Assessment of Cu and CuO nanoparticle ecological responses using laboratory small-scale microcosms†
Received
11th September 2019
, Accepted 18th November 2019
First published on 20th November 2019
Abstract
Copper based nanoparticles (NPs) are used extensively in industrial and commercial products as sensors, catalysts, surfactants, antimicrobials, and for other purposes. The high production volume and increasing use of copper-based NPs make their ecological risk a concern. Commonly used copper-based NPs are composed of metallic copper or copper oxide (Cu and CuO NPs); however, their environmental toxicity can vary dramatically depending on their physico-chemical properties, such as dissolution, aggregation behavior, and the generation of reactive oxygen species. Here, we investigated the NP dissolution, organismal uptake and aquatic toxicity of Cu and CuO NPs at 0, 0.1, 1, 5 or 10 mg Cu per L using a previously developed multi-species microcosm. This 5 day microcosm assay was comprised of C. reinhardtti, E. coli, D. magna, and D. rerio. We hypothesized that Cu and CuO NPs can elicit differential toxicity to the organisms due to alterations in particle dissolution and variations in organismal uptake. The actual concentrations of dissolved Cu released from the NPs were compared to ionic copper controls (CuCl2) at the same concentrations to determine the relative contribution of particulate and dissolved Cu on organism uptake and toxicity. We found that both NPs had higher uptake in D. magna and zebrafish than equivalent ionic exposures, suggesting that both Cu-based NPs are taken up by organisms. Cu NP exposures significantly inhibited algal growth rate, D. magna survival, and zebrafish hatching while exposure to equivalent concentrations of CuCl2 (dissolved Cu fraction) and CuO NPs did not. This indicates that Cu NPs themselves likely elicited a particle-specific mechanism of toxicity to the test organisms, or a combination effect from ionic Cu and the Cu NPs. Overall, this work was the first study to utilize a small-scale rapid assay designed to evaluate the fate and ecotoxicological impacts of Cu and CuO NPs in a mixed aquatic community.
Environmental significance
This work utilizes a small-scale rapid assay designed to evaluate the fate and ecotoxicological impacts of copper (Cu) based nanoparticles (NP) in a mixed aquatic community. The assay can generate large datasets for screening environmental impacts of nanomaterials to inform nanomaterial design and risk management. Once again, this study addresses the importance of investigating the ecological impacts of NPs in a more environmentally realistic test conditions, meanwhile, provides much-needed knowledge about the potential ecological impacts of Cu based NPs. Results demonstrated that Cu NPs likely elicited a particle-specific mechanism of toxicity to the community, or a combination effect from ionic Cu and the Cu NPs. We propose this testing framework is an effective addition to existing testing paradigms to meet current and future needs of the nanoscience community for rapid evaluations of NPs ecological risks and concerns.
|
1. Introduction
Environmentally relevant nanomaterial (NM) ecotoxicological assessments are essential to evaluate the risks of engineered NMs; however, these are difficult to conduct due to the intense financial and labor resources they require, as well as the regulatory requirements for in situ environmental NM exposures. Thus, there remains a large gap in our understanding of ecological impacts of NMs, and single species toxicity testing lacks the environmental diversity that defines ecological risk, making it difficult to truly define the ecotoxicity of NMs.1,2 Alternatively, large-scale mesocosms provide more environmentally relevance, but are still prohibitive in cost, time, and resources and require massive amounts of materials, space, and animals when one considers the current accelerated pace of NM development.3 As such, rapid alternative testing strategies utilizing multiple species from differing trophic levels are necessary to close this data gap and prioritize the wide diversity of engineered NMs for more intensive investigation.4,5
The aquatic environment is particularly at risk for exposure to engineered NMs as is it a natural sink for pollutants and a natural vehicle for pollutant migration.6 This includes copper-based nanoparticles (NPs) entering wastewater streams following industrial and commercial use.5–8 Copper-based NPs are widely used in industrial and commercial products as sensors (49%), catalysts (20%), surfactants (6%), antimicrobials (4%), and other purposes (21%) such antifouling paints.9–12 The high production volume and increasing use of copper-based NPs make their ecological risk a concern.13 Copper-based NP toxicity to individual species has been investigated for multiple aquatic organisms.11 Toxicity can be caused by ionic Cu released from copper-based NPs, this is considered by many to be the main mechanism of toxicity to a variety of aquatic organisms.14–17 Despite this finding, others have suggested that when both ions and NPs are present, non-additive toxicological responses are observed which are indicative of particle-specific mechanism(s) of toxicity.18
Commonly used copper-based NPs are composed of metallic copper or copper oxide (Cu and CuO NPs); however, their toxicity to aquatic organisms can vary dramatically. For instance, the reported LC50 values for Cu NPs in developing zebrafish range from 0.22–24 mg Cu per L,15,16,19–22 while CuO NPs are significantly less toxic, with values reported between 64–840 mg Cu per L.21,23–25 LC50 values of CuO NP exposure to a freshwater crustacean Daphnia magna (D. magna) has been reported to vary from 0.06–9.80 mg L−1;26–29 in comparison, only a few studies investigated the toxicity of Cu NP, where one study reported Cu NP has a LC50 to D. magna at 0.093 mg L−1,10 and other studies reported LC50 at 0.047–0.419 mg L−1 for Ceriodaphnia dubia.29,30 Garner et al. summarized and compared the toxicity of Cu and CuO NP using the species sensitivity distributions (SSDs) for freshwater organisms and results indicated that the toxicity threshold was much higher for CuO NPs relative to Cu NPs, suggesting a higher toxicological concern for Cu NPs.31 A recent study conducted by Keller et al. ranked toxicity based on high-throughput screening assays and found a general toxicity trend, with the toxicity of Cu2+ > nano-Cu > nano-CuO ≈ nano-Cu(OH)2 > micro-Cu ≈ micro-CuO.12 In addition, Adeleye et al. observed a higher fraction of free Cu2+ in Cu NPs dissolution than CuO NPs in aqueous environment, which may make Cu NP more toxic to pelagic organisms.32 The differences in dissolution and surface reactivity, such as reactive oxygen species (ROS) generation, which closely related to the surface oxidation of Cu NPs are previously explained for the difference in observed toxicity.12,33 For instance, higher exposure to Cu NPs resulted in oxidative stress (measured by biotic ROS generation) for E. coli and L. brevis, but there was no effect from CuCl2, CuSO4, micro-Cu or micro-CuO at the concentrations studied ([Cu] up to 250 mg L−1).34 Metallic Cu and CuO NP are theoretically considered insoluble in aqueous solutions at neutral pH, yet Cu ion release is commonly detected from copper-based nanomaterials in various solutions.35–37 The dissolution of metallic Cu generates ROS, while similar ROS generation from the dissolution of CuO is unclear.38,39 In addition, Cu NPs can form a thin oxidized surface layer when exposed to an oxygenated ambient environment, transforming the surface reactivity and adsorption properties of the particle.40 Additionally, Cu and CuO NPs may also have different aggregation tendencies (even if primary particle size is held constant) due to the difference in their surface interactions with each other and the surrounding environment. With any alteration to the surface of the NP, comes the potential for alterations in its ability to release Cu ions.41 All these factors can contribute to the difference between Cu and CuO NPs, in terms of their corresponding toxicity.
In this study, we aimed to investigate and compare the ecotoxicological impacts of Cu and CuO NPs. To compare the two types of nanoparticles, we employed a previously developed small-scale microcosm assay (nanocosm) in which four species (bacteria, algae, crustacean, and fish) are used to rapidly evaluate the fate and toxicity of NMs.5 Previously, we found that increased community complexity can mitigate the toxicity of soluble NMs when compared to individual species exposures due to environmental resilience.42 The same responses are expected in present study with copper exposures. In addition, we hypothesize that Cu NPs and CuO NPs will elicit differential toxicity to the nanocosm system, with crustaceans being the most sensitive organisms, due to variations in released ionic Cu from the NPs and organismal uptake. We use copper chloride as an ionic control by matching the dissolved Cu fraction from Cu and CuO NPs to determine the relative contribution of Cu ions to uptake and toxicity.
2. Materials and methods
2.1 NP characterization
CuO NPs and CuCl2 were purchased from Sigma Aldrich (St. Louis, MO, USA), and Cu NPs were purchase from Alfa Aesar (Ward Hill, MA, USA). Both Cu and CuO NPs were <50 nm in diameter, and the Cu NPs had a 1.4 nm oxidized copper outer shell. Hydrodynamic diameter (HDD) and zeta potential (ZP) were measured by dynamic light scattering using a Malvern Zetasizer (Nano ZS, Malvern Instruments, Worcestershire, UK) every 24 hours up to 120 hours at 10 mg Cu per L. HDD and ZP were measured in exposure media (pH = 7.2 ± 0.2), hereafter referred to as nanocosm media (NCM). Each measurement was taken in triplicate. The detailed parameters for HDD and ZP measurements are described in Table S1.† Transmission electron microscopy (TEM) and energy-dispersive X-ray spectroscopy (EDS) analysis were performed using a FEI Titan TEM with ChemiSTEM capability (ThermoFisher Scientific, Hillsboro, OR, USA). X-ray diffraction (XRD) of both NPs were conducted using a Bruker D2 Phaser (BRUKER AXS, Inc., Madison, WI, USA).
2.2 Exposure setup and toxicity evaluation
The detailed culture information for C. reinhardtii, E. coli, D. magna and embryonic zebrafish (D. rerio) followed previously established methods.5 Briefly, C. reinhardtii was cultured in TAP media and E. coli was purchased from Carolina Biological Supply Company (MicroKwik culture, Burlington, NC, USA) and cultured in Lysogeny broth (LB) media on a shaker at 20 °C and 37 °C, respectively. D. magna were maintained in reconstituted moderately hard water in reverse osmosis water and fed dry spirulina daily.43 The pH of the reconstituted water was measured every other day and maintained in the range of 7.8 ± 0.2. Adult wild-type zebrafish were maintained at the Sinnhuber Aquatic Research Laboratory (SARL) at Oregon State University. Embryos were collected from group spawns and staged to ensure all embryos were 6–8 hours post-fertilization (hpf) at the start of the experiment.
To set up a nanocosm, C. reinhardtii (in exponential growth period) were inoculated into 50 mL Falcon® vented tissue culture flasks (Fisher Scientific, Pittsburgh, PA) at a starting density of ∼2 × 104 cells per mL. D. magna neonates (<24 hours old) were collected from the stock culture and placed in NCM for a 24 hour acclimation period prior to toxicity testing. After 24 hours of incubation, E. coli inoculates were added to the flasks at a density of ∼5 × 105 cells per mL. Initial algal and bacterial cell densities were quantified using an Accuri C6 flow cytometer (BD Biosciences, San Jose, CA) to ensure consistent densities at the start of the experiment. Five NCM-acclimated daphnid neonates (24–48 hours life stage) and eight zebrafish embryos (8 hpf) were introduced into corresponding flasks to sequentially increase community complexity. All organisms were maintained at room temperature with a 16
:
8 h light:dark photoperiod under 1690 ± 246 lux light intensity provided by full-spectrum growth lights. Four nanocosm replicates were prepared for both the Cu and CuO NPs exposures at concentrations at 0, 0.1, 1, 5 or 10 mg Cu per L. CuCl2 was used as an ionic comparison, and the concentrations were determined based on the measured dissolved Cu concentrations found in NPs exposures at 1 (0.47 mg Cu per L), 5 (1.97 mg Cu per L), and 10 mg Cu per L (2.69 mg Cu per L).
Algal and bacterial viability were measured as the proportion of live:dead cells every 24 hours during the experiment. A 200 μL sample from each exposure flask was stained with 0.2 μL SYTOX green dead cell stain (Life Technologies #534860, Grand Island, NY), incubated in the dark for 15–20 minutes, and then analyzed via flow cytometry (BD Accuri C6, San Jose, CA). D. magna mortality was recorded daily. At the end of the 120 hour experimental period, live daphnids were removed from the experimental flasks and imaged using an Olympus SC100 high-resolution digital color camera (Olympus Corporation, Center Valley, PA). Zebrafish embryo hatching and mortality were monitored daily. At the end of the five-day exposure, zebrafish embryos were examined under a dissecting microscope for malformations (body axis, brain, heart, eyes, fins, jaw, trunk, and somite), physiological abnormalities (pigmentation, impaired circulation, pericardial edema, and yolk sac edema), and the presence of a touch response. Zebrafish developmental stage at our experimental temperature was corrected using eqn (1):
| 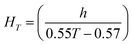 | (1) |
where
HT represents the hours of development at temperature
T, and
h represents the hours of development to reach that stage at 28.5 °C.
44
2.3 Dissolution and organism uptake measurements
Abiotic Cu NPs and CuO NPs dissolution in the NCM was measured by collecting three replicate 0.5 mL samples of the 10 mg L−1 suspension at 0, 24, 72, and 120 hours. Biotic dissolution was measured at the end of 120 hour period by gently agitating the flasks prior to sampling to resuspend any settled NPs or sediment, then collecting a 0.5 mL sample from each replicate nanocosm flask. Samples were centrifuged at 8000 × g for 10 minutes through 3 kDa polyethersulfone (PES) membrane centrifugal filter (VWR # 82031-344) to remove particulates 0.45 mL of the filtrate was transferred to a polystyrene tube and stored at −4 °C until analysis of copper content by inductively coupled plasma optical emission spectrometry (ICP-OES, Teledyne Technologies, Hudson, NH). Known concentrations of CuCl2 were similarly prepared and confirmed minimal ionic Cu loss during centrifugation. Copper ICP standards were purchased from RICCA Chemical Company (Arlington, TX). Three sample replicates were prepared and measured from each exposure conditions.
Cu uptake by daphnids and zebrafish was measured following toxicological observations at the end of the experiment. Individual daphnids and zebrafish were rinsed three times with ultrapure water (Millipore Milli-Q, Burlington, MA) to remove loosely attached algae, bacteria, and NPs. Unhatched zebrafish were manually dissected to remove the chorionic membrane. Washed D. magna, naturally hatched zebrafish, manually detached chorions and zebrafish from four nanocosm replicates were then collected and distributed to three sample replicates. All samples were stored at −4 °C in polystyrene tubes until acid digestion was performed to prepare the samples for ICP-OES analysis of Cu content. Samples were thawed and digested in PTFE tubes at 200 °C with 3 mL 70% trace-metal grade nitric acid.45 The acid was evaporated, and the process repeated a total of three times. Afterwards, trace-metal grade nitric acid (0.3 mL) was added to each PTFE tube while the tube was still hot, followed by 4.7 mL of ultrapure water, bringing the final sample volume to 5 mL with a final concentration of 3% nitric acid. All samples were measured in triplicate. D. magna mean dry mass was obtained from the mean weight of 6 groups of 10 daphnids. Zebrafish dry mass was estimated based on work by Hachicho et al. 2015, using the normalized development at the experimental temperature of 20.5 °C.46
The maximum dissolved Cu concentration from the exposures (pH = 7.2) determined by ICP-OES was further used to model the speciation of dissolved Cu using Visual MINTEQ version 3.1 (downloaded from https://vminteq.lwr.kth.se/) (Table S3†).
2.4 Statistics and calculations
SigmaPlot version 13.0 (Systat Software, San Jose, CA, USA) was used to perform statistical analyses. Dissolved Cu concentrations were compared using one-way ANOVA. The dissolution rate constant (k) and maximum dissolved Cu ([Cudiss]max) was calculated by fitting a first order reaction (eqn (2)) and compared using one-way ANCOVA: | [Cudiss] = [Cudiss]max[1 − e−kt] | (2) |
Algal and bacterial growth rates were modelled using a three-parameter logistic model (eqn (3)): | 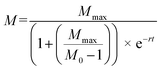 | (3) |
where r is the specific growth rate (h−1), t is the time (hour), Mmax represents the maximum capacity of cells, M0 is the initial cell counts at t = 0, and M represents the cell count at t = t.47 Algal and bacterial survival was corrected using eqn (4) to compensate for possible differences in the surviving proportion of organisms occurring post-treatment: |  | (4) |
where nC is the survival proportion (live cell/total cell) in the control group, nT is the survival proportion in the treatment group at time T, and a and b designate after and before treatment, respectively.48 The corrected algal and bacterial cell mortality in the presence of NPs was compared to control responses using a Kruskal–Wallis rank sum test followed by a Tukey post hoc test. D. magna survival among the control and treatments was compared using a two-way ANOVA with a Tukey post hoc test. Zebrafish hatching rates were compared using a Mann Whitney rank sum test, and developmental abnormalities in zebrafish were compared between treatments and the corresponding controls using a Fisher's exact test. All differences were considered statistically significant at p ≤ 0.05.
3. Results
3.1 NP characterization
Detailed characterization results are presented in the ESI† (Fig. S3, S4, S17–S21). Fig. 1 represents the mean HDD and ZP of Cu and CuO NPs in NCM at 10 mg L−1 throughout the 120 hour period. Both NPs had rapid and significant agglomeration from their primary particle size, though size and ZP did not change over time and was not significantly different between particles (Fig. S1†). The average HDD of Cu NPs was 1137.1 ± 123.8 nm, and their corresponding ZP was −16.1 ± 0.84 mV, whereas CuO NPs had an average HDD of 900.4 ± 100.9 nm with an average ZP of −16.5 ± 0.76.
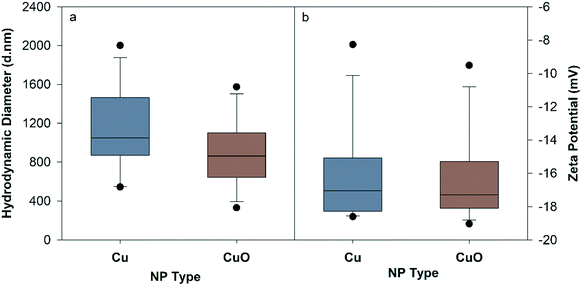 |
| Fig. 1 Hydrodynamic diameter (a) and zeta potential (b) of Cu and CuO NPs measured in nanocosm media (pH = 7.2 ± 0.2) at 10 mg Cu per L averaged over 120 hours. | |
3.2 Dissolved Cu measured in abiotic and biotic environment
The abiotic dissolution of Cu and CuO NPs was measured over 120 hours. Results show similar overall dissolution trends for both particles, despite statistically different Cu released at individual assessed time points (Fig. 2). CuO NPs had significantly higher dissolved Cu than Cu NPs at 16 and 24 hours, and Cu NPs had higher dissolution than CuO NPs at 2, 72, and 120 hours. To obtain the dissolution rates and maximum dissolved Cu, a first order exponential model was fit to the measured Cu concentrations. The dissolution rate constants and predicted maximum dissolved Cu released over 120 hour are shown in Table S2.† The rate constants suggest that CuO NPs released ions more rapidly than Cu NPs, with 0.0334 h−1 for CuO NP and 0.0246 h−1 for Cu NP respectively; however, the final equilibrium dissolved Cu concentration was predicted to be 14.3% higher for the Cu NPs (3.2 mg Cu per L) relative to the CuO NPs (2.8 mg Cu per L).
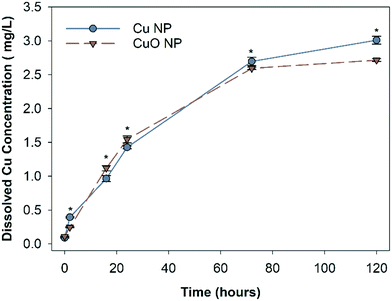 |
| Fig. 2 Abiotic Cu and CuO NP dissolution in nanocosm media (pH = 7.2 ± 0.2) at 10 mg Cu per L. * indicates significantly different between Cu and CuO NPs at each time point. Error bar represents standard error of three sample replicates. | |
The final dissolved Cu concentration measured in each nanocosm (when organisms were present) is shown in Fig. 3. At 1 mg Cu per L, CuO NP exposures had significantly more detectable dissolved Cu than Cu NP exposures; however, at 5 mg Cu per L, the Cu NP exposures resulted in significantly higher dissolved Cu. There was no difference in detected dissolved Cu between Cu NP and CuO NP exposures at 10 mg Cu per L. For CuCl2 exposures, the measured total Cu concentrations decreased by 11.4% and 10.3% than the initial Cu exposed (1.97 mg Cu per L and 2.69 mg Cu per L) in the biotic nanocosm environment after 120 hours (Fig. S2†). In addition, the dissolved Cu concentrations for both NPs measured in biotic environment were significantly decreased by 9.3% for Cu and 3.3% for CuO NPs when compared to those in the abiotic environment at 120 hour in 10 mg Cu per L exposures (comparing Fig. 2 and 3).
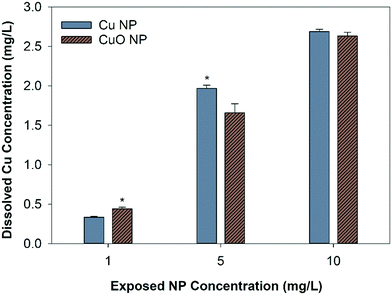 |
| Fig. 3 Dissolved Cu measured from Cu and CuO NPs at 1, 5, and 10 mg Cu per L after 120 hours. * indicates significant difference between Cu NPs and CuO NPs at the same concentration. Error bar represents standard error of three sample replicates. | |
3.3 Cu uptake in D. magna and zebrafish
There was no measurable Cu uptake in D. magna following CuCl2 exposures (Fig. 4a). In comparison, both Cu and CuO NP exposures resulted in significant Cu uptake in D. magna at 1, 5, and 10 mg Cu per L compared to control. This indicates that NPs contributed to the Cu accumulation in D. magna. D. magna exposed to CuO NPs had higher overall Cu uptake than those exposed to Cu NPs at 1, 5, and 10 mg Cu per L.
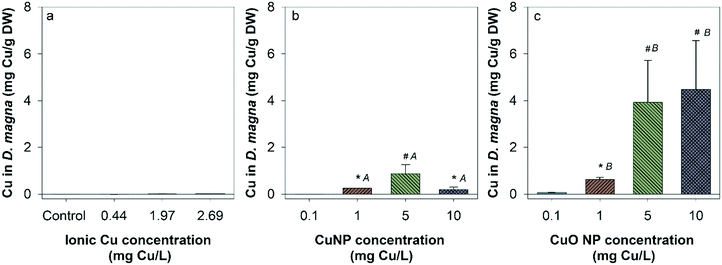 |
| Fig. 4 Cu uptake in D. magna from a) ionic Cu, b) Cu NP, and c) CuO NP at multiple exposure concentrations (control in panel a is the same for panels b and c). Error bar represents standard error of three sample replicates. Symbol * and # represents significant difference among exposure concentrations within the same Cu type exposure. Uppercase letters identify significant different Cu content in D. magna between CuO NP and Cu NP exposures under the same Cu concentrations. | |
Hatched zebrafish had extremely low Cu content across all exposure types and concentrations (Fig. S3†). Even when fish were pooled together from nanocosm replicates, Cu concentrations quantified from fish samples ranged from only 0.00053 to 0.0026 mg Cu per L, which is close to the detection limit for the instrument used (0.001 mg L−1). Thus, the uptake of Cu in zebrafish was too low to be confidently quantified in the present study. However, to determine the uptake of Cu in unhatched zebrafish, unhatched fish chorions were manually removed from zebrafish and stored separately for analysis of Cu content. At the highest exposure concentration, 5, 14, and 14 unhatched zebrafish were successfully separated for ionic Cu, CuO NP, and Cu NP exposures respectively. Chorion and zebrafish collected from each exposure were distributed to three groups for analysis (only one group for ionic Cu exposure due to the low quantity of unhatched zebrafish collected). Large amounts of Cu were measured in zebrafish chorions relative to zebrafish bodies in both Cu and CuO NP exposures, but not with CuCl2 exposure (Fig. 5). CuO NP exposure caused significantly more chorionic accumulation than Cu NP exposure. Table S4† summarizes the potential total Cu taken up by D. manga and zebrafish by using the mean concentrations for each exposure scenario, where CuO NP showed much higher total Cu accumulation (up to 18%) than Cu NP and ionic Cu exposures (Table S4†).
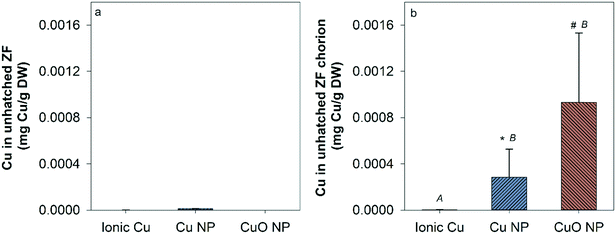 |
| Fig. 5 Cu uptake in unhatched zebrafish embryos after 120 hours (ionic Cu: 2.69 mg Cu per L; NP: 10 mg Cu per L). Panel a represents Cu content in manually dechorionated zebrafish body, and panel b represents Cu concentrations in the removed chorions. Error bar represents standard error of three sample replicates (technical replicates shown ionic Cu exposure groups due to limited unhatched zebrafish collected). Upper case letters indicate significant difference among three types of Cu exposure, and * indicates significant difference between measured Cu in chorion and zebrafish body. | |
3.4 Toxicity results
Fig. 6 summarizes the toxicity of all three Cu exposures based on the measured dissolved Cu concentrations. All three Cu exposure significantly reduced the algal growth rates at 120 hours. However, no other toxicological endpoint was observed in other organisms with CuO NP and CuCl2 exposures. In addition, bacterial growth rates were only affected by Cu NP exposure at 2.63 mg Cu per L (10 mg Cu NP per L) (Fig. 6b). The Cu NP exposure was also the only scenario to cause significant D. magna mortality (Fig. 6c), which occurred at 1.97 mg dissolved Cu per L and 2.69 mg dissolved Cu per L, equivalent to 5 and 10 mg Cu per L Cu NP exposure, respectively. Zebrafish hatching was significantly delayed with Cu NP exposure at 0.44 and 2.69 mg dissolved Cu per L (Fig. 6d). In addition, Cu NPs showed significantly higher toxicity to algae (2.69 mg dissolved Cu per L) and D. magna (1.97 and 2.69 mg dissolved Cu per L) when compared to CuO NPs and CuCl2 (Fig. 6a and c). Zebrafish hatching was significantly lower in Cu NP exposure than CuCl2 at 2.69 mg dissolved Cu per L (Fig. 6d). The detailed algal and bacterial growth rates, D. magna mortality data are also presented in Fig. S4 and S5.† A table summarizing the overall organism response of the exposed nanocosms is listed in the ESI† (Table S5).
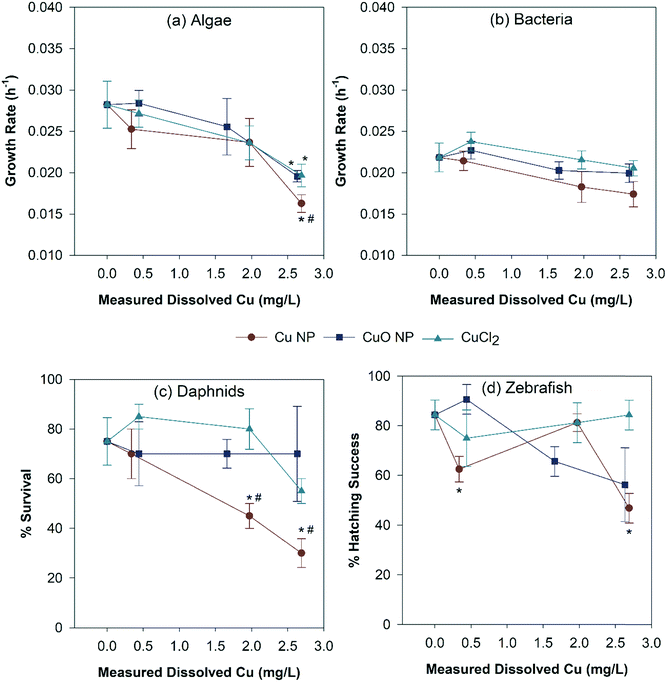 |
| Fig. 6 Evaluated toxicity endpoint in each tested organism using the measured dissolved Cu from each exposure type. * indicates significant difference compared to each corresponding control. # suggests significant differences when compare types of Cu exposure at the same dissolved Cu concentrations. Error bar represents standard error of four sample replicates. | |
4. Discussion
Rapid multi-species alternative testing strategies are necessary to address the large gap that still exists in understanding the ecological impacts of NMs to evaluate potential risks. We compared the ecotoxicological impacts of Cu and CuO NPs using a previously developed nanocosm assay to rapidly evaluate fate and toxicity of NMs in a community environment. We found that Cu NPs elicited significantly different toxicity to our nanocosm community than either CuO NPs or ionic Cu. In addition, organisms in the nanocosm had varied uptake behavior and susceptibility to the differences in types of Cu NMs.
Both NPs had significant agglomeration from their primary particle size and formed large agglomerates in solution, which was expected as NPs tend to agglomerate in electrolyte solutions due to compression of the double layer by the ions in the NCM.49 In the abiotic environment, the dissolved Cu concentration from Cu NPs was higher than what was found from CuO NPs at 120 hours; however, the overall trend was similar. Lower dissolved Cu concentrations were observed in biotic environments than abiotic for all three Cu sources, likely due to the presence of organisms that actively take up dissolved Cu species.50 Miao et al. found that the ratio of free Cu2+/total dissolved Cu significantly decreased in the presence of extracellular polymeric substances, therefore affecting the Cu speciation and bioavailability in aqueous environment.51 In addition, Cu and CuO NPs could be coated by extracellular organic species generated by the organisms or associate with microorganisms to decrease the available reactive surface, both of which inhibit dissolution. Phytoplankton-derived soluble extracellular polymeric substances have found to improve the stability of copper-based NPs and influence their dissolution, depending on the pH of the water and Cl−.32 Visual Minteq was used to model Cu speciation in our exposure environment. The results suggest that over 70% of Cu ions could form precipitates with PO43− under 1.97 and 2.69 mg Cu per L exposures (Table S3†). However, ICP-OES was used to quantify the ionic Cu controls after 120 hours in nanocosm, where only 10.3–11.4% Cu loss was found compared to the initial exposure concentrations (Fig. S2†). This loss suggests the organisms may have impacted precipitate formation. In addition, the 3 kDa membrane filter used before sample digestion may have removed some precipitates prior to analysis, contributing for the loss observed in the ionic control nanocosms over time. The dissolved Cu concentration released from NPs were present mainly as dissolved Cu and not precipitates. The similar dissolved Cu concentrations measured from both Cu and CuO NPs in the nanocosm exposures suggests that the dissolved Cu was unlikely the determinant factor in the differential toxicity observed between Cu exposures.
The Cu uptake in both D. magna and zebrafish significantly increased after exposure to both types of NPs but not ionic copper. One thing we did not investigate is the settled and suspended NP distribution in our nanocosm systems due to the technical difficulties. With the presence of organic matter in the microcosms, NPs were expected to settle over time50 and therefore have a higher potential to attach to the surface of the fish chorion. Another uncertainty in our system is that as filter feeders, D. magna were constant moving and stirring the exposure media, creating a dynamic mixing system in the nanocosm. Results indicate that CuO NP uptake was concentration dependent in both D. magna and zebrafish, while the same trend was not observed with the Cu NP exposures, despite the two particles having similar oxidized outer surface layers. In D. magna, this is likely due to their filter feeding behavior which could cause accumulation of NPs from the exposure environment; however, we would expect this to be similar as the NP surface chemistries and particle sizes were similar. Adam et al. have demonstrated that D. magna could accumulate Cu NPs following waterborne exposure.52 It could also be attributed to trophic transfer from preying on algae and bacteria if the two particles interacted with one or both species differently; however, given the similar surface chemistry, one would think there would be similar interactions with the organisms. We previously demonstrated that CuO NPs can be transferred through organisms in the food chain and accumulate in D. magna via preying on NP contaminated algae.53
In zebrafish, we found large amounts of Cu and CuO NPs associated with the unhatched fish chorion due to the sedimentation of large NP aggregates, but not in the body of the fish, suggesting that the chorion prevented the uptake of Cu and CuO NPs into the zebrafish directly. In addition, it provides strong evidence that the delayed hatching may have been caused by blockage of the chorionic pores by NPs, and Cu2+ released from NPs interference with metal-sensitive binding sites in the active center of the zebrafish hatching enzyme ZHE1.54,55 Keller et al. also indicate that Cu NPs can damage zebrafish DNA plasmids and affect embryo hatching enzymes.12 Jiang et al. previously investigated the uptake kinetics of CuO NP in Gulf killifish (Fundulus grandis), where the dissolved Cu fraction was the major contributor to the Cu uptake in embryonic fish.50 In our study, Cu uptake was not observed with ionic Cu exposures, so the measured Cu content in organisms was likely due to the uptake of NPs. Moreover, higher Cu content in organisms following CuO NP exposures leads us to hypothesize that CuO NPs may have a higher affinity for organic ligands than Cu NPs. This hypothesis was also previously proposed by Geitner et al., where they describe that surface affinity was the key factor for predicting the trophic transfer of NPs in aquatic organisms.56 However, in the present study, uptake did not correlate linearly to the toxicity observed, suggesting other parameters were dominating the toxicity in this study.
When normalized for dissolved Cu content, Cu NPs significantly inhibited algal growth rate, D. magna survival, and zebrafish hatching while CuCl2 and CuO NPs did not. This suggests that Cu NPs themselves elicited particle-specific toxicity to the tested organisms, or there was a combination effect from ionic Cu and Cu NPs.54 Metal NPs can cause toxicity by generating reactive oxygen species (ROS) to organisms.57,58 It has been demonstrated that Cu NP dissolution produces hydrogen peroxide and ROS as a byproduct of the process.38 In comparison, the dissolution of CuO NPs does not generate ROS, though intracellular ROS have been detected in other studies.59 Previously, we demonstrated that the likely mechanistic cause of the different toxicity between Cu NP and CuO NP in embryonic zebrafish was due to the ability of each NP generating ROS.60 In that study, Cu and CuO NPs were compared and higher toxicity was observed for Cu NPs which corresponded with more ROS generation (quantified as H2O2 equivalent), determined using a dichlorofluorescein (DCF) colorimetric assays.60 We observed the same toxicity trend in this study and propose that this phenomenon contributes in part to our findings. Similar conclusion was obtained by Keller et al., their results suggest that ROS production was an important toxicity mechanism of Cu NPs, along with other toxicity mechanisms such as membrane damage, decreased electron transport activity, degradation of plasmid DNA, decreased total antioxidant capacity, and developmental abnormalities, depending on the organisms tested.12 On the other hand, we found that Cu NP seemed more jagged than CuO NP based on the TEM analysis (Fig. S4†). Previous study demonstrated that CuO NP shape may also affect NP surface reactivity and toxicity.62 In that study, CuO nanosheets elicited higher surface reactivity, electrochemical activity, and antimicrobial property compared to sphere shape CuO NP and bulk CuO particles.61 In addition, significantly lower hatching delay was also observed with Cu NP exposure at the lower concentration (1 mg L−1 Cu NP) but not higher concentration (5 mg L−1 Cu N) compared to the corresponding control (Fig. 6d). The higher toxicity at 1 mg L−1 Cu NP exposure might due to the destabilization of Cu NPs at lower exposure concentrations, since colloidal stability is a fundamental driver of NP bioactivity, comprehensively accounting for otherwise inexplicable differential biological effects.62 Overall, the physicochemical properties of Cu and CuO NPs may dictates corresponding toxicity and should be investigated further. The toxicity of all three types of copper evaluated in this study was largely mitigated in the nanocosm compared to the LC50 values for individual species exposures obtained from the literature. For example, ionic copper is considered to be extremely toxic, with mean LC50 estimates of 0.03, 0.07, and 0.28 mg Cu per L for crustaceans, algae, and fish species Daphnia, respectively.11 In our study, we did not observe significant mortality until the concentration reached 1.96 mg Cu per L and decreased algal growth rate at 2.69 mg Cu per L. No impact on bacterial growth or zebrafish survival was observed at any test concentration. The lower toxicity observed in the nanocosm exposure is likely due to the presence of organic matter, species interactions, and organismal sinks. Organic matter can affect the toxicity and bioavailability of Cu metals to organisms by complexing metal ions,63,64 which can mitigate toxicity by reducing exposure to freely available ions.35,45,64 Community resilience can also contribute to mitigated toxicity due to distribution of exposed Cu to multiple species. We have previously demonstrated that the nanocosm exhibits environmental resistance to contaminants due to species interactions and organismal sinks.5,42 The resilience of the community can lead to mitigated toxicity due to the distribution of the exposed Cu to multiple species and lower bioavailable Cu. This provides more evidence that ecotoxicologically relevance should be taking into consideration, since exposure conditions are critical when evaluating ecotoxicity of nanomaterials.
5. Conclusion
Our findings support the hypothesis that Cu NPs have mechanisms of toxicity that differ from CuO NPs and ionic Cu. Cu NPs had higher toxicity than the corresponding dissolved Cu control, indicating Cu NP elicit toxicity not solely due to the release of Cu2+. Although higher Cu content in CuO NP exposed organisms was found, it does not correlate to the toxicity observed. Further work should investigate other mechanisms contributing to Cu NP toxicity and identify how NP surface affinity determines organism uptake behavior. This work again addresses the importance of investigating the environmental impacts of NPs in more environmentally realistic test conditions and provides much-needed knowledge about the potential ecological impacts of Cu based NPs. In addition, this assay can generate large datasets for screening environmental impacts of nanomaterials and inform nanomaterial design and risk management. The ultimate goal of this research is to develop a small-scale standardized assay to rapidly assess the ecological responses of NPs. We propose this testing framework as an effective addition to existing testing paradigms to meet current and future needs of the nanoscience community for rapid evaluations of NPs ecological risks and concerns. Future research will focus on system calibration and method development to reflect the range of whole assay responses to NP exposures.
Ethical statement
All experiments were performed in compliance with national care and use guidelines and approved by the Institutional Animal Care and Use Committee (IACUC) at Oregon State University.
Conflicts of interest
There are no conflicts to declare.
Acknowledgements
This work is funded by the National Science Foundation (NSF Grant # 1438165 and NSF Grant # 1762278) and the National Institutes of Health (Grant #ES017552). We thank the Sinnhuber Aquatic Research Laboratory (SARL) at Oregon State University for providing zebrafish embryos (NIEHS Grant #P30 ES000210).
References
- J. P. Stegemeier, A. Avellan and G. V. Lowry, Effect of Initial Speciation of Copper-and Silver-Based Nanoparticles on Their Long-Term Fate and Phytoavailability in Freshwater Wetland Mesocosms, Environ. Sci. Technol., 2017, 51, 12114–12122 CrossRef CAS PubMed.
- E. S. Bernhardt, B. P. Colman, M. F. Hochella, B. J. Cardinale, R. M. Nisbet, C. J. Richardson and L. Yin, An ecological perspective on nanomaterial impacts in the environment, J. Environ. Qual., 2010, 39, 1954–1965 CrossRef CAS.
- M. Auffan, M. Tella, C. Santaella, L. Brousset, C. Paillès, M. Barakat, B. Espinasse, E. Artells, J. Issartel and A. Masion, An adaptable mesocosm platform for performing integrated assessments of nanomaterial risk in complex environmental systems, Sci. Rep., 2014, 4, 5608 CrossRef CAS.
- B. P. Colman, L. F. Baker, R. S. King, C. W. Matson, J. M. Unrine, S. M. Marinakos, D. E. Gorka and E. S. Bernhardt, Dosing, Not the Dose: Comparing Chronic and Pulsed Silver Nanoparticle Exposures, Environ. Sci. Technol., 2018, 52, 10048–10056 CrossRef CAS.
- F. Wu, B. J. Harper and S. L. Harper, Differential dissolution and toxicity of surface functionalized silver nanoparticles in small-scale microcosms: impacts of community complexity, Environ. Sci.: Nano, 2017, 4, 359–372 RSC.
- B. Nowack and T. D. Bucheli, Occurrence, behavior and effects of nanoparticles in the environment, Environ. Pollut., 2007, 150, 5–22 CrossRef CAS.
- T. Scown, R. Van Aerle and C. Tyler, Review: do engineered nanoparticles pose a significant threat to the aquatic environment?, Crit. Rev. Toxicol., 2010, 40, 653–670 CrossRef CAS.
- A. A. Keller, S. McFerran, A. Lazareva and S. Suh, Global life cycle releases of engineered nanomaterials, J. Nanopart. Res., 2013, 15, 1692 CrossRef.
- E. Ebrahimnia-Bajestan, H. Niazmand, W. Duangthongsuk and S. Wongwises, Numerical investigation of effective parameters in convective heat transfer of nanofluids flowing under a laminar flow regime, Int. J. Heat Mass Transfer, 2011, 54, 4376–4388 CrossRef CAS.
- Y. Xiao, M. G. Vijver, G. Chen and W. J. Peijnenburg, Toxicity and Accumulation of Cu and ZnO nanoparticles in Daphnia magna, Environ. Sci. Technol., 2015, 49, 4657–4664 CrossRef CAS.
- O. Bondarenko, K. Juganson, A. Ivask, K. Kasemets, M. Mortimer and A. Kahru, Toxicity of Ag, CuO and ZnO nanoparticles to selected environmentally relevant test organisms and mammalian cells in vitro: a critical review, Arch. Toxicol., 2013, 87, 1181–1200 CrossRef CAS.
- A. A. Keller, A. S. Adeleye, J. R. Conway, K. L. Garner, L. Zhao, G. N. Cherr, J. Hong, J. L. Gardea-Torresdey, H. A. Godwin and S. Hanna, Comparative environmental fate and toxicity of copper nanomaterials, NanoImpact, 2017, 7, 28–40 CrossRef.
- A. N. Parks, M. G. Cantwell, D. R. Katz, M. A. Cashman, T. P. Luxton, K. T. Ho and R. M. Burgess, Assessing the release of copper from nanocopper-treated and conventional copper-treated lumber into marine waters I: Concentrations and rates, Environ. Toxicol. Chem., 2018, 37, 1956–1968 CrossRef CAS.
- P.-E. Buffet, M. Richard, F. Caupos, A. Vergnoux, H. Perrein-Ettajani, A. Luna-Acosta, F. Akcha, J.-C. Amiard, C. Amiard-Triquet and M. Guibbolini, A mesocosm study of fate and effects of CuO nanoparticles on endobenthic species (Scrobicularia plana, Hediste diversicolor), Environ. Sci. Technol., 2013, 47, 1620–1628 CAS.
- R. J. Griffitt, R. Weil, K. A. Hyndman, N. D. Denslow, K. Powers, D. Taylor and D. S. Barber, Exposure to copper nanoparticles causes gill injury and acute lethality in zebrafish (Danio
rerio), Environ. Sci. Technol., 2007, 41, 8178–8186 CrossRef CAS.
- J. Hua, M. G. Vijver, F. Ahmad, M. K. Richardson and W. J. Peijnenburg, Toxicity of different-sized copper nano-and submicron particles and their shed copper ions to zebrafish embryos, Environ. Toxicol. Chem., 2014, 33, 1774–1782 CrossRef CAS.
- S. K. Misra, S. Nuseibeh, A. Dybowska, D. Berhanu, T. D. Tetley and E. Valsami-Jones, Comparative study using spheres, rods and spindle-shaped nanoplatelets on dispersion stability, dissolution and toxicity of CuO nanomaterials, Nanotoxicology, 2014, 8, 422–432 CrossRef CAS.
- S. Lopes, C. Pinheiro, A. M. Soares and S. Loureiro, Joint toxicity prediction of nanoparticles and ionic counterparts: simulating toxicity under a fate scenario, J. Hazard. Mater., 2016, 320, 1–9 CrossRef CAS.
- W. Bai, W. Tian, Z. Zhang, X. He, Y. Ma, N. Liu and Z. Chai, Effects of copper nanoparticles on the development of zebrafish embryos, J. Nanosci. Nanotechnol., 2010, 10, 8670–8676 CrossRef CAS.
- D. Chen, D. Zhang, C. Y. Jimmy and K. M. Chan, Effects of Cu2O nanoparticle and CuCl2 on zebrafish larvae and a liver cell-line, Aquat. Toxicol., 2011, 105, 344–354 CrossRef CAS.
- J. A. Kovrižnych, R. Sotníková, D. Zeljenková, E. Rollerová, E. Szabová and S. Wimmerová, Acute toxicity of 31 different nanoparticles to zebrafish (Danio rerio) tested in adulthood and in early life stages–comparative study, Interdiscip. Toxicol., 2013, 6, 67–73 Search PubMed.
- L. Song, M. G. Vijver, W. J. Peijnenburg, T. S. Galloway and C. R. Tyler, A comparative analysis on the in vivo toxicity of copper nanoparticles in three species of freshwater fish, Chemosphere, 2015, 139, 181–189 CrossRef CAS PubMed.
- S. Ganesan, N. Anaimalai Thirumurthi, A. Raghunath, S. Vijayakumar and E. Perumal, Acute and sub-lethal exposure to copper oxide nanoparticles causes oxidative stress and teratogenicity in zebrafish embryos, J. Appl. Toxicol., 2016, 36, 554–567 CrossRef CAS PubMed.
- M. Heinlaan, M. Muna, M. Knöbel, D. Kistler, N. Odzak, D. Kühnel, J. Müller, G. S. Gupta, A. Kumar and R. Shanker, Natural water as the test medium for Ag and CuO nanoparticle hazard evaluation: an interlaboratory case study, Environ. Pollut., 2016, 216, 689–699 CrossRef CAS.
- S. Lin, Y. Zhao, T. Xia, H. Meng, Z. Ji, R. Liu, S. George, S. Xiong, X. Wang and H. Zhang, High content screening in zebrafish speeds up hazard ranking of transition metal oxide nanoparticles, ACS Nano, 2011, 5, 7284–7295 CrossRef CAS PubMed.
- M. Heinlaan, A. Ivask, I. Blinova, H.-C. Dubourguier and A. Kahru, Toxicity of nanosized and bulk ZnO, CuO and TiO2 to bacteria Vibrio fischeri and crustaceans Daphnia magna and Thamnocephalus platyurus, Chemosphere, 2008, 71, 1308–1316 CrossRef CAS PubMed.
- L. Manusadžianas, C. Caillet, L. Fachetti, B. Gylytė, R. Grigutytė, S. Jurkonienė, R. Karitonas, K. Sadauskas, F. Thomas and R. Vitkus, Toxicity of copper oxide nanoparticle suspensions to aquatic biota, Environ. Toxicol. Chem., 2012, 31, 108–114 CrossRef PubMed.
- J.-J. Yin, J. Liu, M. Ehrenshaft, J. E. Roberts, P. P. Fu, R. P. Mason and B. Zhao, Phototoxicity of nano titanium dioxides in HaCaT keratinocytes—generation of reactive oxygen species and cell damage, Toxicol. Appl. Pharmacol., 2012, 263, 81–88 CrossRef CAS PubMed.
- R. J. Griffitt, J. Luo, J. Gao, J. C. Bonzongo and D. S. Barber, Effects of particle composition and species on toxicity of metallic nanomaterials in aquatic organisms, Environ. Toxicol. Chem., 2008, 27, 1972–1978 CrossRef CAS PubMed.
- J. Gao, S. Youn, A. Hovsepyan, V. L. Llaneza, Y. Wang, G. Bitton and J.-C. J. Bonzongo, Dispersion and toxicity of selected manufactured nanomaterials in natural river water samples: effects of water chemical composition, Environ. Sci. Technol., 2009, 43, 3322–3328 CrossRef CAS PubMed.
- K. L. Garner, S. Suh, H. S. Lenihan and A. A. Keller, Species sensitivity distributions for engineered nanomaterials, Environ. Sci. Technol., 2015, 49, 5753–5759 CrossRef CAS PubMed.
- A. S. Adeleye, J. R. Conway, T. Perez, P. Rutten and A. A. Keller, Influence of extracellular polymeric substances on the long-term fate, dissolution, and speciation of copper-based nanoparticles, Environ. Sci. Technol., 2014, 48, 12561–12568 CrossRef CAS PubMed.
- M. Shi, H. S. Kwon, Z. Peng, A. Elder and H. Yang, Effects of surface chemistry on the generation of reactive oxygen species by copper nanoparticles, ACS Nano, 2012, 6, 2157–2164 CrossRef CAS PubMed.
- C. Kaweeteerawat, C. H. Chang, K. R. Roy, R. Liu, R. Li, D. Toso, H. Fischer, A. Ivask, Z. Ji and J. I. Zink, Cu nanoparticles have different impacts in Escherichia coli and Lactobacillus brevis than their microsized and ionic analogues, ACS Nano, 2015, 9, 7215–7225 CrossRef CAS.
- S. Lin, A. A. Taylor, J. Zhaoxia, C. H. Chang, N. M. Kinsinger, W. Ueng, S. L. Walker and A. E. Nel, Understanding the transformation, speciation, and hazard potential of copper particles in a model septic tank system using zebrafish to monitor the effluent, ACS Nano, 2015, 9, 2038 CrossRef CAS.
- G. A. Al-Bairuty, D. Boyle, T. B. Henry and R. D. Handy, Sublethal effects of copper sulphate compared to copper nanoparticles in rainbow trout (Oncorhynchus mykiss) at low pH: physiology and metal accumulation, Aquat. Toxicol., 2016, 174, 188–198 CrossRef CAS PubMed.
- R. D. Kent and P. J. Vikesland, Dissolution and persistence of copper-based nanomaterials in undersaturated solutions with respect to cupric solid phases, Environ. Sci. Technol., 2016, 50, 6772–6781 CrossRef CAS PubMed.
- S. Olszowka, M. Manning and A. Barkatt, Copper dissolution and hydrogen peroxide formation in aqueous media, Corrosion, 1992, 48, 411–418 CrossRef CAS.
-
D. A. Palmer and P. Bénézeth, Solubility of Copper Oxides in Water and Steam, 14th International Conference on the Properties of Water and Steam in Kyoto, 2008, pp. 491–496 Search PubMed.
- I. A. Mudunkotuwa, J. M. Pettibone and V. H. Grassian, Environmental implications of nanoparticle aging in the processing and fate of copper-based nanomaterials, Environ. Sci. Technol., 2012, 46, 7001–7010 CrossRef CAS PubMed.
- S.-W. Bian, I. A. Mudunkotuwa, T. Rupasinghe and V. H. Grassian, Aggregation and dissolution of 4 nm ZnO nanoparticles in aqueous environments: influence of pH, ionic strength, size, and adsorption of humic acid, Langmuir, 2011, 27, 6059–6068 CrossRef CAS PubMed.
- F. Wu, B. J. Harper and S. L. Harper, Comparative dissolution, uptake and toxicity of zinc oxide particles in individual aquatic species and mixed populations, Environ. Toxicol. Chem., 2019, 38, 591–602 CrossRef CAS.
-
U.S. Environmental Protection Agency, Methods for measuring the acute toxicity of effluents and receiving waters to freshwater and marine organisms, EPA-821-R-02-012, 2002 Search PubMed.
- C. B. Kimmel, W. W. Ballard, S. R. Kimmel, B. Ullmann and T. F. Schilling, Stages of embryonic development of the zebrafish, Dev. Dyn., 1995, 203, 253–310 CrossRef CAS PubMed.
- K.-T. Kim, L. Truong, L. Wehmas and R. L. Tanguay, Silver nanoparticle toxicity in the embryonic zebrafish is governed by particle dispersion and ionic environment, Nanotechnology, 2013, 24, 115101 CrossRef PubMed.
- N. Hachicho, S. Reithel, A. Miltner, H. J. Heipieper, E. Küster and T. Luckenbach, Body mass parameters, lipid profiles and protein contents of zebrafish embryos and effects of 2, 4-dinitrophenol exposure, PLoS One, 2015, 10, e0134755 CrossRef.
- C. Paine, T. R. Marthews, D. R. Vogt, D. Purves, M. Rees, A. Hector and L. A. Turnbull, How to fit nonlinear plant growth models and calculate growth rates: an update for ecologists, Methods Ecol. Evol., 2012, 3, 245–256 CrossRef.
- C. F. Henderson and E. W. Tilton, Tests with acaricides against the brown wheat mite, J. Econ. Entomol., 1955, 48, 157–161 CrossRef CAS.
- A. M. E. Badawy, T. P. Luxton, R. G. Silva, K. G. Scheckel, M. T. Suidan and T. M. Tolaymat, Impact of environmental conditions (pH, ionic strength, and electrolyte type) on the surface charge and aggregation of silver nanoparticles suspensions, Environ. Sci. Technol., 2010, 44, 1260–1266 CrossRef PubMed.
- C. Jiang, B. T. Castellon, C. W. Matson, G. R. Aiken and H. Hsu-Kim, Relative contributions of copper oxide nanoparticles and dissolved copper to Cu uptake kinetics of Gulf killifish (Fundulus grandis) embryos, Environ. Sci. Technol., 2017, 51, 1395–1404 CrossRef CAS.
- L. Miao, C. Wang, J. Hou, P. Wang, Y. Ao, Y. Li, B. Lv, Y. Yang, G. You and Y. Xu, Enhanced stability and dissolution of CuO nanoparticles by extracellular polymeric substances in aqueous environment, J. Nanopart. Res., 2015, 17, 404 CrossRef.
- N. Adam, F. Leroux, D. Knapen, S. Bals and R. Blust, The uptake of ZnO and CuO nanoparticles in the water-flea Daphnia magna under acute exposure scenarios, Environ. Pollut., 2014, 194, 130–137 CrossRef CAS PubMed.
- F. Wu, A. Bortvedt, B. J. Harper, L. E. Crandon and S. L. Harper, Uptake and toxicity of CuO nanoparticles to Daphnia magna varies between indirect dietary and direct waterborne exposures, Aquat. Toxicol., 2017, 190, 78–86 CrossRef CAS PubMed.
- E. B. Muller, S. Lin and R. M. Nisbet, Quantitative adverse outcome pathway analysis of hatching in zebrafish with CuO nanoparticles, Environ. Sci. Technol., 2015, 49, 11817–11824 CrossRef CAS PubMed.
- S. Lin, Y. Zhao, Z. Ji, J. Ear, C. H. Chang, H. Zhang, C. Low-Kam, K. Yamada, H. Meng and X. Wang, Zebrafish high-throughput screening to study the impact of dissolvable metal oxide nanoparticles on the hatching enzyme, ZHE1, Small, 2013, 9, 1776–1785 CrossRef CAS PubMed.
- N. K. Geitner, S. M. Marinakos, C. Guo, N. O'Brien and M. R. Wiesner, Nanoparticle surface affinity as a predictor of trophic transfer, Environ. Sci. Technol., 2016, 50, 6663–6669 CrossRef CAS PubMed.
- H. L. Karlsson, P. Cronholm, J. Gustafsson and L. Moller, Copper oxide nanoparticles are highly toxic: a comparison between metal oxide nanoparticles and carbon nanotubes, Chem. Res. Toxicol., 2008, 21, 1726–1732 Search PubMed.
- C. Carlson, S. M. Hussain, A. M. Schrand, L. K. Braydich-Stolle, K. L. Hess, R. L. Jones and J. J. Schlager, Unique cellular interaction of silver nanoparticles: size-dependent generation of reactive oxygen species, J. Phys. Chem. B, 2008, 112, 13608–13619 CrossRef CAS PubMed.
- C. Gunawan, W. Y. Teoh, C. P. Marquis and R. Amal, Cytotoxic origin of copper(II) oxide nanoparticles: comparative studies with micron-sized particles, leachate, and metal salts, ACS Nano, 2011, 5, 7214–7225 CrossRef CAS PubMed.
- L. Denluck, F. Wu, L. E. Crandon, B. J. Harper and S. L. Harper, Reactive oxygen species generation is likely a driver of copper based nanomaterial toxicity, Environ. Sci.: Nano, 2018, 5, 1473–1481 RSC.
- S. D. Kim, H. Ma, H. E. Allen and D. K. Cha, Influence of dissolved organic matter on the toxicity of copper to Ceriodaphnia dubia: effect of complexation kinetics, Environ. Toxicol. Chem., 1999, 18, 2433–2437 CrossRef CAS.
- L. M. Gilbertson, E. M. Albalghiti, Z. S. Fishman, F. o. Perreault, C. Corredor, J. D. Posner, M. Elimelech, L. D. Pfefferle and J. B. Zimmerman, Shape-dependent surface reactivity and antimicrobial activity of nano-cupric oxide, Environ. Sci. Technol., 2016, 50, 3975–3984 CrossRef CAS PubMed.
- S. Gonzalo, V. Llaneza, G. Pulido-Reyes, F. Fernández-Piñas, J. C. Bonzongo, F. Leganes, R. Rosal, E. García-Calvo and I. Rodea-Palomares, A colloidal singularity reveals the crucial role of colloidal stability for nanomaterials in-vitro toxicity testing: nZVI-microalgae colloidal system as a case study, PLoS One, 2014, 9, e109645 CrossRef PubMed.
- Y. Xiao, W. J. Peijnenburg, G. Chen and M. G. Vijver, Impact of water chemistry on the particle-specific toxicity of copper nanoparticles to Daphnia magna, Sci. Total Environ., 2018, 610, 1329–1335 CrossRef PubMed.
Footnote |
† Electronic supplementary information (ESI) available. See DOI: 10.1039/c9en01026b |
|
This journal is © The Royal Society of Chemistry 2020 |