DOI:
10.1039/C8FO01777H
(Paper)
Food Funct., 2019,
10, 125-139
Dietary wild bitter gourd displays selective androgen receptor modulator like activity and improves the muscle decline of orchidectomized mice†
Received
10th September 2018
, Accepted 10th December 2018
First published on 11th December 2018
Abstract
Loss of skeletal muscle mass and strength is often associated with disability and poor quality of life. Selective Androgen Receptor Modulators (SARMs) are under development as potential treatment. This study aims at examining the potential of wild bitter gourd (BG) as a SARM and its effects on the muscle decline induced by orchiectomy. In the cell-based androgen receptor (AR) transactivation assay, the BGP extract showed weak agonistic and antagonistic activities, resembling those of some SARMs. Male C57BL/6J mice were sham-operated (Sham group) or castrated (Cast groups) and fed a modified AIN-93G high sucrose diet supplemented without (Cast group) or with 5% lyophilized BG powder (Cast + BGP) or with testosterone propionate (7 mg TP per kg diet, Cast + TP) for 23 weeks. In contrast to the Cast + TP group, the BGP supplementation did not affect the serum testosterone concentration, and prostate and seminal vesicle mass. Both TP and BGP supplementation increased the weight of androgen responsive muscles, bulbocavernosus (BC) and levator ani (LA) (p < 0.05). The grip strength and the performance on a rotarod of the Cast + BGP group were comparable to those of the Cast + TP group (p > 0.05). The number of succinate dehydrogenase (SDH)-positive fibers of the Cast + BGP group was not significantly different from that of the Sham and Cast + TP groups (p > 0.05). The BGP supplementation up-regulated the Pgc1α, Ucp2 or Ucp3 gene expressions in skeletal muscles of castrated mice (p < 0.05). BGP showed some characteristics of the SARM and might improve skeletal muscle function through the up-regulation of mitochondrial biogenic genes and oxidative capacity, and ameliorated the castration-induced decline of skeletal muscle function in mice.
1. Introduction
Loss of skeletal muscle mass and strength, resulting from ageing or co-morbidity of various diseases, is often associated with disability and poor quality of life.1 Sarcopenia, a gradual loss of skeletal muscle mass and function with age, and cachexia, weight loss due to an underlying illness, are the two most common muscle wasting disorders and represent important syndromes impacting quality and quantity of life in the geriatric population.2 Besides exercise and nutrition, the management of these conditions also includes hormones/signaling molecules known to enhance muscle development.2 For example, testosterone, a primary androgenic hormone in men, exerts a broad range of male physiological functions, and has a potent anabolic effect on skeletal muscles.3 Experimental androgen deprivation using either castration or androgen receptor knockout (ARKO) animal models typically results in a rapid loss of muscle mass and decreased muscle strength.4,5 Interestingly, not all muscles respond to androgen deprivation in the same way in these animal studies. The bulbocavernosus (BC) and levator ani (LA), skeletal muscles that attach to the base of the penis, have been found to be highly responsive to androgen.6 In contrast, limb muscles, such as triceps brachii (TRI) and extensor digitorum longus (EDL), are relatively less responsive.
Epidemiologic studies have demonstrated a correlation among the bioavailable testosterone concentration, fat-free mass and muscle strength.7,8 Hormone replacement therapy with androgens improved muscle function in both castrated animals9 and hypogonadal individuals.10,11 However, the uncertainty of the side effects associated with testosterone replacement therapy has limited its wide applications.12 Currently, selective androgen receptor modulators (SARMs) are under development for the treatment of sarcopenia13 or cancer cachexia.14 SARMs form a novel class of androgen receptor ligands which provide anabolic benefits in the absence of androgenic effects on sexual organs.15 The development of SARMs is intended to offer anabolic effects in muscle and bone, and they might also revert other castration-related adverse effects such as those in adipose tissues. As SARMs have an optimal effect on myo-anabolic activity but less of growth effects on prostate and other secondary sexual organs than traditional androgen therapy, they are promising as a new class of function promoting anabolic therapy for multiple clinical indications.16,17
Androgen deficiency contributes to mitochondrial dysfunction and disruption of normal cellular function, including production of ATP and promoting cell death.18 Mitochondrial dysfunction adversely affected the quality and quantity of skeletal muscles and was closely related to the loss of skeletal muscle mass with ageing19 and cachexia.20 Peroxisome proliferator-activated receptor gamma coactivator 1-alpha (PGC1α) plays a crucial role in maintaining muscle metabolic function and controls numerous genes that affect muscle morphology and physiological functions.21 The expression levels of PGC1α in the gastrocnemius skeletal muscle is up-regulated by supplementation of supra-physiological testosterone and down-regulated by AR knockout.22 The roles of PGC1α in enhancing mitochondria biogenesis23 and suppressing gene expressions of forkhead transcription factors 3 (FoxO3), atrophy-specific atrogin-1 (Fbxo32), and Muscle RIN-finger protein-1 (MuRF-1 or Trim63) in skeletal muscles24 have made it another molecular target for developing therapeutics for muscle wasting.
Bitter gourd (Momordica charantia, BG) is a common tropical vegetable belonging to the Cucurbitaceae family. It has traditionally been used in herbal medicine. Scientific evidence for its biological effects,25 especially for metabolic disorders26 have been addressed. BG supplementation improved insulin sensitivity in the skeletal muscles.27,28 Our previous study demonstrated that BG supplementation significantly up-regulated the PGC1α mRNA level in the gastrocnemius muscle of mice.29 Recently, Hsiao et al. reported that 4-week supplementation of BG enhanced muscle strength and reduced fatigue in male ICR mice.30 Bioactive compounds isolated from BG can activate nuclear receptors, including peroxisome proliferator activated receptors (PPARs)31 and estrogen receptors (ERs).32 In this study, we examined the BG extract and index compounds for the transactivation of the androgen receptor (AR) in a cell-based assay. The orchidectomized mouse model was then used to evaluate the SARM-like characteristics of BG.
2. Materials and methods
2.1 Preparation of wild bitter gourd powder and experimental diets
The strain 2381 of wild bitter gourd bred and provided by Hualien District Agricultural Research and Extension Station was used. Whole fresh fruits (with seeds) were sliced, freeze dried and ground to produce the bitter gourd powder (BGP). The high sucrose (HS) diet was modified from the AIN-93G33 diets in which carbohydrate was provided by 50% sucrose and 12.95% cornstarch. The BGP diet was formulated by incorporating 5% (w/w) of BGP into the HS diet. Testosterone propionate (TP) (Sigma-Aldrich, St Louis, MO, USA) was incorporated into the HS diet (7 mg TP per kg diet, TP diet) as a positive control. The composition of experimental diets is listed in ESI Table 1.†
2.2 Animals
Six-week old male C57BL/6J mice were purchased from the National Laboratory Animal Centre (Taipei, Taiwan) and housed individually in steel wire cages in an animal room with controlled temperature and a light–dark cycle. Mice were acclimatized for 2 weeks and then randomly assigned to castration or sham surgery. After a 1-week surgical recovery period, they were further assigned to four experimental groups: sham-operated mice fed the HS diet (Sham, n = 7), castrated mice fed the HS diet (Cast, n = 8), castrated mice fed the BGP diet (Cast + BGP, n = 8) and castrated mice fed the TP diet (Cast + TP, n = 7). The animal study was approved by the Institutional Animal Care and Use Committee (IACUC) of National Taiwan University (No. NTU-105-EL-00009).
2.3 Castration and sham-operated surgery
Surgery was performed under sterile conditions. Mice were anesthetized by an i.p. injection of ketamine (100 mg kg−1) and xylazine (20 mg kg−1). Then, both testes were gently pushed from the abdomen into the scrotum and a 5 mm incision was made on the midline of the scrotum. Another smaller incision was made on the parietal tunica, and then one of the testes was carefully pulled out through the incision till the spermatic cord was exposed. The spermatic cord was clamped with a hemostat and ligated above, and the spermatic cord below the hemostat through the testis was dissected away. The remaining tissue was placed back into the scrotum. This procedure was repeated on the contralateral testis. The scrotal incision was closed with sutures. The sham-operated animals were anesthetized and incisions were made. Their testes were gently pulled out and then placed back into the scrotum, the incisions were then closed with sutures. The absence of testes in every castrated mouse was confirmed at necropsy after 23 weeks of feeding.
2.4 Serum testosterone level analysis
Blood samples were collected from the retro-orbital sinus under light anesthesia. The collected blood samples were centrifuged twice at 12
000g, 4 °C, for 20 min. Serum samples were isolated for measuring serum testosterone concentrations using the Testosterone ELISA kit (Cayman Chemical Company, Ann Arbor, Michigan, USA) following the manufacturer's instructions.
2.5 Muscle strength and performance tests
The grip strength and rotarod performance of the tested mice were examined during the experimental period. To minimize variability, each test was conducted in the evening of the same day and in the same environment by the same operator.
To measure the forelimb grip strength, a mouse was allowed to grab a metal wire grid with its forelimb and then pulled backwards in the horizontal plane with increasing force until it released the grid. The maximal force (in gram) was recorded using an automatic force transducer (Muromachi, Japan). The test consisted of two sessions with at least 30 seconds of rest between the two sessions. In each session, each mouse was tested five times with 5 second intervals. The mean maximal force was taken as the grip strength.
A 5-lane accelerating rotarod (TSE Systems, Bad Homburg, Germany) was used for measuring muscle endurance and motor abilities. Before the test, mice were trained at a relatively slow speed (12 rpm) 3 times with 3 min at each time and at least 10 min intervals on each day. The training was repeated for four consecutive days. On the test days, mice were placed on a static rod, and the motor was turned on and accelerated from 5 to 40 rpm over 5 min until all mice fell off the rod. Each mouse was tested six times with at least 30 min interval between tests. The mean duration times (in second) the mouse remained on the rod were recorded as the latency to fall.
2.6 Blood and tissue sample collection
After 23 weeks of feeding, mice were fasted overnight and killed by CO2 asphyxiation. Blood samples were withdrawn through cardiac puncture. Liver, seminal vesicle (SV)/prostate, testis, epididymis, adrenal gland and skeletal muscle tissue including gastrocnemius (GAS), soleus (SOL), tibialis anterior (TA), extensor digitorum longus (EDL), triceps brachii (TRI), levator ani (LA) and bulbocavernosus (BC) were excised, weighed and immediately frozen in liquid nitrogen and stored at −80 °C for mRNA analysis. For histology analysis, muscle tissues were fixed by overnight immersion in 30% sucrose/PBS at 4 °C. Muscle tissues were then embedded in an optimal cutting temperature compound (OCT, Sakura Finetek USA, Torrance, CA, USA) in dry-ice-cooled 2-methylbutane (Sigma) and stored at −80 °C until preparation of cryosections.
2.7 Histological analysis
Cryosections were cut from the mid-belly region of TA, GAS and TRI muscles on a cryostat (Leica 3050 S, Leica GmbH, Germany) at −23 °C to −25 °C. The sections were then placed on poly-L-lysine coated glass slides (Sigma) and stored at −20 °C until the analysis of succinate dehydrogenase (SDH) activity within a month. For SDH staining, slides were soaked in PBS at room temperature for 10 min and then dried for 5 min before incubation in an SDH working solution, which consisted of 0.24 M disodium succinate (Sigma), 1 mM 1-methoxyphenzine methosulphate (mPMS, Sigma), 1.5 mM nitroblue tetrazolium (NBT, Sigma) and azide/EDTA/PO4 buffer (including 0.02 M sodium azide, 0.125 M disodium EDTA, 0.3 M NaH2PO4 and 0.3 M Na2HPO4 in deionized water, pH = 7.4) at room temperature for 15 min. Slides were then washed in deionized water to stop the enzymatic reaction and air-dried for 10 min in the dark. Slides were mounted with mounting medium (Leica) and stored at −20 °C. For the evaluation of the muscle fiber cross-section area (CSA), hematoxylin and eosin (H&E) staining was performed on muscle cryosections. Briefly, the slides were fixed in 4% paraformaldehyde overnight and then H&E stained following the standard protocol.34 Digital photographs were taken from each section under a microscope (Leica DM1000LED), which was connected to a computerized imaging system. All images were analyzed by manually quantifying the SDH intensity and fiber size with the Image J software (NIH, U.S. National Institutes of Health, Bethesda, MD, USA). In SDH distribution and intensity analyses, all images were converted to a grayscale for histogram analysis with Image J. The intensities of the gray-scale images were expressed on a scale of 0–255 (0: black, 255: white). The number of myofibers with various intensities were quantified and expressed in a distribution graph.
2.8 RNA extraction and quantification of mRNA
Total RNA was isolated from various muscles by using the TRIZOL reagent (Invitrogen, Carlsbad, CA, USA) and an RNA miniprep kit (ZYMO Research, Irvine, CA, USA) following the manufacturer's instruction. Total RNA (2 μg) was reverse transcribed to cDNA in a mixture containing 10× RT (reverse transcription) buffer, 100 mM dNTP mixture buffer, 10× RT random primer and MultiScribe™ RTase (Invitrogen, Carlsbad, CA, USA). The polymerase chain reaction (PCR) was performed in a final volume of 25 μL containing 10 μL cDNA, 12.5 μL TaqMan® Gene Expression Master Mix, 1.25 μL probe/primer assay mix and water. PCR primers and probes were purchased from Applied Biosystems (Carlsbad, CA, USA; ESI Table 2†). The PCR and fluorogenic analysis was performed using a CFX connectTM real-time PCR Detection System (Bio-Rad Laboratories, Berkeley, CA, USA). Amplification conditions were 2 min at 50 °C, 10 min at 95 °C then 40 cycles of 15 s at 95 °C and 1 min at 60 °C. Gene expression was quantified using the relative (ΔΔCT) method with normalization to the levels of glyceraldehyde 3-phosphate dehydrogenase (Gapdh) mRNA expression.
2.9 Transactivation assay for AR activity
BGP was extracted with ethyl acetate (1
:
20, w/v) with stirring at room temperature overnight. The ethyl acetate extract (EAE) of BGP was obtained by filtration and evaporation to remove the solvent. The extraction rates of the EAE was 9.4%. This BGP extract was dissolved in a minimal amount of absolute ethanol and diluted with cell culture medium to concentrations that did not affect cell growth or viability.
Androgen receptor transactivation activity was examined using a cell-based transactivation assay. Briefly, plasmids including pcDNA3-AR, pRL-TK (renilla luciferase plasmid, normalization vector) and 3× ARE Δ56-c-fos GL3 (reporter gene vector), provided by Dr Chih-Pin Chuu (Institute of Cellular and System Medicine, National Health Research Institutes, Miaoli, Taiwan), were transiently co-transfected into CHO-K1 cells (American Type Culture Collection; Manassas, VA, USA) using Lipofectamine™ 2000 (Invitrogen). The transfected cells were treated with varying concentrations of the BGP extract, conjugated linoleic acid (cis-9, trans-11-CLA or trans-10, cis-12-CLA) or 9c,11t,13t-CLN (α-eleostearic acid) in the absence or presence of 5α-dihydrotestosterone (5α-DHT, androgen receptor agonist; Wako, Japan) for 24 h. Luciferase activity was then measured using a Dual-Glo® Luciferase kit (Promega, Madison, Wisconsin, USA). 9c,11t,13t-CLN is one of the active compounds in BG that can activate PPARs and can be converted to 9c,11t-CLA in rats and mice.35–37 The conjugated fatty acids were purchased from Cayman Chemical Co. (Ann Arbor, MI, USA). Samples were dissolved in absolute ethanol and diluted to concentrations that did not affect the cell viability. Folds of activation were calculated by taking the vehicle-treated cells as 1.0.
2.10 Statistical analysis
Data are presented as means ± SD. Data were checked for the adherence to normal distribution. Those that did not were transformed to log, reciprocal or root square values. The significance of differences was analyzed by one-way ANOVA. Where there was a significant effect (p < 0.05), a Duncan's post-hoc test was performed for comparison among groups. In some cases, a two-sample Student's t test was further performed to compare between the supplemented Cast group and the Cast group. The Pearson correlation analysis was used to examine the association between muscle mass and gene expression levels. All analyses were conducted using the SPSS, version 21.0 (SPSS, Chicago, IL). P value < 0.05 was considered significant.
3 Results
3.1 The ethyl acetate extract of BGP weakly modulates the AR activation activity in the cell-based transactivation assay
In the cell-based AR transactivation assay, the dose response was tested in the concentration ranges that did not show cytotoxicity, 25–100 μg mL−1 for the BGP extract, 1–100 μM for 9c,11t-CLA, 1–50 μM for 9c,11t,13t-CLN, and 0.1–50 μM for 10t,12c-CLA. The BGP extract (50 μg mL−1) increased 5.3% of the AR transactivation but suppressed 26.2% and 30.9% of the AR activation when co-treated with 0.1 nM or 10 nM 5α-DHT, respectively (p < 0.05, Fig. 1A). Similarly, 9c,11t-CLA (10 μM) and 10t,12c-CLA (1 μM) increase 27% and 34% of AR transactivation, respectively (p < 0.05, Fig. 1B). 9c,11t,13t-CLN (50 μM) and 10t,12c-CLA (50 μM) suppressed 36% and 46% of the AR activation when co-treated with 10 nM 5α-DHT, respectively (p < 0.05, Fig. 1B).
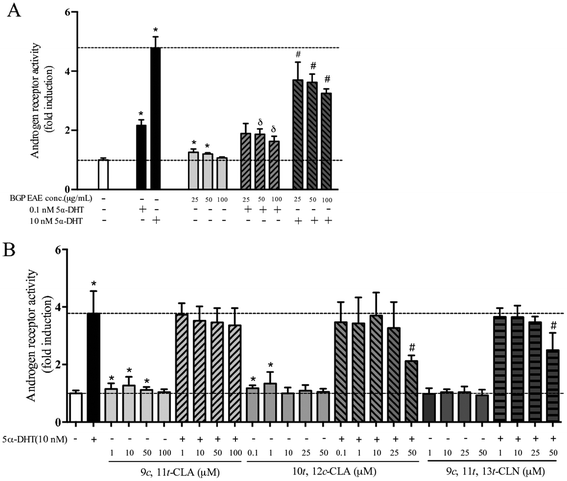 |
| Fig. 1 Transactivation assay of an AR using a BGP ethyl acetate extract (EAE) and conjugated fatty acids with or without 5α-DHT. CHO-K1 cells were co-transfected with pcDNA3-AR, 3× ARE Δ56-c-fos GL3 and pRL-TK (renilla luciferase control plasmid). Four hours after the transfection, cells were treated with 5α-DHT or various concentrations of test samples for 24 h and then harvested. Dual luciferase activities were measured and firefly values were normalized with Renilla values and then calculated as the fold of activation by taking the vehicle-treated cells as 1.0. Values are means ± SD of at least three separate experiments. 5α-Dihydrotestosterone (5α-DHT) is the positive control for the AR. Data were analyzed by Student's t test. * denotes significant difference from the vehicle (veh). δ and # denote significant difference from 0.1 nM DHT and 10 nM DHT, respectively. | |
3.2 Dietary BGP increased the mass of androgen responsive muscle but not SV/prostate in castrated mice
At the beginning of the feeding study, all mice had similar body weights (23.5 ± 0.6 g). After 23 weeks of feeding, the Cast + BGP group had significantly lower final body weight compared to the Sham and Cast groups (p < 0.05). At necropsy, the absolute weights of androgen responsive tissues/organs including epididymis, SV/prostate, and LA and BC muscles of the Cast group were significantly lower than those of the Sham group (p < 0.05) (Table 1). Compared to the Cast group, the Cast + TP group had a significantly higher mass of SV/prostate as well as LA, BC and quadriceps (p < 0.05). In the Cast + BGP group, weights of LA and BC were also higher than those of the Cast group (p < 0.05). However, the mass of the SV/prostate was comparable to that of the Cast group (p > 0.05). Similar results were observed when these tissue weights were calculated as % of the body weight except that the relative weight of quadriceps and GAS of the Cast + BGP group was comparable to those of the Cast + TP group (p > 0.05) (Table 1). Thus, the castration-induced mass reduction of the highly androgen responsive muscles38 was restored (LA) or partially restored (BC) by both TP and BGP treatment, but BGP did not affect the weights of prostate and seminal vesicles, in contrast to the TP treatment.
Table 1 The final body weight and organ/tissue weight of sham or castrated mice fed test diets for 23 weeks
Group |
Sham |
Cast |
Cast + BGP |
Cast + TP |
Final body weight (g) |
37.9 ± 3.6a |
34.7 ± 2.4b |
30.7 ± 2.1c |
32.3 ± 3.6bc |
|
Absolute organ/tissue weight (g) |
Sham |
Cast |
Cast + BGP |
Cast + TP |
Organ/tissue weight
|
Liver |
1.532 ± 0.241a |
1.484 ± 0.114ab |
1.284 ± 0.092c |
1.324 ± 0.137bc |
Testis |
0.196 ± 0.010b |
N/A |
N/A |
N/A |
Epididymis |
0.123 ± 0.022a |
0.009 ± 0.025b |
0.003 ± 0.008b |
0.004 ± 0.007b |
SVa/prostate |
0.519 ± 0.039a |
0.004 ± 0.004c |
0.005 ± 0.006c |
0.133 ± 0.021b |
Adrenal gland |
0.006 ± 0.002b |
0.009 ± 0.002a |
0.008 ± 0.002a |
0.008 ± 0.002ab |
Skeletal muscles
|
LAa |
0.078 ± 0.017a |
0.038 ± 0.014b |
0.065 ± 0.013a |
0.071 ± 0.028a |
BCa |
0.098 ± 0.007a |
0.015 ± 0.004d |
0.024 ± 0.009c |
0.042 ± 0.009b |
Triceps brachii |
0.253 ± 0.014a |
0.217 ± 0.030ab |
0.209 ± 0.013b |
0.226 ± 0.023ab |
Quadriceps |
0.411 ± 0.033a |
0.377 ± 0.021b |
0.400 ± 0.024ab |
0.427 ± 0.035a |
Gastrocnemius |
0.345 ± 0.021a |
0.328 ± 0.017a |
0.303 ± 0.011b |
0.333 ± 0.022a |
Soleus |
0.021 ± 0.003 |
0.024 ± 0.003 |
0.024 ± 0.003 |
0.023 ± 0.004 |
Tibialis anterior |
0.098 ± 0.017 |
0.095 ± 0.020 |
0.095 ± 0.013 |
0.098 ± 0.014 |
|
Relative organ/tissue weight (% body weight) |
Sham |
Cast |
Cast + BGP |
Cast + TP |
SV: seminal vesicle, LA: levator ani muscle, BC: bulbocavernosus muscle, EDL: extensor digitorum longus. Mice were sham-operated or orchidectomized and fed test diets for 23 weeks. The relative organ weight is expressed as the percentage of the body weight. The values represent mean ± SD. Data were analyzed by one-way ANOVA and Duncan's multiple comparison test. Values not sharing the same superscripted letter are significantly different at p < 0.05. |
Organ/tissue
|
Liver |
4.022 ± 0.284 |
4.285 ± 0.367 |
4.197 ± 0.272 |
4.113 ± 0.347 |
Testis |
0.522 ± 0.064 |
N/A |
N/A |
N/A |
Epididymis |
0.327 ± 0.072a |
0.023 ± 0.066b |
0.009 ± 0.024b |
0.011 ± 0.020b |
SVa/prostate |
1.373 ± 0.096a |
0.010 ± 0.012b |
0.017 ± 0.021b |
0.409 ± 0.042ab |
Adrenal gland |
0.016 ± 0.006b |
0.026 ± 0.006a |
0.027 ± 0.007a |
0.025 ± 0.005a |
Skeletal muscles
|
LAa |
0.208 ± 0.055a |
0.112 ± 0.046b |
0.214 ± 0.046a |
0.218 ± 0.070a |
BCa |
0.262 ± 0.041a |
0.042 ± 0.011d |
0.078 ± 0.031c |
0.130 ± 0.021b |
Triceps brachii |
0.673 ± 0.078 |
0.626 ± 0.084 |
0.684 ± 0.067 |
0.709 ± 0.123 |
Quadriceps |
1.091 ± 0.127b |
1.094 ± 0.126b |
1.310 ± 0.096a |
1.330 ± 0.110a |
Gastrocnemius |
0.914 ± 0.049c |
0.947 ± 0.045bc |
0.990 ± 0.042ab |
1.038 ± 0.088a |
Soleus |
0.057 ± 0.008b |
0.069 ± 0.008a |
0.079 ± 0.011a |
0.072 ± 0.009a |
Tibialis anterior |
0.259 ± 0.050 |
0.277 ± 0.067 |
0.312 ± 0.047 |
0.307 ± 0.058 |
EDLa |
0.056 ± 0.011 |
0.065 ± 0.010 |
0.068 ± 0.004 |
0.68 0.017 |
3.3 BGP did not affect the testosterone concentration but ameliorated the castration-induced decline of muscle strength and performance in mice
As shown in Fig. 2, all 4 groups have similar serum testosterone levels before surgery. After castration, serum testosterone declined rapidly as reported.39 BGP supplementation did not affect the serum testosterone level in castrated mice. In contrast, the Cast + TP group had significantly higher serum testosterone levels compared to the Cast and Cast + BGP groups (p < 0.05). However, the levels of serum testosterone of the Cast + TP group was still lower than that of the Sham group (p < 0.05) (Fig. 2A).
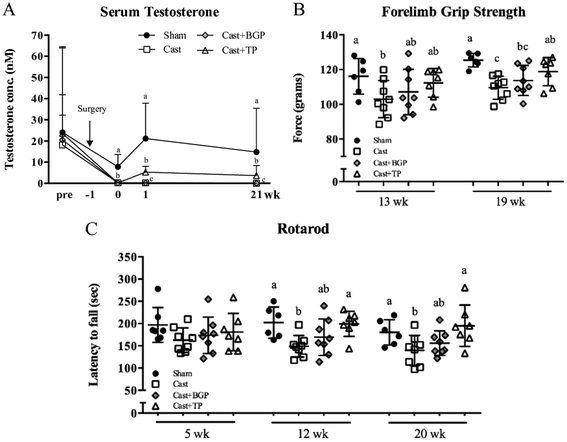 |
| Fig. 2 Serum testosterone concentration and muscle function tests of sham or castrated mice supplemented with BGP or TP. (A) Serum testosterone concentration; (B) average forelimb grip strength in grams; (C) latency to fall in seconds in the accelerating rotarod test (5–40 rpm/5 min). In (B) and (C), each data point is the mean value of each mouse and the group means and SD are also shown. Values are means ± SD. Data were analyzed by one-way ANOVA and Duncan's multiple range test. Data of serum testosterone levels were transformed to log values to conform to normality before statistical analysis. Values not sharing the same superscript letters are significantly different at p < 0.05. | |
Muscle strength and motor coordination performance were measured during the experimental period. After feeding test diets for 19 weeks, the forelimb grip strength showed an 11% reduction in the Cast group compared to that of the Sham group (p < 0.05), while TP treatment restored it (Fig. 2B). In the Cast + BGP group, the grip strength was comparable to that of the Cast + TP group (p > 0.05) (Fig. 2B). After feeding test diets for 12 weeks, the Sham and Cast + TP groups showed significantly longer duration times on the rotarod than the Cast group (p < 0.05) (Fig. 2C). At 20 weeks, the duration time on the rotarod showed a 19% reduction in the Cast group compared to that in the Sham group, while TP supplementation restored it to the level of the Sham group. The performance of the Cast + BGP group on the rotarod was also comparable to that of the Cast + TP group (p > 0.05).
3.4 BGP increased the expression of Pgc1α and improved oxidative capacity in skeletal muscles
The mRNA expression levels of Pgc1α and its target genes in some muscles are shown in Fig. 3. Remarkably, the Cast group showed significantly lower mRNA expression levels of most genes related to mitochondrial biogenesis, morphology and oxidative phosphorylation (OXPHOS) in the androgen responsive BC muscle. Similarly, in GAS (consisting of >50% fast-twitch muscle fibers) and SOL (fast-twitch) muscles, the mRNA expressions of Pgc1α and some of its target genes associated with mitochondrial function were also lower in the Cast group compared to those of the Sham group (Fig. 3C and D). Interestingly, the Cast + BGP group of mice had up-regulated mRNA of Pgc1α and its target genes in the BC muscle (p < 0.05). In addition, the mRNA expressions of Pgc1α, Ucp2 and Ucp3 were also higher in the LA muscle of the Cast + BGP group (p < 0.05). Furthermore, the expression of Pgc1α, Ucp2 or Ucp3 in the hindlimb muscles of the BGP group was also higher than those of the Cast group (p < 0.05).
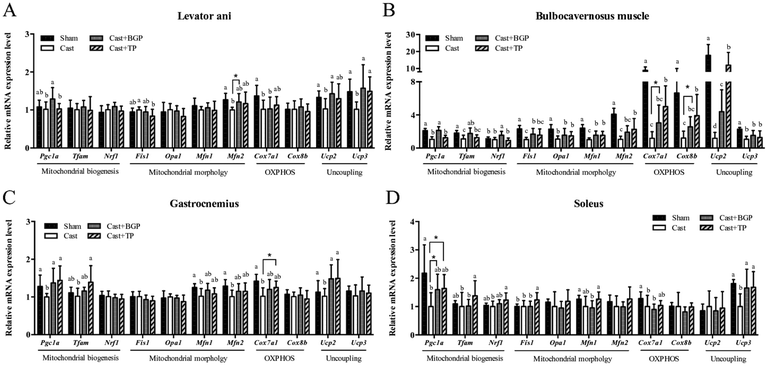 |
| Fig. 3 The mRNA expression levels of genes related to mitochondrial functions in various muscles of sham or castrated mice supplemented with BGP or TP. The mRNA of genes related to mitochondrial biogenesis, morphology, oxidative phosphorylation and uncoupling in highly androgen-responsive levator ani (A) and bulbocavernosus muscles (B) or the hind limb skeletal muscles gastrocnemius (C) and soleus (D) were analyzed. Values are means ± SD. Data are transformed into log or reciprocal if they are not normally distributed before the statistical analysis. The significance of differences were analyzed by one-way ANOVA and Duncan's multiple range test. Values not sharing the same superscript letters are significantly different at p < 0.05. In some cases, a two-sample Student's t test was further performed for the comparison with the Cast group. * denotes significant difference from the Cast group (p < 0.05). | |
We next examined the distribution and intensity of SDH, a marker of oxidative capacity, using SDH staining, in TA, GAS and TRI muscle sections. As shown in Fig. 4, the muscle fibers in the Sham group stained more darkly than those of the Cast group. Compared to the Sham group, the number of SDH-positive fibers of the Cast group decreased 31% (p < 0.05) and 12% in TA and GAS muscle, respectively. On the other hand, the number of SDH-positive fibers in the TA and GAS of the Cast + BGP group was comparable to those of the Sham and Cast + TP groups (p > 0.05) (Fig. 4). In contrast, there was no difference in percent SDH-positive fibers in TRI muscle among groups, which is relatively less responsive to androgen deprivation.
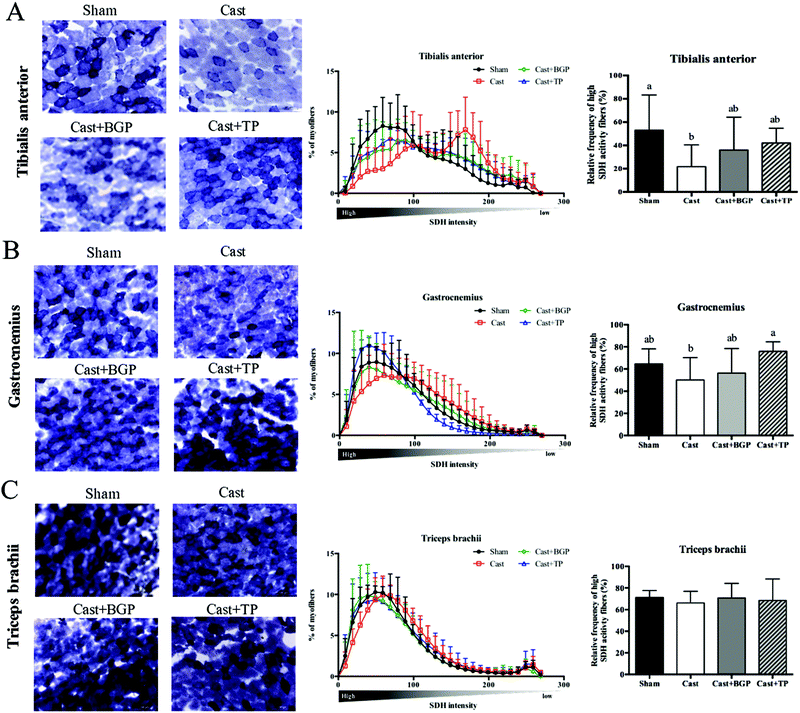 |
| Fig. 4 Histochemical staining of succinate dehydrogenase activity in various limb muscles of sham or castrated mice supplemented with BGP or TP. SDH activity was observed from stained frozen sections of tibialis anterior (A), gastrocnemius (B) and triceps brachii (C) muscles. The staining intensity was analyzed with Image J and plotted as intensity distribution diagrams and high SDH activity fibers were quantified in TA, GAS and TRI muscles of each group. Values in the bar graph are means ± SD. Data were analyzed by one-way ANOVA and Duncan's multiple range test. Values not sharing the same letter are significantly different at p < 0.05. Scale bar: 100 μm. | |
3.5 BGP did not change the fiber size distribution and most of the myogenesis-related gene expression levels in castrated mice
As shown in Fig. 5, the mRNA expressions of myogenic factor 6 (Myf6), insulin-like growth factor-1 (Igf1), eukaryotic translation initiation factor 3, subunit F (elF3f) and myostatin (Mstn) in the BC muscle of the Cast group were lower than those of the Sham group (p < 0.05). The BGP + Cast group had significantly higher mRNA expressions of Mf6 and Igf1 in the BC muscle than the Cast group (p < 0.05).
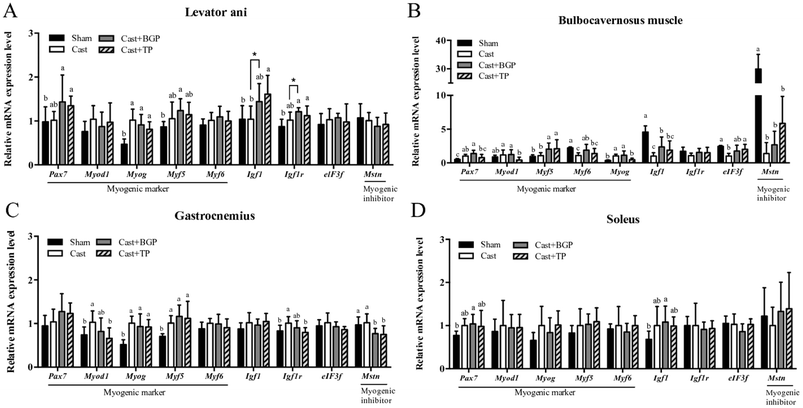 |
| Fig. 5 The mRNA expressions of genes related to myogenesis in various muscles of sham or castrated mice supplemented with BGP or TP. The mRNA expression levels of genes related to myogenesis in the highly androgen-responsive levator ani (A) and bulbocavernosus muscles (B) or the hind limb skeletal muscles gastrocnemius (C) and soleus (D) were analyzed. Values are means ± SD. Data were transformed into log or reciprocal if they are not normally distributed before the statistical analysis. The significance of differences were analyzed by one-way ANOVA and Duncan's multiple range test. Values not sharing the same superscript letters are significantly different at p < 0.05. In some cases, a two-sample Student's t test was further performed for a comparison with the Cast group. * denotes significant difference from the Cast group (p < 0.05). | |
Since it is believed that, at the single fiber level, the maximal force is directly related to its CSA, we also examined the distributions of the myofiber CSA of TA, GAS and TRI muscles. As shown in Fig. 6, the castrated mice tended to have a smaller CSA and their mean CSA was significantly lower than that of the Sham group (p < 0.05). However, the size distribution and mean CSA of Cast + BGP and Cast + TP groups were comparable to those of the Cast group. Supplementation did not significantly change the CSA distribution in castrated mice (Fig. 6). The size distribution of these muscle fibers in castrated mice tended to shift toward the left when compared to the Sham group, and the BGP and TP supplementation did not restore the distribution of the fiber size to the control levels.
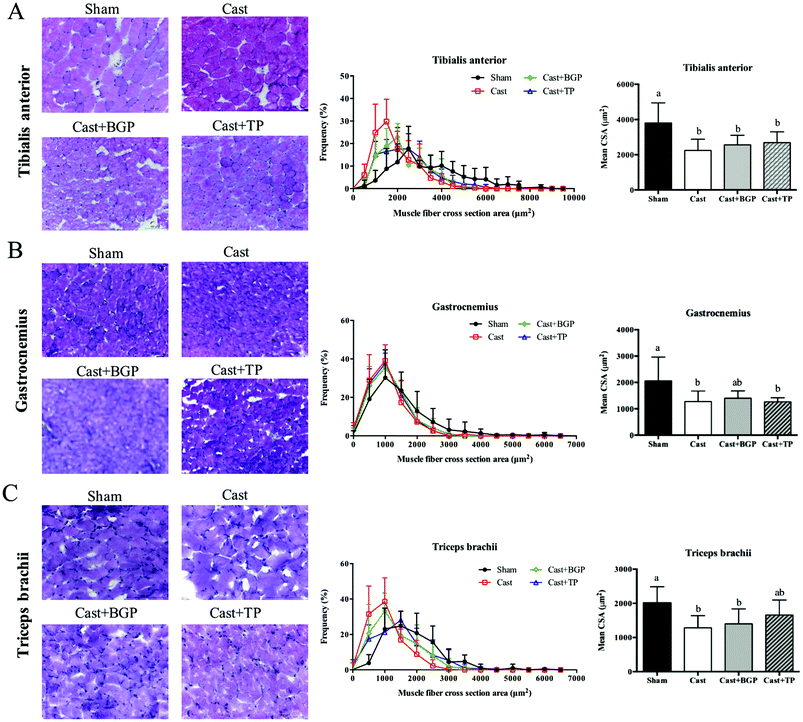 |
| Fig. 6 The cross-section area (CSA) of skeletal muscle fibers in various limb muscles of sham or castrated mice supplemented with BGP or TP sections from dry ice-cooled isopentane frozen tibialis anterior (A), gastrocnemius (B) and triceps brachii (C) muscles were fixed in 4% paraformaldehyde in PBS and stained with hematoxylin and eosin. Histogram distribution of the myofiber cross-section area (CSA) (A, B and C) and the average CSA (D) was analyzed with Image J. Values are means ± SD. Data were analyzed by one-way ANOVA and Duncan's multiple range test. Values not sharing the same letter are significantly different at p < 0.05. Scale bar: 100 μm. | |
3.6 The mRNA expression of Igf1, Ucp2 and Pgc1α positively correlated with the relative weight of androgen responsive muscles
The correlations between the muscle mass and mRNA expressions of several genes in the highly androgen responsive LA and BC muscles are shown in Table 2. Interestingly, the expressions of Igf1, Upc2 and Pgc1α mRNA levels were positively and significantly correlated with the mass in both muscles (p < 0.05). In addition, the mRNA expressions of Ucp3, cytochrome c oxidase subunit VIIa 1 (Cox7a1) and cytochrome c oxidase subunit VIIIb (Cox8b) also positively correlated with the relative weight of the BC muscle. Remarkably, the mRNA expressions of most genes listed are positively and significantly correlated with each other in the BC muscle, implying an association in the expressions of these genes.
Table 2 The correlation coefficients (r) among the relative weight of LA and BC muscles and the level of mRNA expression
|
Relative weight (% of BW) |
Ucp2
|
Ucp3
|
Pgc1a
|
Cox7a1
|
Cox8b
|
Igf1
|
Igf1r
|
Data were analyzed by Pearson's correlation. * denotes significant correlation, p < 0.05. ** denotes significant correlation, p < 0.01. LA: levator ani muscle, BC: bulbocavernosus muscle. |
LA muscle
|
Relative weight (% of BW) |
1 |
|
|
|
|
|
|
|
Ucp2
|
0.437* |
1 |
|
|
|
|
|
|
Ucp3
|
0.189 |
0.327 |
1 |
|
|
|
|
|
Pgc1a
|
0.378* |
0.454* |
0.309 |
1 |
|
|
|
|
Cox7a1
|
−0.267 |
0.120 |
0.477** |
0.061 |
1 |
|
|
|
Cox8b
|
−0.434* |
−0.152 |
0.324 |
0.243 |
0.574** |
1 |
|
|
Igf1
|
0.516** |
0.095 |
−0.079 |
0.304 |
−0.320 |
−0.26 |
1 |
|
Igf1r
|
−0.110 |
0.126 |
0.309 |
0.407* |
0.129 |
0.444* |
0.182 |
1 |
|
BC muscle
|
Relative weight (% of BW) |
1 |
|
|
|
|
|
|
|
Ucp2
|
0.889** |
1 |
|
|
|
|
|
|
Ucp3
|
0.608** |
0.597** |
1 |
|
|
|
|
|
Pgc1a
|
0.494* |
0.419* |
0.730** |
1 |
|
|
|
|
Cox7a1
|
0.836** |
0.773** |
0.663** |
0.451* |
1 |
|
|
|
Cox8b
|
0.689** |
0.668** |
0.620** |
0.441* |
0.883** |
1 |
|
|
Igf1
|
0.818** |
0.688** |
0.617** |
0.595** |
0.826** |
0.770** |
1 |
|
Igf1r
|
0.345 |
0.528** |
0.683** |
0.561** |
0.356 |
0.355 |
0.317 |
1 |
4 Discussion
The in vivo efficacy of a SARM is usually tested by the Hershberger assay, in which the weights of LA muscle, and prostate and seminal vesicles of treated castrated rats are compared to those of castrated and sham-operated rats.16 Using a similar approach in a mouse model, this study provides evidence that BGP shows SARM-like properties. In addition, our cell-based AR transactivation assay further demonstrated that a BGP EA extract weakly modulated AR activation activities similar to those of some SARMs in development.40,41
In our animal study, data of the serum testosterone concentration, weights of androgen responsive tissues, including prostate/seminal vesicles, and LA and BC muscles, as well as grip strength and rotarod performance tests confirmed the androgen deficient status of the Cast mice. Supplementation of TP restored these parameters in a non-selective manner. In contrast, supplementation of BGP to castrated mice did not affect the serum testosterone and seminal vesicle/prostate weights, but improved the androgen responsive LA and BC muscle weight as well as the grip strength and rotarod performance, suggesting a tissue selective androgen receptor modulation activity.
Although the SV/prostate and BC muscle weights of the Cast + TP group were significantly higher than those of the Cast group, these values were still significantly lower than those of the Sham group (Table 1). These differences between the Sham and Cast + TP groups coincide with the difference in the serum testosterone concentrations between these two groups (Fig. 2A). These results suggest that the androgen status of the Cast + TP group in this study was lower than the normal physiological condition. Interestingly, the weight of the LA muscle of the Cast + TP group was comparable to that of the Sham group, suggesting that a lower androgen threshold is required to support this muscle.
Except for LA and BC, the remaining skeletal muscles weighed in this study hardly corresponded to the androgen status, agreeing with previous reports.6,38 However, our results of the SDH staining (Fig. 4) showed that the oxidative capacity of TA and GAS muscles was affected by the androgen status. Results of the two functional tests, grip strength and rotarod performance, corresponded to the androgen status as well.
The CSA is a commonly used indicator of muscle fiber hypertrophy. The anabolic effects of testosterone have been shown to increase the muscle weight and CSA in mice42 or humans.43 In this study, castrated mice showed a greater number of small size fibers in TA, GAS and TRI muscles as expected. However, the BGP and TP treatment did not change the mean CSA in castrated mice. Previous studies also showed that the anabolic effect of testosterone on muscle is dose dependent.44 Serum testosterone levels equal to or higher than those of a young adult are required to show the increased volume of some muscles.44 In addition, fiber-hypertrophy in mice was shown to be induced by supra-physiological testosterone supplementation for 8 weeks.45 The TP and BGP treatment in this study did not change the mean CSA in the castrated mice which might be related to the lower-than normal physiological androgen status.
Among the 4 muscles measured, the mRNA expressions of genes related to mitochondria of the BC muscle corresponded most pronouncedly to the androgen status, especially those of oxidative phosphorylation and Ucp2 (Fig. 2). These results agree with the data of BC weight which was supported by the positive and significant correlations of BC weight and mRNA expressions of Ucp2, Ucp3, Pgc1α, Cox7a, and Cox8b (Table 2), again, suggesting that this androgen responsive muscle tissue might be more sensitive to the androgen status. In this regard, BGP supplementation significantly elevated the BC weight of castrated mice, but the BC weight of the Cast + BGP group was still significantly lower than that of the Cast + TP group. It is thus speculated that the androgen status of the Cast + BGP group might be even lower than that of the Cast + TP group. The weak agonistic activity of the BG extract in the AR transactivation assay also supports this speculation. This may account for the insignificant differences between the performance in the functional tests and SDH staining of TA and GAS muscles between the Cast + BGP group and the Cast group. Nevertheless, there were also no significant differences of these parameters between the Cast + BGP and Cast + TP groups, suggesting that BGP showed a trend of effects similar to TP.
Testosterone up-regulates Igf1 gene expression through the androgen response elements (AREs) within the upstream promoter region of the IGF1 gene.46 Interestingly, the Igf1 mRNA expression level in the BC muscle corresponded to the androgen status in this study (Fig. 5B) and was positively correlated with the BC weight (Table 2). The BGP supplementation also significantly up-regulated Igf1 mRNA expression to a level comparable to TP supplementation. Similarly, the mRNA of Ucp3 expression in the LA, BC and SOL muscles, and Ucp2 in the LA, BC and GAS muscles, corresponded to the androgen status as well. The BGP supplementation also up-regulated Ucp3 and/or Ucp2 expressions in these muscles, to levels comparable to those of the Sham and Cast + TP groups, except Ucp2 in the BC which was significantly lower than that of the Cast + TP group.
PGC1α is well recognized for its role in mitochondrial biogenesis and respiration through an induction of nuclear respiratory factor NRF1, NRF2 and UCP2 gene expressions47,48 and has been demonstrated to spare muscle mass and improve endurance in atrophic muscles.49 Skeletal muscles PGC1α and PPARβ/δ have been proposed to be key regulators of exercise performance. Overexpression of PGC1α specifically in skeletal muscles increased the muscle oxidative capacity and the proportions of type I and IIa fibers in transgenic mice.50,51 In contrast, the muscle-specific knock-out of the Pgc1α gene in mice exhibited a reduction in the exercise performance and muscle respiratory capacity compared to those of wild type control mice.52 In addition, testosterone replacement up-regulated Pgc1a and Ucp3 expression in the TRI muscle of castrated mice.53 In this study, castration lowered the mRNA expressions of Pgc1α in BC, GAS and SOL muscles. The BGP supplementation increased Pgc1α mRNA in all these four muscles. In contrast, TP supplementation only restored these to some extent in GAS and SOL, but not in BC muscles. The up-regulation of Pgc1α mRNA in muscles of the Cast + BGP group might thus be, at least in part, from mechanisms other than the androgen receptor.
Emerging evidence suggests that UCP2 plays a positive physiological role by regulating mitochondrial biogenesis, substrate utilization and ROS elimination,54 thereby providing possibly anti-ageing effects.55 We also observed up-regulations of the Ucp2 mRNA in LA, BC and GAS muscles of the BGP and TP treated castrated mice. Overexpression of Pgc1α in C2C12 myotubes has been shown to up-regulate UCP2 expression.48 But the pattern of UCP2 expression in response to various treatments in this study were not completely parallel to that of the Pgc1α expression in all 4 muscles tested, suggesting that other mechanisms might be involved. Computational BLAST was thus conducted to preliminarily search for possible AREs in the promoter region of the mouse UCP2 gene (ESI Table 3†). Further studies are required to explore whether these putative ARE sites are functional.
The fiber size and oxidative capacity of muscles are known to be determined from the balance among myofibrillar protein synthesis, mitochondrial biosynthesis and mitochondrial degradation.56 The inverse relationship between the striated muscle fiber size and its oxidative capacity, the so-called “fiber type-fiber size paradox”, is interpreted in part from the competition between signaling pathways for the synthesis of myofibrillar proteins and proteins associated with oxidative metabolism, such as increased mitochondrial biogenesis via AMP-activated protein kinase (AMPK).56 AMPK controls several processes via the transcriptional co-activator PGC1α and promotes the slow, oxidative phenotype in skeletal muscles.57 Previous studies have clarified another role of AMPK in inhibiting protein synthesis and opposing actions of mTOR and AMPK on muscle homeostasis.58 For example, AMPK activation suppresses the translation of myofibrillar proteins by inhibiting the mTOR-p70S6K pathway via activation of TSC-2 and by phosphorylation and inactivation of eEF-2. This interaction indicates that AMPK, in addition to being a stimulus of biosynthesis of mitochondria, is also an inhibitor of protein synthesis.59,60 These findings showed that mTOR and AMPK are cross-balancing pathways under physiologic conditions. In this regard, some cucurbitane-type triterpenoids isolated from BG were shown to activate AMPK in adipocytes and myocytes.61,62 Furthermore, AMPK can increase gene expressions of mitochondria biogenesis through directly phosphorylating the PGC1α protein. The phosphorylated PGC1α protein is required for the PGC1α dependent induction of the PGC1α promoter.63 We previously showed that Pgc1α mRNA is up-regulated in muscles of mice fed the BGP diet.29 It is therefore speculated that the BGP supplementation might improve the oxidative capacity of muscle fibers through the activation of the AMPK pathway, but these fibers remained in relatively small sizes.
We have identified various biologically active compounds from the BG. Those that can activate PPARα and γ include 9c,11t,13t-CLN,64 some cucurbitane-type triterpenoids,29 phytol and lutein.65 Some of the cucurbitane-type triterpenoids have also been shown to activate estrogen receptors32 and stimulate glucagon-like peptide 1 secretion in an enteroendocrine cell line.66 Some cucurbitane-type triterpenoids have been shown to activate AMPK.61,62 Therefore, multiple compounds in this common tropical vegetable may act on various molecular targets and lead to beneficial health effects. In this study, we tested 9c,11t,13t-CLN and its metabolite in rodents, 9c,11t-CLA35–37 and its isomer 10t-12c CLA,67 along with the BGP extract, in the AR transactivation assay. The weak agonistic and antagonistic activity of the BGP extract can also be observed in these conjugated fatty acids. These data imply that 9c,11t,13t-CLN and its metabolite 9c,11t-CLA may be one of the bioactive compounds in BGP. The possibility of the contribution of other active compounds cannot be excluded.
Bitter gourd is a common vegetable in the tropical area. In our previous study, ∼5 g day−1 freeze-dried bitter gourd powder was supplemented to patients with the metabolic syndrome for 3 months in a preliminary clinical trial. This level is equivalent to 1% (wt/wt) BGP in the diet for mice study, as the common Taiwanese diet has been estimated to be 450–500 g d−1 in the dry weight basis. Results of this trial demonstrated not only the safety of the supplementation, but also the activity of BG in ameliorating the metabolic syndrome.68 On the other hand, ∼5 g of BGP is equivalent to ∼100 g of fresh bitter gourd which is a serving of vegetable. Dietary guidelines recommended at least 3 servings of vegetable per day.
In conclusion, this study demonstrated that BGP supplementation may ameliorate castration-induced decline of grip strength, exercise performance, and muscle mass and up-regulate Pgc1α, Ucp2 and mitochondrial biogenic genes in some muscles, but did not increase the mass of the prostate in orchidectomized mice. In vitro, the BGP extract weakly modulated the AR transactivation activity.
Abbreviations
5α-DHT | 5α-Dihydrotestosterone |
AR | Androgen receptor |
BC | Bulbocavernosus |
BGP | Bitter gourd powder |
Cast group | Castrated mice fed a high sucrose diet |
Cast + BGP group | Castrated mice fed a high sucrose diet supplemented with 5% lyophilized BGP |
Cast + TP group | Castrated mice fed a high sucrose diet supplemented with TP |
CSA | Cross-section area |
GAS | Gastrocnemius |
LA | Levator ani |
PGC1α | Peroxisome proliferator-activated receptor gamma coactivator 1 alpha |
SARM | Selective androgen receptor modulator |
SDH | Succinate dehydrogenase |
Sham group | Sham-operated mice fed the high sucrose diet in which carbohydrate was provided by 50% sucrose and 12.95% cornstarch |
SOL | Soleus |
SV | Seminal vesicle |
TA | Tibialis anterior |
TP | Testosterone propionate |
TRI | Triceps brachii |
UCP2/3 | Uncoupling protein 2/3 |
Conflicts of interest
There are no conflicts of interest to declare.
Acknowledgements
This study is supported by a grant 105-2320-B-002-029-MY3 from the Ministry of Science and Technology, Taiwan.
References
- S. Cohen, J. A. Nathan and A. L. Goldberg, Muscle wasting in disease: molecular mechanisms and promising therapies, Nat. Rev. Drug Discovery, 2015, 14, 58–74 CrossRef CAS PubMed.
- S. Ali and J. M. Garcia, Sarcopenia, cachexia and aging: diagnosis, mechanisms and therapeutic options - a mini-review, Gerontology, 2014, 60, 294–305 CrossRef CAS PubMed.
- V. Dubois, M. Laurent, S. Boonen, D. Vanderschueren and F. Claessens, Androgens and skeletal muscle: cellular and molecular action mechanisms underlying the anabolic actions, Cell. Mol. Life Sci., 2012, 69, 1651–1667 CrossRef CAS PubMed.
- C. de Rooy, M. Grossmann, J. D. Zajac and A. S. Cheung, Targeting muscle signaling pathways to minimize adverse effects of androgen deprivation, Endocr.-Relat. Cancer, 2016, 23, R15–R26 CAS.
- Q. Jiao, A. M. Pruznak, D. Huber, T. C. Vary and C. H. Lang, Castration differentially alters basal and leucine-stimulated tissue protein synthesis in skeletal muscle and adipose tissue, American Journal of Physiology: Endocrinology and Metabolism, 2009, 297, E1222–E1232 CAS.
- J. A. Johansen, S. M. Breedlove and C. L. Jordan, Androgen receptor expression in the levator ani muscle of male mice, J. Neuroendocrinol., 2007, 19, 823–826 CrossRef CAS PubMed.
- F. Sattler, S. Bhasin, J. He, C. P. Chou, C. Castaneda-Sceppa, K. Yarasheski, E. Binder, E. T. Schroeder, M. Kawakubo, A. Zhang, R. Roubenoff and S. Azen, Testosterone threshold levels and lean tissue mass targets needed to enhance skeletal muscle strength and function: the HORMA trial, J. Gerontol., Ser. A, 2011, 66, 122–129 CrossRef PubMed.
- A. Renoud, R. Ecochard, F. Marchand, R. Chapurlat and P. Szulc, Predictive parameters of accelerated muscle loss in men-MINOS study, Am. J. Med., 2014, 127, 554–561 CrossRef PubMed.
- C. Serra, N. L. Sandor, H. Jang, D. Lee, G. Toraldo, T. Guarneri, S. Wong, A. Zhang, W. Guo, R. Jasuja and S. Bhasin, The effects of testosterone deprivation and supplementation on proteasomal and autophagy activity in the skeletal muscle of the male mouse: differential effects on high-androgen responder and low-androgen responder muscle groups, Endocrinology, 2013, 154, 4594–4606 CrossRef CAS PubMed.
- R. A. Atkinson, U. Srinivas-Shankar, S. A. Roberts, M. J. Connolly, J. E. Adams, J. A. Oldham, F. C. W. Wu, O. R. Seynnes, C. E. H. Stewart, C. N. Maganaris and M. V. Narici, Effects of Testosterone on Skeletal Muscle Architecture in Intermediate-Frail and Frail Elderly Men, J. Gerontol., Ser. A, 2010, 65, 1215–1219 CrossRef PubMed.
- R. J. Urban, Y. H. Bodenburg, C. Gilkison, J. Foxworth, A. R. Coggan, R. R. Wolfe and A. Ferrando, Testosterone administration to elderly men increases skeletal muscle strength and protein synthesis, Am. J. Physiol., 1995, 269, E820–E826 CAS.
- E. C. Osterberg, A. M. Bernie and R. Ramasamy, Risks of testosterone replacement therapy in men, Indian J. Urol., 2014, 30, 2–7 CrossRef PubMed.
- A. McKee, J. E. Morley, A. M. Matsumoto and A. Vinik, Sarcopenia: an endocrine disorder?, Endocr. Pract., 2017, 23, 1140–1149 Search PubMed.
- M. Morimoto, K. Aikawa, T. Hara and M. Yamaoka, Prevention of body weight loss and sarcopenia by a novel selective androgen receptor modulator in cancer cachexia models, Oncol. Lett., 2017, 14, 8066–8071 Search PubMed.
- S. Bhasin and R. Jasuja, Selective androgen receptor modulators as function promoting therapies, Curr. Opin. Clin. Nutr. Metab. Care, 2009, 12, 232–240 CrossRef CAS PubMed.
- R. Narayanan, C. C. Coss and J. T. Dalton, Development of selective androgen receptor modulators (SARMs), Mol. Cell. Endocrinol., 2018, 465, 134–142 CrossRef CAS PubMed.
- M. A. Naafs, Selective Androgen Receptor Modulators (SARMs)- A Mini-Review, Open Acc. J. Reprod. Sex. Disord., 2018, 1 Search PubMed , OAJRSD.MS.ID.000103.
- A. M. Traish, B. Abdallah and G. Yu, Androgen deficiency and mitochondrial dysfunction: implications for fatigue, muscle dysfunction, insulin resistance, diabetes, and cardiovascular disease, Horm. Mol. Biol. Clin. Invest., 2011, 8, 431–444 CAS.
- D. Y. Seo, S. R. Lee, N. Kim, K. S. Ko, B. D. Rhee and J. Han, Age-related changes in skeletal muscle mitochondria: the role of exercise, Integr. Med. Res., 2016, 5, 182–186 CrossRef PubMed.
- B. N. VanderVeen, D. K. Fix and J. A. Carson, Disrupted Skeletal Muscle Mitochondrial Dynamics, Mitophagy, and Biogenesis during Cancer Cachexia: A Role for Inflammation, Oxid. Med. Cell. Longevity, 2017, 2017, 3292087 Search PubMed.
- C. Kang and L. L. Ji, Role of PGC-1α in muscle function and aging, J. Sport Health Sci., 2013, 2, 81–86 CrossRef.
- T. Usui, K. Kajita, T. Kajita, I. Mori, T. Hanamoto, T. Ikeda, H. Okada, K. Taguchi, Y. Kitada, H. Morita, T. Sasaki, T. Kitamura, T. Sato, I. Kojima and T. Ishizuka, Elevated mitochondrial biogenesis in skeletal muscle is associated with testosterone-induced body weight loss in male mice, FEBS Lett., 2014, 588, 1935–1941 CrossRef CAS PubMed.
- C. Kang and L. Li Ji, Role of PGC-1alpha signaling in skeletal muscle health and disease, Ann. N. Y. Acad. Sci., 2012, 1271, 110–117 CrossRef CAS PubMed.
- M. Sandri, J. Lin, C. Handschin, W. Yang, Z. P. Arany, S. H. Lecker, A. L. Goldberg and B. M. Spiegelman, PGC-1alpha protects skeletal muscle from atrophy by suppressing FoxO3 action and atrophy-specific gene transcription, Proc. Natl. Acad. Sci. U. S. A., 2006, 103, 16260–16265 CrossRef CAS PubMed.
- S. Wang, Z. Li, G. Yang, C. T. Ho and S. Li, Momordica charantia: a popular health-promoting vegetable with multifunctionality, Food Funct., 2017, 8, 1749–1762 RSC.
- M. A. Alam, R. Uddin, N. Subhan, M. M. Rahman, P. Jain and H. M. Reza, Beneficial role of bitter melon supplementation in obesity and related complications in metabolic syndrome, J. Lipids, 2015, 2015, 496169 Search PubMed.
- M. G. Sridhar, R. Vinayagamoorthi, V. Arul Suyambunathan, Z. Bobby and N. Selvaraj, Bitter gourd (Momordica charantia) improves insulin sensitivity by increasing skeletal muscle insulin-stimulated IRS-1 tyrosine phosphorylation in high-fat-fed rats, Br. J. Nutr., 2008, 99, 806–812 CrossRef CAS PubMed.
- Z. Q. Wang, X. H. Zhang, Y. Yu, A. Poulev, D. Ribnicky, Z. E. Floyd and W. T. Cefalu, Bioactives from bitter melon enhance insulin signaling and modulate acyl carnitine content in skeletal muscle in high-fat diet-fed mice, J. Nutr. Biochem., 2011, 22, 1064–1073 CrossRef CAS PubMed.
- K. N. Lu, C. Hsu, M. L. Chang and C. J. Huang, Wild bitter gourd increased metabolic rate and up-regulated genes related to mitochondria biogenesis and UCP-1 in mice, J. Funct. Foods, 2013, 5, 668–678 CrossRef CAS.
- C. Y. Hsiao, Y. M. Chen, Y. J. Hsu, C. C. Huang, H. C. Sung and S. S. Chen, Supplementation with Hualian No. 4 wild bitter gourd (Momordica charantia Linn. var. abbreviata ser.) extract increases anti-fatigue activities and enhances exercise performance in mice, J. Vet. Med. Sci., 2017, 79, 1110–1119 CrossRef CAS PubMed.
- C. Y. Chao and C. J. Huang, Bitter gourd (Momordica charantia) extract activates peroxisome proliferator-activated receptors and upregulates the expression of the acyl CoA oxidase gene in H4IIEC3 hepatoma cells, J. Biomed. Sci., 2003, 10, 782–791 Search PubMed.
- C. Hsu, C. L. Hsieh, Y. H. Kuo and C. J. Huang, Isolation and identification of cucurbitane-type triterpenoids with partial agonist/antagonist potential for estrogen receptors from Momordica charantia, J. Agric. Food Chem., 2011, 59, 4553–4561 CrossRef CAS PubMed.
- P. G. Reeves, F. H. Nielsen and G. C. Fahey Jr., AIN-93 purified diets for laboratory rodents: final report of the American Institute of Nutrition ad hoc writing committee on the reformulation of the AIN-76A rodent diet, J. Nutr., 1993, 123, 1939–1951 CrossRef CAS PubMed.
- A. Bonetto, D. C. Andersson and D. L. Waning, Assessment of muscle mass and strength in mice, BoneKEy Rep., 2015, 4, 732 Search PubMed.
- L. T. Yoshime, I. L. P. de Melo, J. A. G. Sattler, E. B. T. de Carvalho and J. Mancini-Filho, Bitter gourd (Momordica charantia L.) seed oil as a naturally rich source of bioactive compounds for nutraceutical purposes, Nutrire, 2016, 41, 12 CrossRef.
- T. Tsuzuki, Y. Tokuyama, M. Igarashi, K. Nakagawa, Y. Ohsaki, M. Komai and T. Miyazawa, Alpha-eleostearic acid (9Z11E13E-18:3) is quickly converted to conjugated linoleic acid (9Z11E-18:2) in rats, J. Nutr., 2004, 134, 2634–2639 CrossRef CAS PubMed.
- G. Yuan, H. Sun, A. J. Sinclair and D. Li, Effects of conjugated linolenic acid and conjugated linoleic acid on lipid metabolism in mice, Eur. J. Lipid Sci. Technol., 2009, 111, 537–545 CrossRef CAS.
- H. De Naeyer, S. Lamon, A. P. Russell, I. Everaert, A. De Spaey, B. Vanheel, Y. Taes and W. Derave, Androgenic and estrogenic regulation of Atrogin-1, MuRF1 and myostatin expression in different muscle types of male mice, Eur. J. Appl. Physiol., 2014, 114, 751–761 CrossRef CAS PubMed.
- C. Y. Lin, M. T. Lin, R. T. Cheng and S. H. Chen, Testosterone depletion by castration may protect mice from heat-induced multiple organ damage and lethality, J. Biomed. Biotechnol., 2010, 2010, 485306 Search PubMed.
- R. Narayanan, C. C. Coss, M. Yepuru, J. D. Kearbey, D. D. Miller and J. T. Dalton, Steroidal androgens and nonsteroidal, tissue-selective androgen receptor modulator, S-22, regulate androgen receptor function through distinct genomic and nongenomic signaling pathways, Mol. Endocrinol., 2008, 22, 2448–2465 CrossRef CAS PubMed.
- L. Min, T. Yanase, T. Tanaka, W. Fan, M. Nomura, H. Kawate, T. Okabe, R. Takayanagi and H. Nawata, A novel synthetic androgen receptor ligand, S42, works as a selective androgen receptor modulator and possesses metabolic effects with little impact on the prostate, Endocrinology, 2009, 150, 5606–5616 CrossRef CAS PubMed.
- I. Sinha, A. P. Sinha-Hikim, A. J. Wagers and I. Sinha-Hikim, Testosterone is essential for skeletal muscle growth in aged mice in a heterochronic parabiosis model, Cell Tissue Res., 2014, 357, 815–821 CrossRef CAS PubMed.
- I. Sinha-Hikim, J. Artaza, L. Woodhouse, N. Gonzalez-Cadavid, A. B. Singh, M. I. Lee, T. W. Storer, R. Casaburi, R. Shen and S. Bhasin, Testosterone-induced increase in muscle size in healthy young men is associated with muscle fiber hypertrophy, Am. J. Physiol.: Endocrinol. Metab., 2002, 283, E154–E164 CrossRef CAS PubMed.
- S. Bhasin, L. Woodhouse, R. Casaburi, A. B. Singh, D. Bhasin, N. Berman, X. Chen, K. E. Yarasheski, L. Magliano, C. Dzekov, J. Dzekov, R. Bross, J. Phillips, I. Sinha-Hikim, R. Shen and T. W. Storer, Testosterone dose-response relationships in healthy young men, Am. J. Physiol.: Endocrinol. Metab., 2001, 281, E1172–E1181 CrossRef CAS PubMed.
- D. Brown, A. P. Hikim, E. L. Kovacheva and I. Sinha-Hikim, Mouse model of testosterone-induced muscle fiber hypertrophy: involvement of p38 mitogen-activated protein kinase-mediated Notch signaling, J. Endocrinol., 2009, 201, 129–139 CAS.
- Y. Wu, W. Zhao, J. Zhao, J. Pan, Q. Wu, Y. Zhang, W. A. Bauman and C. P. Cardozo, Identification of androgen response elements in the insulin-like growth factor
I upstream promoter, Endocrinology, 2007, 148, 2984–2993 CrossRef CAS PubMed.
- F. R. Jornayvaz and G. I. Shulman, Regulation of mitochondrial biogenesis, Essays Biochem., 2010, 47, 69–84 CrossRef CAS PubMed.
- Z. Wu, P. Puigserver, U. Andersson, C. Zhang, G. Adelmant, V. Mootha, A. Troy, S. Cinti, B. Lowell, R. C. Scarpulla and B. M. Spiegelman, Mechanisms controlling mitochondrial biogenesis and respiration through the thermogenic coactivator PGC-1, Cell, 1999, 98, 115–124 CrossRef CAS PubMed.
- A. Vainshtein, E. M. Desjardins, A. Armani, M. Sandri and D. A. Hood, PGC-1alpha modulates denervation-induced mitophagy in skeletal muscle, Skeletal Muscle, 2015, 5, 9 CrossRef PubMed.
- J. Perez-Schindler, K. Svensson, E. Vargas-Fernandez, G. Santos, W. Wahli and C. Handschin, The coactivator PGC-1alpha regulates skeletal muscle oxidative metabolism independently of the nuclear receptor PPARbeta/delta in sedentary mice fed a regular chow diet, Diabetologia, 2014, 57, 2405–2412 CrossRef CAS PubMed.
- J. Lin, H. Wu, P. T. Tarr, C. Y. Zhang, Z. Wu, O. Boss, L. F. Michael, P. Puigserver, E. Isotani, E. N. Olson, B. B. Lowell, R. Bassel-Duby and B. M. Spiegelman, Transcriptional co-activator PGC-1 alpha drives the formation of slow-twitch muscle fibres, Nature, 2002, 418, 797–801 CrossRef CAS PubMed.
- C. Zechner, L. Lai, J. F. Zechner, T. Geng, Z. Yan, J. W. Rumsey, D. Collia, Z. Chen, D. F. Wozniak, T. C. Leone and D. P. Kelly, Total Skeletal Muscle PGC-1 Deficiency Uncouples Mitochondrial Derangements from Fiber Type Determination and Insulin Sensitivity, Cell Metab., 2010, 12, 633–642 CrossRef CAS PubMed.
- C. Ibebunjo, J. K. Eash, C. Li, Q. Ma and D. J. Glass, Voluntary running, skeletal muscle gene expression, and signaling inversely regulated by orchidectomy and testosterone replacement, Am. J. Physiol.: Endocrinol. Metab., 2011, 300, E327–E340 CrossRef CAS PubMed.
- A. Kukat, S. A. Dogan, D. Edgar, A. Mourier, C. Jacoby, P. Maiti, J. Mauer, C. Becker, K. Senft, R. Wibom, A. P. Kudin, K. Hultenby, U. Flogel, S. Rosenkranz, D. Ricquier, W. S. Kunz and A. Trifunovic, Loss of UCP2 attenuates mitochondrial dysfunction without altering ROS production and uncoupling activity, PLoS Genet., 2014, 10, e1004385 CrossRef PubMed.
- Y. W. Fridell, A. Sanchez-Blanco, B. A. Silvia and S. L. Helfand, Targeted expression of the human uncoupling protein 2 (hUCP2) to adult neurons extends life span in the fly, Cell Metab., 2005, 1, 145–152 CrossRef CAS PubMed.
- T. van Wessel, A. de Haan, W. J. van der Laarse and R. T. Jaspers, The muscle fiber type-fiber size paradox: hypertrophy or oxidative metabolism?, Eur. J. Appl. Physiol., 2010, 110, 665–694 CrossRef CAS PubMed.
- V. Ljubicic, P. Miura, M. Burt, L. Boudreault, S. Khogali, J. A. Lunde, J. M. Renaud and B. J. Jasmin, Chronic AMPK activation evokes the slow, oxidative myogenic program and triggers beneficial adaptations in mdx mouse skeletal muscle, Hum. Mol. Genet., 2011, 20, 3478–3493 CrossRef CAS PubMed.
- R. Mounier, L. Lantier, J. Leclerc, A. Sotiropoulos, M. Foretz and B. Viollet, Antagonistic control of muscle cell size by AMPK and mTORC1, Cell Cycle, 2011, 10, 2640–2646 CrossRef CAS PubMed.
- A. Y. Chan and J. R. Dyck, Activation of AMP-activated protein kinase (AMPK) inhibits protein synthesis: a potential strategy to prevent the development of cardiac hypertrophy, Can. J. Physiol. Pharmacol., 2005, 83, 24–28 CrossRef CAS PubMed.
- R. Mounier, L. Lantier, J. Leclerc, A. Sotiropoulos, M. Pende, D. Daegelen, K. Sakamoto, M. Foretz and B. Viollet, Important role for AMPKalpha1 in limiting skeletal muscle cell hypertrophy, FASEB J., 2009, 23, 2264–2273 CrossRef CAS PubMed.
- H. L. Cheng, H. K. Huang, C. I. Chang, C. P. Tsai and C. H. Chou, A cell-based screening identifies compounds from the stem of Momordica charantia that overcome insulin resistance and activate AMP-activated protein kinase, J. Agric. Food Chem., 2008, 56, 6835–6843 CrossRef CAS PubMed.
- M. J. Tan, J. M. Ye, N. Turner, C. Hohnen-Behrens, C. Q. Ke, C. P. Tang, T. Chen, H. C. Weiss, E. R. Gesing, A. Rowland, D. E. James and Y. Ye, Antidiabetic activities of triterpenoids isolated from bitter melon associated with activation of the AMPK pathway, Chem. Biol., 2008, 15, 263–273 CrossRef CAS PubMed.
- S. Jager, C. Handschin, J. St-Pierre and B. M. Spiegelman, AMP-activated protein kinase (AMPK) action in skeletal muscle via direct phosphorylation of PGC-1alpha, Proc. Natl. Acad. Sci. U. S. A., 2007, 104, 12017–12022 CrossRef PubMed.
- C. Y. Chuang, C. Hsu, C. Y. Chao, Y. S. Wein, Y. H. Kuo and C. J. Huang, Fractionation and identification of 9c, 11t, 13t-conjugated linolenic acid as an activator of PPARalpha in bitter gourd (Momordica charantia L.), J. Biomed. Sci., 2006, 13, 763–772 CrossRef CAS PubMed.
- C. Hsu, T. H. Tsai, Y. Y. Li, W. H. Wu, C. J. Huang and P. J. Tsai, Wild bitter melon (Momordica charantia Linn. var. abbreviata Ser.) extract and its bioactive components suppress Propionibacterium acnes-induced inflammation, Food Chem., 2012, 135, 976–984 CrossRef CAS PubMed.
- T. N. Huang, K. N. Lu, Y. P. Pai, H. Chin and C. J. Huang, Role of GLP-1 in the Hypoglycemic Effects of Wild Bitter Gourd, Evid. Based Complement. Alternat. Med., 2013, 2013, 625892 Search PubMed.
- M. W. Pariza, Y. Park and M. E. Cook, The biologically active isomers of conjugated linoleic acid, Prog. Lipid Res., 2001, 40, 283–298 CrossRef CAS PubMed.
- C. H. Tsai, E. C. F. Chen, H. S. Tsay and C. J. Huang, Wild bitter gourd improves metabolic syndrome: a preliminary dietary supplementation trial, Nutr. J., 2012, 11, 4–4 CrossRef CAS PubMed.
Footnote |
† Electronic supplementary information (ESI) available. See DOI: 10.1039/c8fo01777h |
|
This journal is © The Royal Society of Chemistry 2019 |
Click here to see how this site uses Cookies. View our privacy policy here.