView (AB SCIEX) for the examination of the extracted ion chromatograms (XIC). The raw LC-MS/MS data regarding the retention time, peak intensity, and exact mass were imported to LipidView software (AB SCIEX) for multiple statistical analyses. Identification of the lipids was supported on MS and MS/MS information by searching in the METLIN (http://metlin.scripps.edu), Lipidmaps (http://www.lipidmaps.org), and HMDB (http://www.hmdb.ca). An accuracy error of 5 ppm was set both in MS and MS/MS data to confirm the tentative identification of metabolites. Multivariate analysis (MA) was performed on the output table from LipidView using MetaboAnalyst 4.0 (http://www.metaboanalyst.ca) and pareto scaling was used for data normalization before MA. Partial least-squares-discrimination analysis (PLS-DA, a supervised classification) was used to study the contribution of variables in group separation. And the first two principal components were applied to construct the scores plot and loading plot. Principal component analysis (PCA, an unsupervised technique) was performed to identify similarities or differences between different groups. Potential metabolite markers changing among experimental groups were selected according to the variable importance in the projection (VIP > 1) from the PLS-DA model and Student's t-test (p value < 0.05).
3. Results and discussion
3.1 Body weight and Lee's index
Obesity is associated with body weight gain.20 The initial body weight of rats in different groups was approximately 125 g (Fig. 1A). The model group reached the highest level of body weight after the 8-week experiment. Compared with non-treated rats, treatment with FP and NFP significantly decreased 8% and 7% of the body weight of obese rats, respectively (p < 0.05). The level of body weight in the N + FPM and N + NFP groups was less than that in the normal group. For obese individuals, 5% to 10% decrease of body weight was generally a recommended therapeutic target.21 Collectively, these results demonstrated that fermentation could promote the weight loss effect of M. charantia polysaccharides. To investigate whether the reduction in body weight gain correlates with Lee's index, we measured the body length in rats. Similar to the changes in body weight, all treated groups reduced remarkably compared to the model group after the 8-week experiment (p < 0.01) (Fig. 1B). Meanwhile, all FP-treated groups were lower than the NFP group. The FPM group presented the lowest Lee's index among three FP-treated obese groups, suggesting that FP might exert a positive impact on regulating the Lee's index in obese rats. Moreover, N + FPM and N + NFP also showed lower levels than that in the normal group.
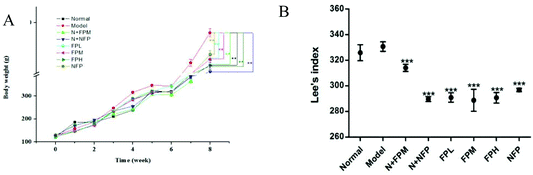 |
| Fig. 1 Body weight and Lee's index of different groups. (A) Body weight and (B) Lee's index. Normal, normal diet feed; model, high fat diet feed; N + FPM, normal diet feed plus polysaccharides from fermented M. charantia juice at middle dose with 100 mg per kg b.w.; N + NFP, normal diet feed plus polysaccharide from non-fermented M. charantia juice 100 mg per kg b.w.; FPL, high fat diet feed plus polysaccharides from fermented M. charantia juice at low dose with 50 mg per kg b.w.; FPM, high fat diet feed plus fermented M. charantia juice at middle dose with 100 mg per kg b.w.; FPH, high fat diet feed plus fermented M. charantia juice at high dose with 200 mg per kg b.w.; NFP, high fat diet feed plus polysaccharides from fermented M. charantia juice at middle dose with 100 mg per kg b.w. All values are expressed as means ± standard deviation. * indicates the significant difference compared with model groups, *p < 0.05, **p < 0.01, ***p < 0.001. | |
3.2 Serum glucose
The oral glucose tolerance test was used for investigating the antihyperglycemic effect of M. charantia polysaccharides. The area under the curve (AUC) for the glucose response during the oral glucose tolerance test was calculated by the trapezoidal rule.22 The glucose level of the model group presented a notably increasing trend. Treatment with the middle-dose FP slightly lowered the glucose level and the AUC of obese rats at 180 minutes (Fig. 2B). No significant difference was observed in the N + FPM and N + NFP groups in blood glucose concentrations compared with the normal group. The antihyperglycemic effect of FP and NFP was not efficacious in obese rats, so it is fully necessary to investigate the mechanism of the anti-obesity effects of M. charantia polysaccharides.
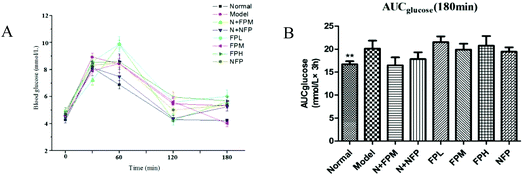 |
| Fig. 2 OGTT (A) and AUC (B) of different groups. * indicates the significant difference compared with model groups, *p < 0.05, **p < 0.01, ***p < 0.001. | |
3.3 Serum lipids
Obese individuals are associated with higher risk of dyslipidaemia and cardiovascular disease.23 Abnormal lipid metabolism is generally reflected in the increase of TC and the decrease of HDL-c in serum.24 Levels of TC, TG, and LDL-c were increased 0.4, 3.6 and 0.8-fold and the HDL-c level was decreased by 6% in the model group compared with the normal group, respectively (Table 1). Administration of FP remarkably reduced the TC and TG levels (except for low-dose FP) (p < 0.01), whereas the LDL-c level exhibited no obvious improvement in obese rats. FP and NFP treated the elevated level of HDL-c in obese rats. Moreover, the N + FPM and N + NFP group had no significant difference compared with the normal group. The previous investigation revealed that M. charantia could ameliorate dyslipidemia in obesity,25 and polysaccharides also could improve the serum lipid in HFD-induced rats.26 The present results are possibly due to the fact that M. charantia contains polysaccharides. Moreover, carrot juice fermented with L. plantarum NCU116 ameliorates serum lipids in HFD-induced rats.27 Thus, the beneficial effect of FP on the serum lipid profile in obese rats may be attributed to its fermentation effect.
Table 1 Levels of serum lipids in different groups
Groups |
TC (mmol L−1) |
TG (mmol L−1) |
LDL-C (mmol L−1) |
HDL-C (mmol L−1) |
* indicates the significant difference compared with model groups, *p < 0.05, **p < 0.01, ***p < 0.001. |
Normal |
1.64 ± 0.20*** |
1.20 ± 0.23*** |
0.26 ± 0.03*** |
1.26 ± 0.04 |
Model |
2.33 ± 0.36 |
5.54 ± 1.28 |
0.47 ± 0.11 |
1.18 ± 0.06 |
N + FPM |
1.77 ± 0.25*** |
1.29 ± 0.26*** |
0.30 ± 0.03*** |
1.38 ± 0.06 |
N + NFP |
1.70 ± 0.26*** |
1.42 ± 0.31*** |
0.32 ± 0.05*** |
1.37 ± 0.06 |
FPL |
1.96 ± 0.14 |
2.98 ± 0.81*** |
0.43 ± 0.06 |
1.33 ± 0.05 |
FPM |
1.99 ± 0.39 |
4.39 ± 1.21 |
0.43 ± 0.09 |
1.32 ± 0.06 |
FPH |
2.14 ± 0.32 |
3.80 ± 1.37*** |
0.49 ± 0.09 |
1.37 ± 0.07 |
NFP |
2.31 ± 0.26 |
4.50 ± 0.52 |
0.48 ± 0.64 |
1.48 ± 0.04** |
3.4 Serum hormones
Insulin is a peptide released by pancreatic cells. This substance has multiple functions both in the periphery and central nervous system.28 The result of the insulin level in the model and normal groups was different from the previous report.29 Intake of FP notably reduced the serum insulin level in obese rats. The concentrations of insulin were negatively associated with the dosage of FP. NFP displayed similar effects to those observed in obese rats but the values were not statistically prominent compared with the FP group. The HOMA-IR model was used for evaluating insulin resistance.30 The model group exhibited the highest value of HOMA-IR, which was in line with the previous literature.31 Administration of FP significantly decreased HOMA-IR with dose dependent effects. Meanwhile, HOMA-IR values of the FP-treated groups were lower than those of the NFP group. Based on these results, fermentation could enhance the regulatory effect on insulin resistance and sensitivity (Table 2).
Table 2 Effect on insulin levels, leptin, adiponectin levels and insulin sensitivity in different groups
Groups |
Insulin (mIU mL−1) |
HOMA-IR |
QUICK |
Leptin (ng mL−1) |
Adiponectin (mg L−1) |
* indicates the significant difference compared with model groups, *p < 0.05, **p < 0.01, ***p < 0.001. |
Normal |
40.81 ± 3.13 |
7.74 ± 0.24 |
0.45 ± 0.003 |
6.5 ± 1.02 |
3.78 ± 1.19* |
Model |
38.56 ± 6.37 |
8.08 ± 0.44 |
0.44 ± 0.007 |
7.08 ± 1.34 |
1.33 ± 0.51 |
N + FPM |
29.62 ± 6.14* |
6.01 ± 0.43** |
0.47 ± 0.009* |
5.77 ± 1.42 |
2.13 ± 0.64 |
N + NFP |
24.4 ± 2.66*** |
4.84 ± 0.22*** |
0.49 ± 0.005*** |
13.86 ± 4.18 |
2.69 ± 2.22 |
FPL |
29.83 ± 4.77* |
6.28 ± 0.41* |
0.47 ± 0.006 |
6.26 ± 1.77 |
5.17 ± 1.07*** |
FPM |
28.91 ± 4.00** |
5.91 ± 0.33** |
0.47 ± 0.005* |
4.95 ± 0.69 |
3.71 ± 0.61* |
FPH |
22.58 ± 2.31*** |
4.95 ± 0.21*** |
0.49 ± 0.004*** |
5.69 ± 1.04 |
4.17 ± 1.68** |
NFP |
31.61 ± 3.35 |
6.63 ± 0.29 |
0.46 ± 0.004 |
4.86 ± 1.21 |
5.06 ± 1.61*** |
Both leptin and adiponectin were reliable markers of adiposity in humans.32 Leptin regulates weight control and its level is mainly related to the extent of fat in the cells; it played a critical role in the regulation of energy homeostasis, insulin sensitivity, and lipid and carbohydrate metabolism.33 Concentrations of leptin in all obese rats were in accordance with the trend that plasma leptin concentrations are proportional to the degree of adiposity.34 However, adiponectin levels have an inverse relationship with the amount of adipose tissue.35 The FPL group was the highest among all-treated groups, which indicated that the low-dose FP exerted the most effective effect in regulating adiponectin. Supplementation of L. plantarum NCU116 increased the level of adiponectin.19 The trend was in line with the research which has demonstrated that obese individuals presented an increase in leptin and a decrease in adiponectin which may lead to the development of insulin resistance.36 Taken together, these results suggested that polysaccharides from Lactobacillus plantarum fermented M. charantia possessed the anti-obesity effect.
3.5 Histopathological examination
The recent study found that M. charantia treatment could decrease the cell size of epididymal adipose tissue in obese rats,25 this paper aims to explore whether M. charantia polysaccharides exerted similar effects. High-fat diet obese rats had considerably increased body weight, subcutaneous and epididymal fat accumulation, adipocyte number and size.37 To investigate whether the decrease in body weight gain is related to a loss of fat accumulation, we measured the sizes of epididymal adipose. Consistent with the trend in body weight, the size of epididymal adipose tissue in the model group was larger than that in the normal group (Fig. 3). For all treated groups, their sizes of epididymal adipose were smaller than the model group. FPL and FPM groups showed smaller adipocyte sizes than NFP groups, suggesting that L. plantarum fermentation might stimulate the regulation of M. charantia polysaccharides on adipose tissue in obese rats. The results indicated that M. charantia polysaccharides also could inhibit the development of obesity via alleviating fat accumulation, and the effect of M. charantia polysaccharides on the inhibition of body weight gain might be associated with a reduction in fat mass.
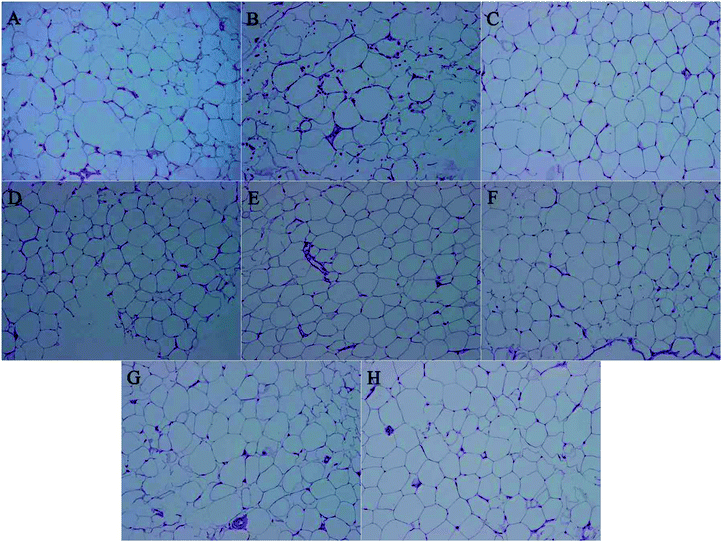 |
| Fig. 3 Histological sections of epididymis fat in different groups. (A) Normal, (B) model, (C) N + FPM, (D) N + NFP, (E) FPL, (F) FPM, (G) FPH, and (H) NFP at 200×. | |
3.6 Oxidative stress
As a primary factor to some metabolic dysfunction, obesity is related to oxidative stress. And obese individuals showed significantly lower activities of such antioxidant enzymes SOD and higher MDA concentration.38 As shown in Table 4, the level of SOD in the model group was lower than that in the normal group (p < 0.01). The FPL group reached the highest level of SOD (p < 0.001). And the level of SOD in the FPM group was closest to 3 times for the NFP group. The results showed that FP more effectively improved the antioxidant enzyme activities than NFP. With MDA concentration, the model group exhibited an increased trend (Table 4). Treatment with FPM and FPH reduced the MDA content by 50.65% (p < 0.01) and 39.05% (p < 0.05) compared with the model group. The NFP group displayed no prominent effect on MDA. The present investigation demonstrated that FP possessed the potent antioxidant activity. In obese individuals, fat accumulation is closely associated with systemic oxidative stress.38 We found that the adiponectin remains in an upward trend in obesity; furthermore, adiponectin levels correlated inversely with systemic oxidative stress,39 and our results fully proved the conclusion that increased oxidative stress in accumulated fat results in a secretion disorder of adipocytokines (Table 3).
Table 3 Effect on the antioxidant enzyme activities and lipid peroxidation of serum in different groups
Groups |
SOD (units) |
MDA (μM L−1) |
* indicates the significant difference compared with model groups, *p < 0.05, **p < 0.01, ***p < 0.001. |
Normal |
2.05 ± 0.16** |
32.16 ± 5.02** |
Model |
0.26 ± 0.04 |
66.83 ± 6.94 |
N + NFP |
2.79 ± 0.12*** |
33.21 ± 5.1** |
N + FPM |
4.14 ± 0.48*** |
20.69 ± 2.7*** |
FPL |
2.47 ± 0.65*** |
42.37 ± 8.98 |
FPM |
0.48 ± 0.12 |
32.98 ± 5.96** |
FPH |
0.53 ± 0.12 |
40.73 ± 4.85* |
NFP |
0.18 ± 0.07 |
58.07 ± 4.08 |
Table 4 24 differential metabolites associated with the risk of obese rats
Metabolite |
Chemical class |
Fold change |
Trenda |
p-Value |
VIP |
FDR |
↑ and ↓ mean the metabolite is up and down regulated in model groups compared with the normal group.
|
Cer 28:1;2 |
Ceramides |
0.26 |
↓ |
4.75 × 10−3 |
1.86 |
2.51 × 10−2 |
Cer 30:0;2 |
Ceramides |
0.06 |
↓ |
1.10 × 10−3 |
1.99 |
7.67 × 10−3 |
Cer 42:0;2 |
Ceramides |
0.07 |
↓ |
1.45 × 10−12 |
2.33 |
1.92 × 10−10 |
DAG 34:0 |
Diacylglycerols |
2.80 |
↑ |
4.42 × 10−3 |
1.38 |
2.43 × 10−2 |
EE 18:3 |
Ethyl esters |
3.53 |
↑ |
5.07 × 10−4 |
1.41 |
3.71 × 10−3 |
FFA 20:4 |
Fatty acids |
3.95 |
↑ |
1.05 × 10−2 |
1.25 |
4.61 × 10−2 |
GlcdE 18:0 |
Glucosylceramide E |
0.31 |
↓ |
1.93 × 10−4 |
1.45 |
2.12 × 10−3 |
GlcdE 20:0 |
Glucosylceramide E |
22.54 |
↑ |
7.95 × 10−3 |
1.85 |
3.75 × 10−2 |
GlcdE 20:4 |
Glucosylceramide E |
6.73 |
↑ |
4.44 × 10−10 |
1.95 |
8.37 × 10−9 |
GlcdE 22:4 |
Glucosylceramide E |
6.76 |
↑ |
2.57 × 10−10 |
1.95 |
5.65 × 10−9 |
LPA 16:1 |
Monoacylglycerophosphates |
3.51 |
↑ |
3.58 × 10−4 |
1.42 |
2.96 × 10−3 |
LPA 20:2 |
Monoacylglycerophosphates |
4.43 |
↑ |
3.11 × 10−4 |
1.83 |
2.94 × 10−3 |
LPS 16:0 |
L-Serine |
0.05 |
↓ |
1.87 × 10−10 |
2.48 |
5.32 × 10−9 |
MAG 16:0 |
Hexadecanoyl-glycerol |
6.29 |
↑ |
2.02 × 10−10 |
1.91 |
5.32 × 10−9 |
MAG 18:0 |
Monoacylglycerols |
3.07 |
↑ |
5.34 × 10−3 |
1.2 |
2.61 × 10−2 |
MAG 18:2 |
Monoacylglycerols |
0.25 |
↓ |
1.62 × 10−4 |
1.54 |
1.95 × 10−3 |
MAG 20:0 |
Monoacylglycerols |
3.65 |
↑ |
3.49 × 10−4 |
1.44 |
2.96 × 10−3 |
MAG 20:3 |
Monoacylglycerols |
6.97 |
↑ |
1.73 × 10−10 |
1.97 |
5.32 × 10−9 |
MAG 20:4 |
Monoacylglycerols |
0.04 |
↓ |
2.38 × 10−9 |
2.54 |
3.15 × 10−8 |
ME 10:0 |
Methyl ethanolamines |
5.94 |
↑ |
9.21 × 10−10 |
1.88 |
1.52 × 10−8 |
ME 12:0 |
Methyl ethanolamines |
5.27 |
↑ |
1.66 × 10−9 |
1.81 |
2.43 × 10−8 |
ME 16:0 |
Methyl ethanolamines |
3.56 |
↑ |
4.03 × 10−4 |
1.42 |
3.13 × 10−3 |
ME 18:2 |
Methyl ethanolamines |
8.03 |
↑ |
1.17 × 10−10 |
2.04 |
5.32 × 10−9 |
MGMG 10:0 |
Glycerolipids |
0.23 |
↓ |
2.13 × 10−4 |
1.56 |
2.16 × 10−3 |
3.7 Lipidomics analysis
Ingestion of M. charantia contributed to a decrease in the TG content of the primary organs, which may be related to lipolysis and lipid oxidation.40 According to the results of serum lipids, FP could ameliorate serum TG in obese rats (p < 0.01). For all treated groups, the epididymal adipose size was smaller than that in the model group. The intervention of FP and NFP caused a chain of physiological reactions to reduce body fat accumulation and improve serum lipids, however the mechanism remains to be elucidated. The previous study demonstrated that plasma metabolites could explain the differences between fermented and non-fermented products in anti-obesity effects.41 Consequently, the lipidomics need to be illuminated for the complicated mechanisms of obesity.
3.8 Detection and identification of biomarker candidates
Identifying biomarkers could give a reliable explanation of the underlying mechanisms associated with obesity. For the serum lipidomic analysis, the PLS-DA model showed a clear separation between the normal and model groups (Fig. 4). R2 (describing the goodness of fit) for the model and Q2 (estimating the predictive power after cross validation) for the prediction in the positive model (R2 = 0.966, Q2 = 0.883) and negative model (R2 = 0.903, Q2 = 0.685) indicated that the PLS-DA model was reliable.
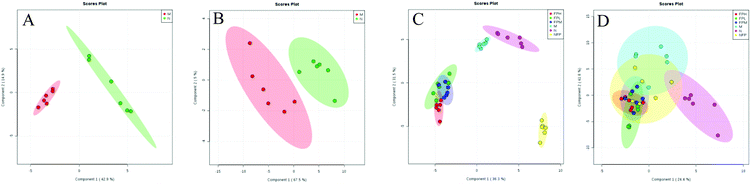 |
| Fig. 4 Lipidomics: PLS-DA score plots between model and normal groups in the positive (A, R2 = 0.966, Q2 = 0.883) and negative (B, R2 = 0.903, Q2 = 0.685) modes; PLS-DA score plots of the M, N, FPL, FPM, FPH and NFP groups in the positive (C, R2 = 0.905, Q2 = 0.868) and negative (D, R2 = 0.652, Q2 = 0.276) modes. | |
Among the 123 metabolites in the multivariate analysis (ESI Table S1†), 55 lipids were significantly altered in the model vs. normal group from lipidomics (ESI Table S2†). The VIP value was applied to find the potential biomarkers among these significantly changed metabolites (generally, metabolites with VIP > 1 can be considered as biomarkers), and 24 of these 55 metabolites were associated with the risk of obesity (with VIP > 1, p < 0.05 and FDR < 0.05) (Table 4). The largest effect size was observed for monoacylglycerols (MAG 20:4) (VIP value = 2.54, FDR = 3.15 × 10−8 and P = 2.38 × 109). These 24 selected biomarkers mainly belonged to the class of ceramides, diacylglycerols, monoacylglycerols, ethyl esters, fatty acids, glucosylceramide E, L-serine, monoacylglycerophosphates, and methyl ethanolamines. The Pearson correlation matrix analysis method was used to find the correlations between these potential biomarkers (Fig. 5), correlation coefficients ranged from −1 to 1, and a value of 0 indicates no correlation. Strong correlations of these related metabolites were found within these groups, and 5 positive correlated metabolites (ceremides, L-serine, MAG 20:4, MAG 18:2, GlcdE 18:0, MGMG 10:0) were negatively related to FFA, LPA, and ME. L-Serine (LPS 16:0) was negatively related to fatty acids (r = −0.79), diacylglycerols (r = −0.57), LPA (r = −0.82), ethyl esters (r = −0.84), and methyl ethanolamines (r = −0.94).
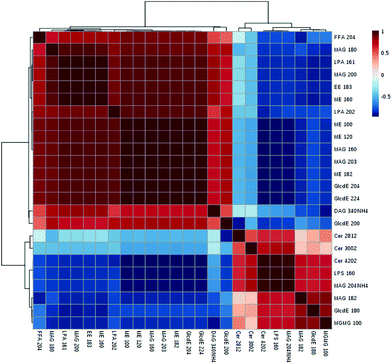 |
| Fig. 5 The overall Pearson's correlation of the significant metabolites based on the 24 metabolites. | |
3.9
M. charantia polysaccharide treatment improved lipid metabolism
The score plots of PLS-DA in positive and negative modes are shown in Fig. 3C and D, and the model group and FP and NFP treatment groups were significantly different from the normal group, suggesting that FP and NFP altered lipid metabolism of plasma of obese rats. However, the model and normal groups were not obviously separated in negative mode, indicating that the positive ion mode was more preferable than the negative mode.
After M. charantia polysaccharide treatment in obese rats, 21 metabolites significantly changed (p < 0.05, significance occurred in at least 2 dosages) (Table 5). For primary glycerolipids, the low- and middle-dose FP treatment notably decreased the levels of monoacylglycerols (MAG 20:0, MAG 20:3), and the levels of diacyglycerols (DAG 28:3, DAG 32:0) were positively correlated with the dose of FP, whereas triacylglycerol hadn't been detected. Higher plasma free fatty acids (FFAs) in the major organs (e.g. adipose, liver, and skeletal) were caused by HFD which was linked to insulin resistance.42 In the present study, the reduced free fatty acids may be associated with the decrease of insulin resistance in high-fat diet rats directly or indirectly. The elevated FFAs are a crucial symbol of obesity-associated metabolic syndrome.43 Regulating FFA levels can be considered as a new therapy for obesity and related metabolic diseases.44 And FP significantly decreased the fatty acyls including free fatty acids, ethyl esters, and methyl ethanolamines (especially by middle and high-dose groups) compared with the model group. In addition, glycerophospholipids and related metabolism were closely associated with obesity.45 Both FP and NFP regulated glycerophospholipids such as monoacylglycero-phosphates and monoacylglycero-phosphoethanolamines and phosphoserines. Phosphoserines were remarkably reduced in all medicated obese rats, whereas phosphoethanolamines were increased. Xia et al.46 found that a negative correlation exists between ceramide levels and adiponectin function, and the reduced ceramides deliver protection against insulin resistance. Interestingly, ceramides and lanosterol class of sphingolipids and sterol lipids were on the rise in the study, respectively. Sphingolipid metabolism was affected by the high-fat diet in obese individuals. The higher ceramide level is mostly due to elevated de novo synthesis, whereby surplus fatty acids provided a continuous substrate supply for the pathway.47 The results imply that M. charantia polysaccharide could improve the lipid metabolism by significantly adjusting the trend of lipids including fatty acyls, glycerolipids, sphingolipids, sterol lipids and glycerophospholipids.
Table 5 21 metabolites that were significantly influenced by M. charantia polysaccharide treatment in obese rats
Metabolites |
Chemical class |
FPL/M |
FPM/M |
FPH/M |
NFP/M |
Fold change |
p-Value |
Fold change |
p-Value |
Fold change |
p-Value |
Fold change |
p-Value |
Cer 32:0;2 |
Ceramides |
10.72 |
1.27 × 10−2 |
3.19 |
6.46 × 10−3 |
— |
— |
— |
— |
DAG 28:3 |
Diacylglycerols |
26.14 |
1.29 × 10−14 |
26.42 |
2.04 × 10−13 |
59.83 |
8.02 × 10−15 |
28.08 |
1.63 × 10−13 |
DAG 32:0 |
Diacylglycerols |
11.12 |
1.23 × 10−2 |
14.13 |
6.15 × 10−4 |
30.15 |
6.73 × 10−4 |
— |
— |
EE 18:2 |
Ethyl esters |
0.05 |
3.02 × 10−15 |
0.06 |
3.10 × 10−17 |
0.03 |
1.54 × 10−17 |
0.06 |
6.74 × 10−16 |
FFA 22:6 |
Fatty acids |
0.06 |
3.55 × 10−4 |
0.07 |
5.36 × 10−4 |
0.04 |
4.75 × 10−4 |
0.07 |
5.39 × 10−4 |
GlcdE 20:0 |
Glucosylceramide E |
6.99 |
1.82 × 10−3 |
8.12 |
1.22 × 10−3 |
10.55 |
1.43 × 10−3 |
11.95 |
5.20 × 10−4 |
Lan 18:1 |
Lanosterol |
10.01 |
1.25 × 10−2 |
— |
— |
21.32 |
1.20 × 10−2 |
14.71 |
5.13 × 10−4 |
LMMPE 10:0 |
Monomethyl PE |
0.06 |
3.33 × 10−4 |
0.07 |
4.96 × 10−4 |
0.03 |
4.44 × 10−4 |
0.07 |
4.98 × 10−4 |
LMMPE 12:0 |
Monomethyl PE |
0.04 |
5.94 × 10−15 |
0.05 |
3.75 × 10−16 |
0.02 |
9.77 × 10−17 |
0.05 |
2.29 × 10−15 |
LPA 16:1 |
Monoacylglycerophosphates |
0.36 |
2.90 × 10−3 |
0.41 |
7.26 × 10−3 |
— |
— |
— |
— |
LPE O-20:0 |
Monoacylglycerophosphoethanolamines |
26.96 |
7.70 × 10−16 |
24.93 |
1.79 × 10−14 |
54.14 |
1.27 × 10−15 |
24.81 |
6.89 × 10−15 |
LPIP 18:1 |
Diacylglycerophosphoinositol monophosphates |
— |
— |
0.16 |
2.10 × 10−4 |
0.15 |
2.96 × 10−4 |
0.05 |
3.34 × 10−14 |
LPS 12:0 |
Monoacylglycerophosphoserines |
0.05 |
2.75 × 10−14 |
0.05 |
3.95 × 10−15 |
0.03 |
7.44 × 10−16 |
0.05 |
1.47 × 10−14 |
LPS 14:0 |
Monoacylglycerophosphoserines |
0.06 |
3.31 × 10−4 |
0.07 |
4.95 × 10−4 |
0.03 |
4.43 × 10−4 |
0.07 |
4.98 × 10−4 |
MAG 20:0 |
Monoacylglycerols |
0.35 |
3.02 × 10−3 |
0.40 |
7.36 × 10−3 |
— |
— |
— |
— |
MAG 20:3 |
Monoacylglycerols |
0.34 |
1.83 × 10−3 |
0.47 |
1.07 × 10−2 |
— |
— |
0.46 |
9.82 × 10−3 |
ME 16:0 |
Methyl ethanolamines |
0.35 |
3.02 × 10−3 |
0.4 |
7.42 × 10−3 |
— |
— |
— |
— |
ME 20:0 |
Methyl ethanolamines |
0.05 |
6.81 × 10−15 |
0.06 |
1.89 × 10−16 |
— |
6.58 × 10−17 |
0.06 |
2.04 × 10−15 |
PA 30:1 |
Monoacylglycerophosphates |
10.53 |
1.23 × 10−2 |
14.10 |
6.10 × 10−4 |
27.04 |
6.82 × 10−4 |
19.13 |
2.26 × 10−14 |
PA 32:1 |
Monoacylglycerophosphates |
— |
— |
14.16 |
1.27 × 10−2 |
33.6 |
6.86 × 10−4 |
12.83 |
1.02 × 10−2 |
PE 32:1 |
Monoacylglycerophosphoethanolamines |
32.71 |
5.58 × 10−13 |
35.00 |
4.43 × 10−14 |
77.28 |
7.49 × 10−15 |
49.48 |
1.34 × 10−13 |
4. Conclusion
The present results demonstrated that the polysaccharides isolated from fermented M. charantia juice have potential anti-obesity effects. Administration of the fermented M. charantia polysaccharides reduced the body weight, Lee's index and ameliorated adipose cell and fat accumulation. FP decreased the levels of serum lipid and oxidative stress and increased the adiponectin content. In addition, treatment with FP and NFP contributed to that some of the lipids associated with obesity such as fatty acids and phosphoserines were reduced, whereas ceramides and diacyglycerols were increased. Consequently, these results demonstrated that FP could inhibit obesity, and fermentation may be considered as one of the effective approaches for improving the ability of polysaccharides to ameliorate obesity.
Conflicts of interest
There are no conflicts to declare.
Acknowledgements
The financial support for this study by the National Key Research and Development Program of China (2017YFD0400705-2) and the Jiangxi Provincial Major Program of Research and Development Foundation (Agriculture field) (20165ABC28004) is acknowledged.
References
- J. J. Heindel, R. Newbold and T. T. Schug, Nat. Rev. Endocrinol., 2015, 11, 653 CrossRef CAS PubMed.
- G. Srivastava and C. M. Apovian, Nat. Rev. Endocrinol., 2018, 14, 12–24 CrossRef CAS PubMed.
- S. B. Heymsfield and T. A. Wadden, N. Engl. J. Med., 2017, 376, 254–266 CrossRef CAS PubMed.
- M. G. Saklayen, Curr. Hypertens. Rep., 2018, 20, 12 CrossRef PubMed.
- D. K. Dahiya, N. Renuka, M. Puniya, U. K. Shandilya, T. Dhewa, N. Kumar, S. Kumar, A. K. Puniya and P. Shukla, Front. Microbiol., 2017, 8, 563 CrossRef PubMed.
- A. A. Farooqi, S. Khalid, F. Tahir, U. Y. Sabitaliyevich, I. Yaylim, R. Attar and B. Xu, Food Chem. Toxicol., 2018, 119, 98–105 CrossRef CAS PubMed.
- J.-H. Han, N. Q. Tuan, M.-H. Park, K. T. Quan, J. Oh, K.-S. Heo, M. Na and C.-S. Myung, Mol. Nutr. Food Res., 2018, 62, e1700769 CrossRef PubMed.
- L. D. Jones, P. Pangloli, H. B. Krishnan and V. P. Dia, Phytomedicine, 2018, 42, 226–232 CrossRef CAS PubMed.
- S. Wang, Z. Li, G. Yang, C.-T. Ho and S. Li, Food Funct., 2017, 8, 1749–1762 RSC.
- H. Gao, J.-J. Wen, J.-L. Hu, Q.-X. Nie, H.-H. Chen, T. Xiong, S.-P. Nie and M.-Y. Xie, Carbohydr. Polym., 2018, 201, 624–633 CrossRef CAS PubMed.
- C. Zhang, H. Chen and W. Bai, Int. J. Biol. Macromol., 2018, 115, 45–52 CrossRef CAS PubMed.
- J.-L. Hu, S.-P. Nie, C. Li, S. Wang and M.-Y. Xie, Food Hydrocolloids, 2018, 76, 60–66 CrossRef CAS.
- W. Lee, N. Kang, E.-A. Kim, H.-W. Yang, J.-Y. Oh, I. P. S. Fernando, K.-N. Kim, G. Ahn and Y.-J. Jeon, J. Funct. Foods, 2017, 28, 83–89 CrossRef CAS.
- F. C. Prado, J. L. Parada, A. Pandey and C. R. Soccol, Food Res. Int., 2008, 41, 111–123 CrossRef CAS.
- T. Xiong, J. Li, F. Liang, Y. Wang, Q. Guan and M. Xie, LWT–Food Sci. Technol., 2016, 69, 169–174 CrossRef CAS.
- T. Xiong, S. Song, X. Huang, C. Feng, G. Liu, J. Huang and M. Xie, J. Food Sci., 2013, 78, M84–M89 CrossRef CAS PubMed.
-
A. M. Staub, Removeal of protein-sevag method, 1965 Search PubMed.
- S. Subramaniam, M. H. B. Rosdi and U. R. Kuppusamy, J. Food Qual., 2017, 2017, 1–9 CrossRef.
- C. Li, S.-P. Nie, Q. Ding, K.-X. Zhu, Z.-J. Wang, T. Xiong, J. Gong and M.-Y. Xie, J. Funct. Foods, 2014, 8, 340–347 CrossRef CAS.
- F. Magkos, G. Fraterrigo, J. Yoshino, C. Luecking, K. Kirbach, S. C. Kelly, L. de las Fuentes, S. He, A. L. Okunade, B. W. Patterson and S. Klein, Cell Metab., 2016, 23, 591–601 CrossRef CAS PubMed.
- M. D. Jensen, D. H. Ryan, C. M. Apovian, J. D. Ard, A. G. Comuzzie, K. A. Donato, F. B. Hu, V. S. Hubbard, J. M. Jakicic and R. F. Kushner, J. Am. Coll. Cardiol., 2014, 63, 2985–3023 CrossRef PubMed.
- S. K. Cho, J. S. Yoon, M. G. Lee, D. H. Lee, L. A. Lim, K. Park, M. S. Park and J. Y. Chung, Clin. Pharmacol. Ther., 2011, 89, 416–421 CrossRef CAS PubMed.
- M. Zhang, L. F. Bi, J. H. Fang, X. L. Su, G. L. Da, T. Kuwamori and S. Kagamimori, Amino Acids, 2004, 26, 267–271 CAS.
- J. L. Sonnenburg and B. Fredrik, Nature, 2016, 535, 56 CrossRef CAS PubMed.
- J. Bai, Y. Zhu and Y. Dong, J. Ethnopharmacol., 2016, 194, 717–726 CrossRef PubMed.
- B.-M. Kim, J.-H. Park, D.-S. Kim, Y.-M. Kim, J.-Y. Jun, I.-H. Jeong and Y.-M. Chi, J. Food Sci., 2016, 81, H1840–H1845 CrossRef CAS PubMed.
- C. Li, Q. Ding, S.-P. Nie, Y.-S. Zhang, T. Xiong and M.-Y. Xie, J. Agric. Food Chem., 2014, 62, 11884–11891 CrossRef CAS PubMed.
- L. G. Cheke, H. M. Bonnici, N. S. Clayton and J. S. Simons, Neuropsychologia, 2017, 96, 137–149 CrossRef PubMed.
- J. Xu, K. Cao, Z. Feng and J. Liu, J. Funct. Foods, 2018, 42, 216–223 CrossRef CAS.
- S. Acar, A. Paketçi, T. Küme, H. Tuhan, Ö. Gürsoy Çalan, K. Demir, E. Böber and A. Abacı, Peptides, 2017, 95, 51–56 CrossRef CAS PubMed.
- M. P. Mollica, G. Mattace Raso, G. Cavaliere, G. Trinchese, C. De Filippo, S. Aceto, M. Prisco, C. Pirozzi, F. Di Guida, A. Lama, M. Crispino, D. Tronino, P. Di Vaio, R. Berni Canani, A. Calignano and R. Meli, Diabetes, 2017, 66, 1405–1418 CrossRef CAS PubMed.
- R. Ricci and F. Bevilacqua, Vet. J., 2012, 191, 292–298 CrossRef CAS PubMed.
-
S. Khosropour, M. Shojaee and P. Lotfi, Obesity, Leptin and Leptin Receptors, 2016 Search PubMed.
- R. V. Considine, M. K. Sinha, M. L. Heiman, A. Kriauciunas, T. W. Stephens, M. R. Nyce, J. P. Ohannesian, C. C. Marco, L. J. McKee and T. L. Bauer, N. Engl. J. Med., 1996, 334, 292–295 CrossRef CAS PubMed.
- K. Hotta, T. Funahashi, N. L. Bodkin, H. K. Ortmeyer, Y. Arita, B. C. Hansen and Y. Matsuzawa, Diabetes, 2001, 50, 1126 CrossRef CAS PubMed.
- X. Huang and Z. Yang, J. Endocrinol. Invest., 2016, 39, 607–615 CrossRef CAS PubMed.
- C. J. Chang, C. C. Lu, C. S. Lin, J. Martel, Y. F. Ko, D. M. Ojcius, T. R. Wu, Y. H. Tsai, T. S. Yeh and J. J. Lu, Int. J. Obes., 2017, 42 CAS.
- H. K. Vincent and A. G. Taylor, Int. J. Obes., 2005, 30, 400 CrossRef PubMed.
- S. Furukawa, T. Fujita, M. Shimabukuro, M. Iwaki, Y. Yamada, Y. Nakajima, O. Nakayama, M. Makishima, M. Matsuda and I. Shimomura, J. Clin. Invest., 2004, 114, 1752–1761 CrossRef CAS PubMed.
- Q. Chen and E. T. S. Li, Br. J. Nutr., 2007, 93, 747 CrossRef.
- D. H. Suh, E. S. Jung, H. M. Park, S. H. Kim, S. Lee, Y. H. Jo, M. K. Lee, G. Jung, S.-G. Do and C. H. Lee, PLoS One, 2016, 11, e0149022 CrossRef PubMed.
- G. Boden, Exp. Clin. Endocrinol. Diabetes, 2003, 111, 121–124 CrossRef CAS PubMed.
- G. Boden, Curr. Opin. Endocrinol., Diabetes Obes., 2011, 18, 139–143 CrossRef CAS PubMed.
- J. Kusunoki, A. Kanatani and D. E. Moller, Endocrine, 2006, 29, 91–100 CrossRef CAS PubMed.
- L. K. Heilbronn, A. C. F. Coster, L. V. Campbell, J. R. Greenfield, K. Lange, M. J. Christopher, P. J. Meikle and D. Samocha-Bonet, Obesity, 2013, 21, E649–E659 CrossRef CAS PubMed.
- J. Y. Xia, W. L. Holland, C. M. Kusminski, K. Sun, A. X. Sharma, M. J. Pearson, A. J. Sifuentes, J. G. McDonald, R. Gordillo and P. E. Scherer, Cell Metab., 2015, 22, 266–278 CrossRef CAS PubMed.
- S. Choi and A. J. Snider, Mediators Inflammation, 2015, 2015, 12 Search PubMed.
Footnote |
† Electronic supplementary information (ESI) available. See DOI: 10.1039/c8fo01609g |
|
This journal is © The Royal Society of Chemistry 2019 |
Click here to see how this site uses Cookies. View our privacy policy here.