DOI:
10.1039/C8FO02004C
(Paper)
Food Funct., 2019,
10, 216-223
Betaine increases mitochondrial content and improves hepatic lipid metabolism
Received
13th October 2018
, Accepted 3rd December 2018
First published on 4th December 2018
Abstract
The liver plays a critical role in lipid metabolism. Hepatic dysfunction is not only the direct cause of fatty liver disease, but the main risk factor for obesity, diabetes, and other metabolic diseases. So far, therapeutic strategies against fatty liver disease are very limited. Betaine is a methyl donor. Current studies reported that the intake of betaine decreases body fat and is beneficial for treatment of fatty liver disease and metabolic syndrome. However, the underlying mechanisms remain largely unknown. In this study, to investigate the role of betaine on hepatic lipid metabolism and explore the underlying mechanism, HepG2 cells were cultured with fatty acids and betaine. The data indicated that betaine inhibited hepatic fat accumulation and promoted mitochondrial content and activity, suggesting that betaine is involved in the regulation of lipid and energy metabolism. Gene expression analysis implied that betaine inhibits fatty acid synthesis, but stimulates fatty acid oxidation and lipid secretion. Further, to study the mechanism of betaine, FTO (RNA demethylase) and its mutant (loss of demethylase activity) were used. The results showed that FTO blocked the ability of betaine to regulate lipid metabolism and mitochondrial content, but the FTO mutant had no effect, suggesting that betaine influences RNA methylation. This work links betaine administration with mitochondrial activity and RNA methylation, and provides a potential target for the development of new therapeutic strategies for the treatment of fatty liver disease.
1. Introduction
The liver is a key metabolic organ that not only utilizes lipids to produce energy, but also secretes lipids in the form of very low-density lipoproteins (VLDLs). The liver also plays a dual function in lipid metabolism. NAFLD is a liver lipid metabolism disorder.1 However, measures to prevent and treat this type of metabolic disorder, especially by diet modification, remain unclear.
Betaine, also known as trimethylglycine, can promote methylation as a methyl donor and is widely distributed in marine invertebrates, plants, and animals.2 Growing evidence indicates that betaine is beneficial for the treatment of NAFLD,3 cardiovascular disease,4 metabolic syndrome,5 and cancer.6 In addition, clinical trials have shown that betaine is a safe and well tolerated drug for the treatment of nonalcoholic fatty liver disease.7
A recent human study demonstrated that the intake of betaine was negatively correlated with body fat, body mass index, and waist circumference.8 In an animal model, betaine impaired the accumulation of lipids from high-fat diets. Metabolomic analysis revealed that carnitine levels were significantly elevated after betaine supplementation, suggesting that betaine is involved in the regulation of lipid metabolism through carnitine.9 Moreover, betaine was found to inhibit the expression of monoacylglycerol transferase, which is a key enzyme in triglyceride (TG) synthesis.3 Gene expression analysis showed that betaine significantly stimulated fibroblast growth factor 21 (Fgf21) expression levels both in the liver and blood. However, treatment of fgf21−/− mice with betaine did not improve glucose levels or fat deposition, indicating that Fgf21 is indispensable in the role of betaine.10 The data from adipose tissue showed that betaine decreased expression of the stress-regulated transcription factor CHOP and inhibited the activation of the c-Jun N-terminal kinase pathway, thereby alleviating obesity-induced stress to the endoplasmic reticulum.11
As a methyl donor, betaine significantly increases RNA methylation levels,12 suggesting that betaine plays a role in epigenetic modification. N6-methyladenosine (m6A) is the most common form of methylation in RNA. Current studies have demonstrated that m6A is closely related to lipid accumulation. In 2007, the fat mass and obesity-related genes (FTO) was discovered13 and subsequently identified as a demethylase, which removes m6A from mRNA.14 It has been reported that FTO promotes adipocyte differentiation and fat deposition by influencing m6A levels.15,16 Interestingly, changing of m6A levels with a methylation inhibitor or methyl donor can also regulate fat deposition.12 These results confirmed m6A plays an important role in the regulation of lipid metabolism.
In summary, betaine enhances m6A levels of RNA and m6A is involved in lipid metabolism. However, whether betaine regulates lipid metabolism by inducing changes in m6A levels remains unclear. Here, the effects of betaine and FTO (m6A demethylase) on hepatic lipid accumulation and energy metabolism were investigated. The findings of this study revealed a link between betaine and mitochondrial activity and clarified the underlying mechanism of betaine.
2 Materials and methods
2.1 Materials
TRIzol reagent and a quantitative protein assay kit were purchased from Thermo-Fisher Scientific (Waltham, MA, USA). PolyJet Transfection Reagent was acquired from SignaGen Laboratories (Rockville, MD, USA). Betaine was purchased from Beijing Solarbio Science & Technology Co., Ltd (Beijing, China). Penicillin–streptomycin solution, oil red O, oleic acid, palmitic acid, fetal bovine serum, and bovine serum albumin (BSA) were acquired from Guangxi Xilong Chemical Co., Ltd (Guangxi, China). A tissue TG assay kit was purchased from Pulilai Biological Technology Co. Ltd (Beijing, China). The SYBR Green PCR Kit was acquired from TaKaRa Biotechnology (Dalian) Co., Ltd (Dalian, China).
2.2 Cell culture and transfection
HepG2 cells were maintained in Dulbecco's modified Eagle's medium (DMEM) supplemented with 10% fetal bovine serum under an atmosphere of 5% CO2 at 37 °C. The coding sequence of the FTO gene was amplified by PCR and inserted into the PCMV-Tag2B vector. At 80% confluence, the cells were transfected with the PCMV-Tag2B-FTO vector using PolyJet Transfection Reagent. An empty PCMV-Tag2B plasmid was used as a control and another group of cells was transfected with the PcDNA3.1-FTO-mutant (R316A) vector using PolyJet Transfection Reagent. An Empty PcDNA3.1 plasmid was used as a control. After 5 h of incubation, the medium was changed to DMEM supplemented with oleic (200 μM) and palmitic (100 μM) acids. The cells were collected after 24 h of incubation. AML12 cells were maintained in Dulbecco's modified Eagle's mediudam's F-12 supplemented with 10% fetal bovine serum, ITS Liquid Media Supplement, and dexamethasone(40 ng ml−1) under an atmosphere of 5% CO2 at 37 °C. At 80% confluence the medium was changed to DMEM/F-12 supplemented with oleic (200 μM) and palmitic (100 μM) acids. The cells were collected after 24 h of incubation.
2.3 RNA extraction and quantitative PCR
Total RNA of tissues and cells was extracted using TRIZOL reagent and cDNA was synthesized using a commercial reverse transcription kit (Takara Bio, Inc., Shiga, Japan). Gene expression levels were measured by quantitative PCR using the 2−ΔΔCt method with β-actin as an internal control.
2.4 DNA isolation and quantitative PCR analysis of mitochondria
DNA was isolated from cultured cells using PCR lysis buffer.17 The relative content of mitochondrial DNA (mtDNA) was determined by quantitative PCR. The ratio of mtDNA to nuclear DNA (nDNA) reflects the content of the mitochondria.
2.5 Adenosine triphosphate (ATP) assay
ATP levels were determined using an ATP Assay Kit (Beyotime Institute of Biotechnology, Haimen, China) according to the manufacturer's instructions. Briefly, cells were lysed and 200 μL aliquots (100 μL of each supernatant mixed with 100 μL of ATP detection working dilution) were used to determine ATP levels. Values were normalized to protein concentrations that were measured using the Pierce BCA protein quantitative assay kit (Thermo Fisher Scientific).
2.6 Staining and observation
Cells were seeded in 24-well culture plates. After fixation, the cells were washed and treated with 0.5% oil red O for 30 min. Then, the cells were washed again and stained with 5 μM 4,6-diamidino-2-phenylindole (DAPI) for 10 min. Afterward, the cells were rinsed again and stained for 10 min with 5 μM DAPI. Finally, the cells were washed three times and observed under a fluorescence microscope (IX53; Olympus Corporation, Tokyo, Japan).
2.7 TG detection
The TG content was measured using a TG assay kit in accordance with the manufacturer's instructions. Briefly, cells and tissues were lysed and 10 μL of lysate were mixed with 190 μL of working fluid. The reaction was carried out at 37 °C. The results were determined with a microplate reader and normalized against the protein concentration.
2.8 Statistical analysis
The results are presented as the mean ± standard deviation (SD). Each experiment was performed in triplicate. Statistical analysis was performed using the unpaired two-tailed t-test (for two groups) or analysis of variance (for multiple groups). A probability (p) value of <0.05 was considered statistically significant.
3 Results
3.1 Betaine influences lipid and energy metabolism
To study the effect of betaine on lipid metabolism, 2 mM betaine was used to treat HepG2 cells and AML12 cells supplemented with oleic acid (200 μM) and palmitic acid (100 μM). After 24 h of treatment, intracellular and extracellular TG levels were measured. The oil red O staining of HepG2 cells showed that the dyeing signal was greater in the control group than the betaine-treated group, indicating that betaine significantly decreased cellular lipid accumulation (Fig. 1A). Quantitative data of TG levels confirmed this finding and demonstrated that betaine reduced intracellular lipid content and increased extracellular lipid content (Fig. 1B and C). And in AML12 cells we obtained a similar data (Fig. 1D and E), suggesting that betaine inhibits lipid deposition and promotes lipid secretion. Mitochondria are critical organelles in lipid metabolism. Therefore, the effect of betaine on mitochondrial content and activity was tested. Mito-Tracker Green was used to stain intracellular mitochondria. The data showed that betaine treatment augmented the fluorescent signals, indicating that betaine increased mitochondrial content (Fig. 2A and B). Afterwards, quantitative data of mtDNA were consistent and verified the effect of betaine administration on mitochondrial content (Fig. 2C). Further, to study the role of betaine on mitochondrial function, cellular ATP levels were measured, which showed that betaine promoted ATP levels, suggesting that betaine increases mitochondrial activity (Fig. 2D). The consistent result was confirmed in AML12 cells (Fig. 2E).
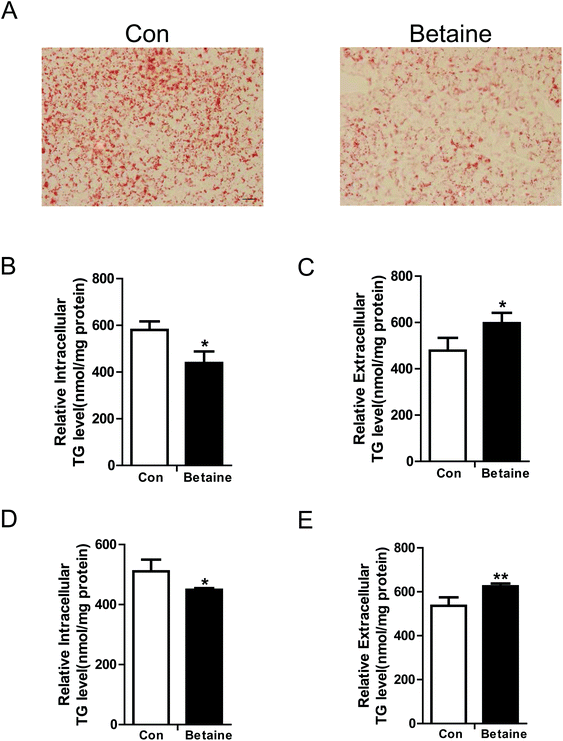 |
| Fig. 1 Betaine attenuates hepatic lipid accumulation. (A) Red oil O staining of HepG2 cells. (B) Intracellular and (C) extracellular TG levels of HepG2 cells. (A–C) HepG2 cells were cultured in DMEM supplemented with oleic (200 μM) and palmitic (100 μM) acids, and BSA (75 μM). (D) Intracellular and (E) extracellular TG levels of AML12 cells. (D–E) AML12 cells were cultured in DMEM/F-12 supplemented with oleic (200 μM) and palmitic (100 μM) acids, and BSA (75 μM). The control (Con) and Betaine groups were treated without or with 2 mM betaine for 24 h. Then, the cells and medium were collected for staining or detection. The cell lysate and medium were used to measure intracellular and extracellular TG levels, respectively. TG levels were normalized to the protein concentration. Bar, 20 μm. Each experiment was performed at least three times. Data are presented as the mean ± SD. *p < 0.05; **p < 0.01. | |
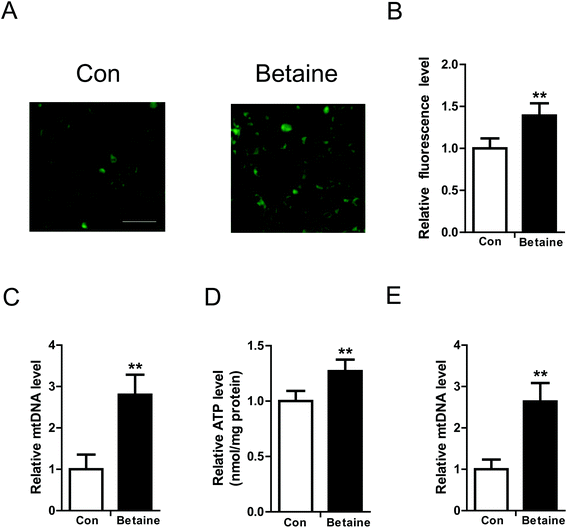 |
| Fig. 2 Betaine improves mitochondrial function. (A) Fluorescence images stained with Mito-Tracker Green. (B) Relative quantification of mitochondrial fluorescence intensity. (C) Relative mtDNA levels. (D) Cellular ATP levels. (E) Relative mtDNA level in AML12 cells. HepG2 cells and AML12 cells were cultured with fatty acids and without (Con) or with betaine (Betaine). Mitochondrial content was detected by Mito-Tracker Green staining. The fluorescence intensity was measured and quantified using a fluorescent microplate reader. The relative content of mtDNA was tested by real-time PCR. The ratio of mtDNA to nDNA reflects mitochondrial content. ATP levels were determined using an ATP Assay Kit (Beyotime Institute of Biotechnology). Bar, 50 μm. Each experiment was performed at least three times. Data are presented as the mean ± SD. **p < 0.01. | |
3.2 The effect of betaine on gene expression
Our data revealed that betaine regulates lipid metabolism. However, the underlying mechanisms remain unclear. To clarify this issue, the relative expression levels of some genes were detected. On the one hand, the genes involved in the synthesis of fatty acid and TGs were identified by real-time PCR. The data indicated that betaine inhibited the expression of the genes FASN, SCD1, ACC1, and SREBP1, which are involved with fatty acid synthesis (Fig. 3A), and attenuated the expression of monoacylglycerol transferase, which catalyzes the synthesis of TGs. On the other hand, betaine stimulated the expression of CPT1α and PGC1α, which are involved with fatty acid oxidation, and also upregulated the mRNA levels of microsomal triglyceride transfer protein (MTTP) and apolipoprotein B (ApoB), which are involved in VLDL secretion. These results suggest that betaine regulates lipid metabolism by inhibiting fatty acid synthesis and promoting fatty acid oxidation and lipid secretion (Fig. 3A). Meanwhile, recent studies have reported that m6A of RNA is closely related to lipid metabolism. Considering that betaine promotes methylation as a methyl donor, the expression levels of the RNA methylases METTL3 and METTL14 and the demethylases FTO and ALKBH5 were measured. The data showed that betaine suppressed expression of the RNA methylases, but stimulated those of the demethylases (Fig. 3B), suggesting that betaine influences the homeostasis of RNA methylation and the cells invoke a feedback loop in response to this change.
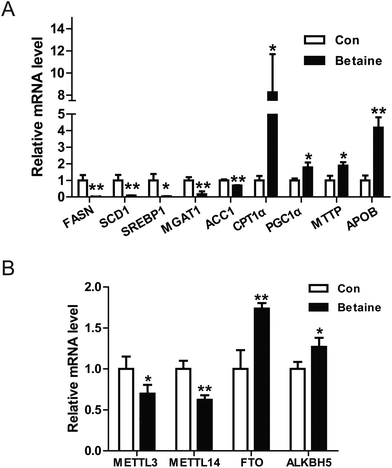 |
| Fig. 3 Betaine influences gene transcription. (A) Effect of betaine on the transcription of lipid metabolism-associated genes. (B) Effect of betaine on the transcription of RNA methylation-associated genes. Total RNA was isolated from HepG2 cells and purified with TRIzol reagent (Thermo Fisher Scientific). Relative mRNA levels were quantified by real-time PCR. Each experiment was performed at least three times. Data are presented as the mean ± SD. *p < 0.05; **p < 0.01. | |
3.3 RNA methylation is involved in betaine activities
FTO is an RNA demethylase. Some groups have reported that FTO regulates lipid metabolism by influencing m6A levels. Considering that betaine also regulates methylation, further investigations are warranted to determine whether RNA methylation is involved in betaine activities. To change cellular RNA methylation, FTO and its mutant (loss of demethylase activity)18 were used. Our data indicated that FTO overexpression blocked the action of betaine, which increased intracellular TG levels and decreased mitochondrial content (Fig. 4A and B), revealing a negative association between mitochondrial content and TG levels. To further confirm this relationship, an FTO mutant (R316A) with no demethylase activity was used. Interestingly, R316A had no effect on TG levels or mitochondrial content (Fig. 4C and D). These data confirmed the link between m6A and TG levels and suggested that betaine regulates RNA methylation.
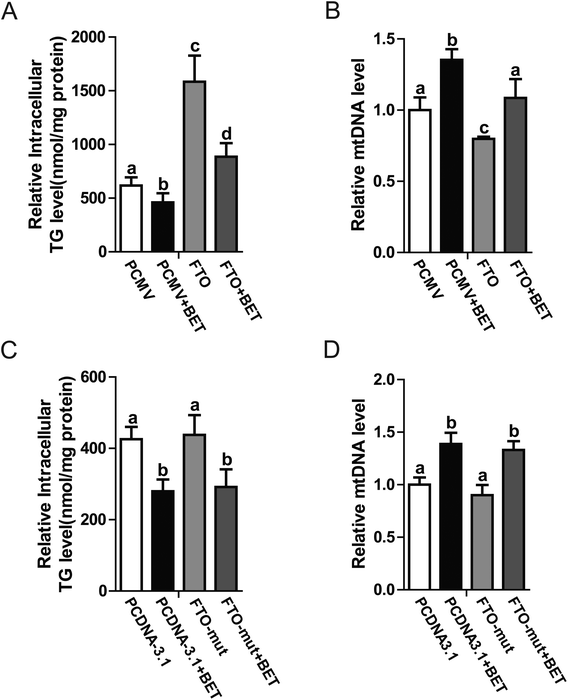 |
| Fig. 4 The effect of RNA methylation on betaine activities. (A) Intracellular TG levels in response to treatment with betaine and FTO. (B) Relative mtDNA levels in response to treatment with betaine and FTO. (A–B) The control (Con) and betaine (BET) groups were treated with or without betaine and transfected with PCMV-Tag2B (blank vector). The FTO (FTO) and FTO + betaine (FTO + BET) groups were treated with or without betaine and transfected with FTO. (C) Intracellular TG levels in response to treatment with betaine and the FTO mutant. (D) Relative mtDNA levels in response to treatment with betaine and the FTO mutant (R316A). (C–D) The control (Con) and betaine (BET) groups were treated with or without betaine and transfected with PcDNA3.1 (blank vector). The FTO mutant (FTO-mut) and FTO mutant (R316A) + betaine (FTO-mut + BET) groups were treated with or without betaine and transfected with FTO (R316A). All groups were cultured in DMEM supplemented with oleic (200 μM) and palmitic (100 μM) acids, and BSA (75 μM). Each experiment was performed at least three times. Data are presented as the mean ± SD. *p < 0.05; **p < 0.01. Values with different letters are significantly different. | |
4 Discussion
NAFLD is a lipid metabolic disorder in the liver. Excessive fat accumulation in the liver will lead to local and systematic issues. Currently, there is no effective approach to prevent and treat NAFLD. Accumulating evidence has indicated that betaine supplementation of food is a promising strategy to improve hepatic lipid metabolism,19 however, the underlying mechanism is largely unknown.
In this study, oleic and palmitic acids were used to treat the cells and create a high-fat model in vitro.20 Measurements of TG levels showed that betaine administration dramatically inhibited lipid accumulation (Fig. 1A), which was confirmed by quantitative analysis (Fig. 1B and D). This result is consistent with other studies, which found that betaine can improve hepatic steatosis.21,22 Further, because mitochondria are the main sites of energy metabolism in the cell and play a key role in fatty acid oxidation, we tested the effect of betaine on mitochondrial content and activity. The data supported our hypothesis that betaine enhances both mitochondrial content and ATP levels (Fig. 2A–E). Civitarese et al. also reported a similar trend that calorie restriction reduces fat mass and increases mitochondria.23
Interestingly, betaine also increases extracellular TG levels (Fig. 1C and E), suggesting it stimulates lipid secretion, in line with the results of previous studies.24,25 So, we measured the expression levels of associated genes, such as MTTP, which encodes microsomal TG transfer protein and plays a central role in VLDL assembly and lipid secretion.26 Our data showed that betaine significantly up-regulated MTTP expression (Fig. 3A). Similar results were found for APOB, implying that betaine promotes lipid secretion via mediating lipid assembly.
Betaine is an important methyl donor. Although it is reported that betaine is closely associated with m6A levels,27,28 the underlying mechanism is not very clear. FTO is an RNA demethylase14 and up-regulated by betaine (Fig. 3B). Zhao et al. reported that FTO plays a key role in the regulation of lipogenesis by regulating the RNA binding ability of SRSF2 and m6A demethylation to control selective splicing of mRNA.15 To investigate the regulatory mechanism of betaine, FTO and its mutant were used. Overexpression of FTO prevented the effect of betaine, which increased intracellular TG levels and decreased mitochondrial content (Fig. 4A and B). However, overexpression of the FTO (R316A) mutant,18 which cannot remove m6A, had no effect on the regulation of mitochondrial content and TG levels (Fig. 4C and D). These data revealed the relationship between betaine and m6A levels and supported that hypothesis that betaine functions by influencing RNA methylation. However, due to this work solely on in vitro studies, more in vivo studies need to be done to confirm the action of betaine.
5 Conclusions
In summary, betaine promotes mitochondrial activity by mediating RNA methylation and regulates lipid accumulation via influencing energy metabolism. This work links epigenetic modification with energy metabolism via betaine and reveals the underlying mechanism of betaine, suggesting RNA methylation is a potential target for hepatic lipid metabolism.
Author contributions
Lifang Zhang and Lei Zhou conceived the project and designed the protocol; Lifang Zhang, Yilin Qi, Zhier ALuo, Siqi Liu and Zhiwang Zhang performed the experiments; Lifang Zhang and Lei Zhou wrote the manuscript. All authors read and approved the final manuscript.
Conflicts of interest
The authors have no competing interests to declare.
Acknowledgements
This work was supported by the grants from National Key R&D Program of China (2018YFD0500402), National Natural Science Foundation of China (31660641), Guangxi Natural Science Foundation (2017GXNSFAA198139), Guangxi Hundred-Talent Program, State Key Laboratory for Conservation and Utilization of Subtropical Agro-bioresources (SKLCUSA-a201808), and Scientific Research Foundation of Guangxi University (XTZ130719).
References
- D. R. LaBrecque, Z. Abbas, F. Anania, P. Ferenci, A. G. Khan, K. L. Goh, S. S. Hamid, V. Isakov, M. Lizarzabal, M. M. Penaranda, J. F. Ramos, S. Sarin, D. Stimac, A. B. Thomson, M. Umar, J. Krabshuis and A. LeMair, World Gastroenterology Organisation global guidelines: Nonalcoholic fatty liver disease and nonalcoholic steatohepatitis, J. Clin. Gastroenterol., 2014, 48, 467–473 Search PubMed.
- M. Lever and S. Slow, The clinical significance of betaine, an osmolyte with a key role in methyl group metabolism, Clin. Biochem., 2010, 43, 732–744 CrossRef CAS.
- R. Deminice, R. P. da Silva, S. G. Lamarre, K. B. Kelly, R. L. Jacobs, M. E. Brosnan and J. T. Brosnan, Betaine supplementation prevents fatty liver induced by a high-fat diet: effects on one-carbon metabolism, Amino acids, 2015, 47, 839–846 CrossRef CAS PubMed.
- S. Rajaie and A. Esmaillzadeh, Dietary choline and betaine intakes and risk of cardiovascular diseases: review of epidemiological evidence, ARYA Atheroscler., 2011, 7, 78–86 Search PubMed.
- S. V. Konstantinova, G. S. Tell, S. E. Vollset, O. Nygard, O. Bleie and P. M. Ueland, Divergent associations of plasma choline and betaine with components of metabolic syndrome in middle age and elderly men and women, J. Nutr., 2008, 138, 914–920 CrossRef CAS PubMed.
- P. M. Ueland, Choline and betaine in health and disease, J. Inherited Metab. Dis., 2011, 34, 3–15 CrossRef CAS PubMed.
- M. F. Abdelmalek, P. Angulo, R. A. Jorgensen, P. B. Sylvestre and K. D. Lindor, Betaine, a promising new agent for patients with nonalcoholic steatohepatitis: results of a pilot study, Am. J. Gastroenterol., 2001, 96, 2711 CrossRef CAS PubMed.
- X. Gao, Y. Wang, E. Randell, P. Pedram, Y. Yi, W. Gulliver and G. Sun, Higher Dietary Choline and Betaine Intakes Are Associated with Better Body Composition in the Adult Population of Newfoundland, Canada, PLoS One, 2015, 11, e0155403 CrossRef PubMed.
- J. Pekkinen, K. Olli, A. Huotari, K. Tiihonen, P. Keski-Rahkonen, M. Lehtonen, S. Auriola, M. Kolehmainen, H. Mykkanen, K. Poutanen and K. Hanhineva, Betaine supplementation causes increase in carnitine metabolites in the muscle and liver of mice fed a high-fat diet as studied by nontargeted LC-MS metabolomics approach, Mol. Nutr. Food Res., 2013, 57, 1959–1968 CrossRef CAS PubMed.
- A. Ejaz, L. Martinez-Guino, A. B. Goldfine, F. Ribas-Aulinas, V. De Nigris, S. Ribo, A. Gonzalez-Franquesa, P. M. Garcia-Roves, E. Li, J. M. Dreyfuss, W. Gall, J. K. Kim, T. Bottiglieri, F. Villarroya, R. E. Gerszten, M. E. Patti and C. Lerin, Dietary Betaine Supplementation Increases Fgf21 Levels to Improve Glucose Homeostasis and Reduce Hepatic Lipid Accumulation in Mice, Diabetes, 2016, 65, 902–912 CrossRef CAS PubMed.
- Z. Wang, T. Yao, M. Pini, Z. Zhou, G. Fantuzzi and Z. Song, Betaine improved adipose tissue function in mice fed a high-fat diet: a mechanism for hepatoprotective effect of betaine in nonalcoholic fatty liver disease, Am. J. Physiol., 2010, 298, G634–G642 CAS.
- J. Chen, X. Zhou, W. Wu, X. Wang and Y. Wang, FTO-dependent function of N6-methyladenosine is involved in the hepatoprotective effects of betaine on adolescent mice, J. Physiol. Biochem., 2015, 71, 405–413 CrossRef CAS.
- T. M. Frayling, N. J. Timpson, M. N. Weedon, E. Zeggini, R. M. Freathy, C. M. Lindgren, J. R. Perry, K. S. Elliott, H. Lango, N. W. Rayner, B. Shields, L. W. Harries, J. C. Barrett, S. Ellard, C. J. Groves, B. Knight, A. M. Patch, A. R. Ness, S. Ebrahim, D. A. Lawlor, S. M. Ring, Y. Ben-Shlomo, M. R. Jarvelin, U. Sovio, A. J. Bennett, D. Melzer, L. Ferrucci, R. J. Loos, I. Barroso, N. J. Wareham, F. Karpe, K. R. Owen, L. R. Cardon, M. Walker, G. A. Hitman, C. N. Palmer, A. S. Doney, A. D. Morris, G. D. Smith, A. T. Hattersley and M. I. McCarthy, A common variant in the FTO gene is associated with body mass index and predisposes to childhood and adult obesity, Science, 2007, 316, 889–894 CrossRef CAS PubMed.
- G. Jia, Y. Fu, X. Zhao, Q. Dai, G. Zheng, Y. Yang, C. Yi, T. Lindahl, T. Pan, Y. G. Yang and C. He, N6-methyladenosine in nuclear RNA is a major substrate of the obesity-associated FTO, Nat. Chem. Biol., 2011, 7, 885–887 CrossRef CAS.
- X. Zhao, Y. Yang, B. F. Sun, Y. Shi, X. Yang, W. Xiao, Y. J. Hao, X. L. Ping, Y. S. Chen, W. J. Wang, K. X. Jin, X. Wang, C. M. Huang, Y. Fu, X. M. Ge, S. H. Song, H. S. Jeong, H. Yanagisawa, Y. Niu, G. F. Jia, W. Wu, W. M. Tong, A. Okamoto, C. He, J. M. Rendtlew Danielsen, X. J. Wang and Y. G. Yang, FTO-dependent demethylation of N6-methyladenosine regulates mRNA splicing and is required for adipogenesis, Cell Res., 2014, 24, 1403–1419 CrossRef CAS PubMed.
- M. Merkestein, S. Laber, F. McMurray, D. Andrew, G. Sachse, J. Sanderson, M. Li, S. Usher, D. Sellayah, F. M. Ashcroft and R. D. Cox, FTO influences adipogenesis by regulating mitotic clonal expansion, Nat. Commun., 2015, 6, 6792 CrossRef CAS PubMed.
- L. Zhou, X. Yu, Q. Meng, H. Li, C. Niu, Y. Jiang, Y. Cai, M. Li, Q. Li, C. An, L. Shu, A. Chen, H. Su, Y. Tang, S. Yin, S. Raschke, K. Eckardt, J. Eckel and Z. Yang, Resistin reduces mitochondria and induces hepatic steatosis in mice by the protein kinase C/protein kinase G/p65/PPAR gamma coactivator 1 alpha pathway, Hepatology, 2013, 57, 1384–1393 CrossRef CAS PubMed.
- T. Gerken, C. A. Girard, Y. C. Tung, C. J. Webby, V. Saudek, K. S. Hewitson, G. S. Yeo, M. A. McDonough, S. Cunliffe, L. A. McNeill, J. Galvanovskis, P. Rorsman, P. Robins, X. Prieur, A. P. Coll, M. Ma, Z. Jovanovic, I. S. Farooqi, B. Sedgwick, I. Barroso, T. Lindahl, C. P. Ponting, F. M. Ashcroft, S. O'Rahilly and C. J. Schofield, The obesity-associated FTO gene encodes a 2-oxoglutarate-dependent nucleic acid demethylase, Science, 2007, 318, 1469–1472 CrossRef CAS PubMed.
- C. R. Day and S. A. Kempson, Betaine chemistry, roles, and potential use in liver disease, Biochim. Biophys. Acta, 2016, 1860, 1098–1106 CrossRef CAS PubMed.
- H. Li, A. Chen, L. Shu, X. Yu, L. Gan, L. Zhou and Z. Yang, Translocation of CIDEC in hepatocytes depends on fatty acids, Genes Cells, 2014, 19, 793–802 CrossRef CAS.
- C. X. Ge, R. Yu, M. X. Xu, P. Q. Li, C. Y. Fan, J. M. Li and L. D. Kong, Betaine prevented fructose-induced NAFLD by regulating LXRalpha/PPARalpha pathway and alleviating ER stress in rats, Eur. J. Pharmacol., 2016, 770, 154–164 CrossRef CAS PubMed.
- S. Sookoian, P. Puri, G. O. Castano, R. Scian, F. Mirshahi, A. J. Sanyal and C. J. Pirola, Nonalcoholic steatohepatitis is associated with a state of betaine-insufficiency, Liver Int., 2017, 37, 611–619 CrossRef CAS PubMed.
- A. E. Civitarese, S. Carling, L. K. Heilbronn, M. H. Hulver, B. Ukropcova, W. A. Deutsch, S. R. Smith, E. Ravussin and C. P. Team, Calorie restriction increases muscle mitochondrial biogenesis in healthy humans, PLoS Med., 2007, 4, e76 CrossRef PubMed.
- K. K. Kharbanda, D. D. Rogers 2nd, M. E. Mailliard, G. L. Siford, A. J. Barak, H. C. Beckenhauer, M. F. Sorrell and D. J. Tuma, A comparison of the effects of betaine and S-adenosylmethionine on ethanol-induced changes in methionine metabolism and steatosis in rat hepatocytes, J. Nutr., 2005, 135, 519–524 CrossRef CAS PubMed.
- K. K. Kharbanda, M. E. Mailliard, C. R. Baldwin, H. C. Beckenhauer, M. F. Sorrell and D. J. Tuma, Betaine attenuates alcoholic steatosis by restoring phosphatidylcholine generation via the phosphatidylethanolamine methyltransferase pathway, J. Hepatol., 2007, 46, 314–321 CrossRef CAS PubMed.
- C. P. Oliveira, J. T. Stefano, A. M. Cavaleiro, M. A. Zanella Fortes, S. M. Vieira, V. M. Rodrigues Lima, T. E. Santos, V. N. Santos, A. L. de Azevedo Salgado, E. R. Parise, V. A. Ferreira Alves, F. J. Carrilho and M. L. Correa-Giannella, Association of polymorphisms of glutamate-cystein ligase and microsomal triglyceride transfer protein genes in non-alcoholic fatty liver disease, J. Gastroenterol. Hepatol., 2010, 25, 357–361 CrossRef CAS PubMed.
- X. Wang, L. Zhu, J. Chen and Y. Wang, mRNA m(6)A methylation downregulates adipogenesis in porcine adipocytes, Biochem. Biophys. Res. Commun., 2015, 459, 201–207 CrossRef CAS.
- X. Zhou, J. Chen, J. Chen, W. Wu, X. Wang and Y. Wang, The beneficial effects of betaine on dysfunctional adipose tissue and N6-methyladenosine mRNA methylation requires the AMP-activated protein kinase alpha1 subunit, J. Nutr. Biochem., 2015, 26, 1678–1684 CrossRef CAS.
|
This journal is © The Royal Society of Chemistry 2019 |
Click here to see how this site uses Cookies. View our privacy policy here.