Role of nano-biochar in attenuating the allelopathic effect from Imperata cylindrica on rice seedlings†
Received
23rd July 2019
, Accepted 30th November 2019
First published on 2nd December 2019
Abstract
It is reported that Imperata cylindrica, a successful invasive plant in North America, has covered many parts of the United States, especially the southeastern region which is the main rice growing region. The invasion of I. cylindrica could threaten rice production. In this study, we hypothesize that biochar nanoparticles (nano-BC) can reduce the stress from the root exudates of I. cylindrica. Ferulic acid (FA) is a major chemical of I. cylindrica root exudates, and we selected its analog, salicylic acid, as an experimental control. We found that nano-BC has detoxification effects for rice seedlings under FA treatments. Rice seedlings grow better and the phenotype of rice seedlings recovers after nano-BC application under FA treatments. In comparison to the seedlings treated with FA, the biomass of the seedlings increases 344% and 435% after adding 200 mg kg−1 and 400 mg kg−1 of nano-BC to the FA treatment in the pot experiment, respectively. Superoxide dismutase activities (antioxidant system index) and malondialdehyde concentration (lipid peroxidation marker) decline after the addition of nano-BC. Additionally, the rice growth is better after the addition of nano-BC in the FA treatment. The total chlorophyll concentration displays a recovery trend, and the recovery rates are 55% and 96% after 400 mg L−1 and 400 mg kg−1 of nano-BC are added to the FA treatments in the hydroponic and pot experiments, respectively. The expression of three salicylic acid-related genes also demonstrates recovery in the treatment with nano-BC and FA. Therefore, nano-BC is a potential material that can be used to protect rice production from the stress of I. cylindrica invasion.
Environmental significance
The spread of invasion plant species has caused up to 120 billion dollars loss per year in the USA from 2005. Imperata cylindrica (L.) Beauv. is a widespread invasive species all over the world. In America, I. cylindrica survives best in the southeast (and, according to a 2003 survey, has overtaken more acreage in that region than notorious kudzu). According to a United States Department of Agriculture (USDA) report, the south part is the rice production area. With the wide spread of I. cylindrica, a method should be developed to reduce the allelopathy effects and protect rice growth from the stress of I. cylindrical invasion. This study is the first attempt to apply nano-BC to protect the native environment and native species, and reduce the stress from invasive species. In our results, we found that the application of nano-BC could promote rice seedling growth, enhance biomass, root length and chlorophyll concentration, reduce the oxidative stress and lipid peroxidation, and decrease the negative gene expressions at the molecular level under invasion species root exudate treatment. The results shed new light on the impact of addition of nano-BC on the invasion plant control, and the new application of nano-BC in environmental science and agriculture.
|
Introduction
The spread of invasive plant species has caused up to $120 billion in losses per year in the United States since 2005.1 The expansion of invasive plant species could have a negative impact on local soil nutrient contents, water balance, and biodiversity;2,3 and it could also inhibit the growth of native plants.4,5Imperata cylindrica (L.) Beauv. is a widespread invasive species in North America, Northern Asia, Europe, and Africa. It is listed as an invasive weed in some areas.6 In the United States, it survives best in the southeast (and, according to a 2003 survey, it has overtaken more acreage in that region than the notorious kudzu), but it has been reported to exist as far north as West Virginia and Oregon.7 In Florida, I. cylindrica is found in areas where the soil has been disturbed, such as roadsides, building sites, timber harvesting areas, and borrow pits.8 According to the United States Department of Agriculture (USDA), the southern region of the country is the rice production area. I. cylindrica has already spread in and invaded some rice culture regions, such as Papua New Guinea and Indonesia,9,10 and has caused much economy loss in local areas. With the widespread invasion of I. cylindrica, it is urgent to find an effective method to reduce its allelopathic effects on rice growth.
In terms of the new weapon hypothesis (NWH) theory, the allelochemicals released from invasive plants are the major factor for the decrease in native plant growth in the environment.11,12 In our previous study, we found that the allelochemicals from Bidens pilosa root exudates could inhibit the growth of native Pteris multifida.13 It was reported that the release of allyl isothiocyanate in the soil from successful invaders, Alliaria petiolata, could reduce fungal growth and fern spore germination in the Trillium Trail Wildflower Reserve forest.14 Therefore, decreasing the concentrations of allelochemicals in soil would be a useful way to control the damage that invasive species cause to native plants. Ferulic acid (FA) was reported to be a major root exudate from I. cylindrica,15 and, in this study, we employed this allelochemical in our Oryza sativa culture experiments. O. sativa has been cultivated in the United States for more than 400 years; its harvest area was recorded to be over 2.7 million ha in the south in 2013, and its production exceeded 19.9 billion pounds in 2012. The O. sativa cultivation area includes the I. cylindrica invasion region (Fig. 1). Thus, in this study, we discuss the negative effects caused by I. cylindrica allelochemical and how to reduce this effect via biochar nanoparticles.
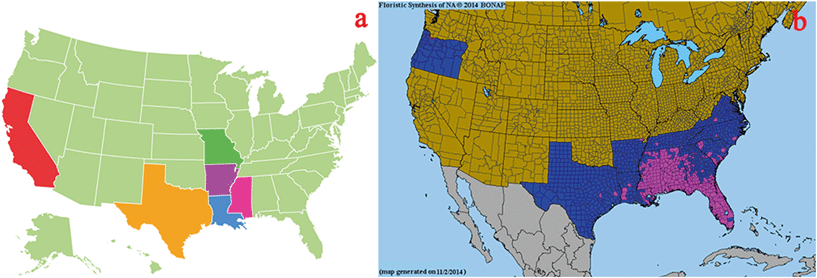 |
| Fig. 1 The rice culture region (a) and the spread area of Imperata cylindrica (L.) Beauv. (b) in the USA. Note: the USA rice culture regions are Arkansas (purple), California (red), Louisiana (blue), Mississippi (pink), Missouri (dark green) and Texas (orange) (USA rice, 2018). The spread area of Imperata cylindrica (L.) Beauv. is colored in purple, and the extreme area is colored in pink (Biota of North America Program (BONAP), 2014). | |
Due to their large specific surface area, surface hydrophobicity, and micro-porosity, biochar nanoparticles (NPs) have a higher sorption capacity for a variety of contaminants, such as heavy metals, herbicides, polychlorinated biphenyls (PCBs), and polycyclic aromatic hydrocarbons (PAHs), and they could be used to neutralize acidic soils due to their high pH and alkalinity.16,17 Also, nanocarbon materials, such as black carbon, activated carbon, and engineered carbon nanoparticles, have been found to adsorb dissolved natural organic matter in the environment.18–20 It was found that nano Fe2O3 particles have higher capacities for ofloxacin and norfloxacin in the soil when their surface is covered with humic acid.21 Single-walled carbon nanotubes have demonstrated good performance in the sorption capacity of phenoxy acid herbicides (a type of organic phenoxy systemic herbicide) in water.22 Biochar particles have characteristics that are similar to other carbon materials.23,24 Moreover, in the present study, we discuss the plant protection capability of nano-BC in agriculture. The application of nano-BC is an important topic in the fields of environmental science and agriculture.
We hypothesized that the adsorption of allelochemicals through nano-BC could reduce the allelopathic effects from I. cylindrica on O. sativa growth and development. This study aimed to: (1) reduce the allelopathic negative effects on O. sativa caused by I. cylindrica in agriculture, (2) propose a new method to protect native species, and (3) verify, for the first time, the biological safety of releasing nano-BC into an agricultural environment.
Materials and methods
Plant preparation and experimental design
Oryza sativa L. cv. ‘Early Wright’ seeds were chosen for this study. This species is a popular variety of rice cultivated in the state of Louisiana. The seeds were surface-sterilized with 3% H2O2 for 5 min, and then germinated in deionized water in the dark at 37 °C for three days and transferred to a net floating on deionized water for another three days. The seedlings were transferred to a greenhouse with a light intensity of 280 μmol−2 s−1 and with a humidity of 65%. The temperature was maintained at 28 °C during the day and 25 °C during the night with a 12 h photoperiod. The plants were grown in a 0.5 strength Kimura B nutrient solution (details about the compositions are presented in Table S1†). The pH value of the nutrient solution was adjusted to 5.6 with 0.1 M NaOH, and the solution was renewed every three days until the 20th day. The biochar and nano-BC preparation and properties are listed in the ESI† (Tables S3 and S4). And we chose the I. cylindrica half maximal inhibitory concentration (IC50) as the maximum ferulic acid (FA) treatment concentration in this study.15
Hydroponic experiment.
The 20 day seedlings were transferred to 200 mL culture bottles. We prepared 285 mg L−1 (2.06 mM) salicylic acid (SA), 400 mg L−1 (2.06 mM) FA, 400 mg L−1 nano-biochar, 400 mg L−1 FA + 400 mg L−1 nano-BC, and 400 mg L−1 FA + 200 mg L−1 nano-biochar with the nutrient solution as the treatments for a series of experiments. The half strength Kimura B nutrient solution was set as the control (Table S1†). The nutrient solution was renewed every three days. Each treatment was repeated three times. And we adjusted the solution pH at 5.6 at every check point.
Pot experiment.
A pot experiment was conducted with three treatments (400 mg kg−1 FA, 400 mg kg−1 FA + 400 mg kg−1 nano-BC, and 400 mg kg−1 FA + 200 mg kg−1 nano-BC) and the control. Each treatment was repeated three times. The pots (6 cm bottom diameter and 8 cm height, containing 250 g soil.) were watered with the treated solution every six days under controlled conditions (day/night temperature of 25/20 °C and relative humidity of 65%) in the greenhouse of the College of Natural Science (CNS), University of Massachusetts, Amherst. Forty pots were used in the experiments. All-purpose potting soil (ProMix BX, Premier Hort Tech, Quakertown, PA, USA) was added to the pots. At the end of the experiment, the roots of the plants were washed with deionized water for biomass measurement and observation. Details about the nano-BC particle preparation are presented in the ESI.† The purity of the SA used in this study is over 99% (Acros Organics, Fisher Scientific, USA) and FA purity is over 98% (L'eternel World, LLC, USA).
Biomass measurement
Fresh O. sativa samples of the four treatments were harvested at the 6th, 12th, 18th, 24th, and 30th day, respectively, and the initial O. sativa total biomass (M0) was recorded at day 0. An electronic scale was used to measure the rice total biomass (MT). The formula below was used to calculate the relative biomass change (%), using the methods described by Shen (2019).25
Relative biomass change(%) = (MT − M0)/M0 × 100% |
Total chlorophyll concentration, superoxidase dismutase (SOD) and malondialdehyde (MDA)
The total chlorophyll concentration was calculated via the sum of chlorophyll a and chlorophyll b, using the method described by Moran (1982).26 The chlorophyll a and chlorophyll b concentrations of the rice seedlings in all treatments are listed in the ESI† (Fig. S3).
Enzyme extraction was conducted based on the method reported by Bradford (1976).27 The fresh O. sativa leaf samples of each treatment were pooled and ground into fine powder using liquid nitrogen. Then, 5 g of this powder was homogenized in 5 mL 0.1 M phosphate buffer (pH 6.5), containing 14 mM dithioerythritol (DTE) and 1 mM ethylenediaminetetraacetic acid (EDTA). The extraction was prepared by centrifugation at 10
000g for 30 min. The supernatant was used to determine the SOD activity.
The SOD [EC 1.15.1.1] activity was determined using the method by Lee et al. (2001).28 The reaction mixture (4 mL) consisted of 0.8 mL distilled water, 0.1 mL extract, 3.1 mL phosphate buffer (pH 7.5), 80 mg methionine, 4 mg nitroblue tetrazolium (NBT), 4 mg Na2EDTA, and 0.4 mg riboflavin. Then, the mixture was set under 4000 lux light for 10 min. A control was used without an enzyme in the reaction series, and the mixture, which was kept in the dark, served as a blank. One unit of enzyme activity was defined as the amount of SOD required to cause 50% inhibition of the p-nitro blue tetrazolium chloride reduction rate at 560 nm.
The MDA concentration was determined using the method described by Kosugi and Kikugawa (1985).29 In brief, each group of fresh rice leaf samples of 0.1 g was homogenized with 5 mL phosphate buffer (pH 7.0). The homogenate was centrifuged at 960g for 20 min, and the supernatant was used for lipid peroxidation analysis. Then, 3 milliliters of 0.1% (w/v) trichloroacetic acid (TCA), composed of 3 mL of 0.5% (w/v) thiobarbituric acid (TBA), were added to 1 mL of the prepared supernatant. The mixture was set in boiling water for 30 min, and then cooled quickly in an ice bath. The cooled tubes were centrifuged at 10
000g for 30 min, and the absorbance of the supernatant was observed at 532 nm. The nonspecific absorbance value at 600 nm was monitored and subtracted. The MDA concentration was calculated using the extinction coefficient of 155 mM−1 cm−1.
Gene expression
To better understand the accumulation of FA in O. sativa, we applied SA, which is an analog to FA, in the hydroponic and pot experiments. We selected three SA-related genes (OsNCED1, OsABA8ox1, and OsPR10a), and analyzed their expression when SA, FA, and nano-biochar were added in the experiments. Total RNA was extracted from the leaf samples using the Trizol method.30 Genomic DNA was degraded using DNase I (Takara Bio Inc., Shiga, Japan). Polymerase chain reaction (PCR) was performed using SYBR Premix Ex TaqII (Tli RNaseH Plus) (TaKaRa, Japan). Once the appropriate DNA fragments were generated by RT-PCR, quantitative RT-PCR (qRT-PCR) amplification and detection were performed using the Gene Amp 5700 sequence detection system (PE Biosystems, Foster City, CA, USA). The primers used for RT-PCR are listed in Table S2.† The experiments were repeated three times for the analysis.
Statistical analysis
The experiments were performed with four biological replicates per treatment. Statistical analyses of the data were performed using analysis of variance (ANOVA) with SPSS 21.0 statistical software (IBM, USA). The means were compared using the least significant difference (LSD) test and Duncan's new multiple range test. Statistical significance was set at the p < 0.05 level. The p < 0.05 level indicates the range of majority of significant changes among the data comparison in this study.
Results
The phenotypes and biomass changes
Hydroponic experiment is a rapid and effective way to detect the plant growth response to soluble compounds.31,32 We found that the number of leaves for the O. sativa seedlings was one less in the treatments with 285 mg L−1 SA, 400 mg L−1 FA, and 400 mg L−1 FA + 200 mg L−1 nano-BC than the leaf number in the control, 400 mg L−1 nano-BC and 400 mg L−1 FA + 400 mg L−1 nano-BC (Fig. 2a). The heights of the control, 400 mg L−1 nano-BC, and 400 mg L−1 FA + 400 mg L−1 nano-BC seedling samples were higher than the heights of the samples in the three other treatments. We also found that the root lengths under the SA and FA treatments were significantly shorter than the ones in the other four treatments (p < 0.05) (Fig. S4†).
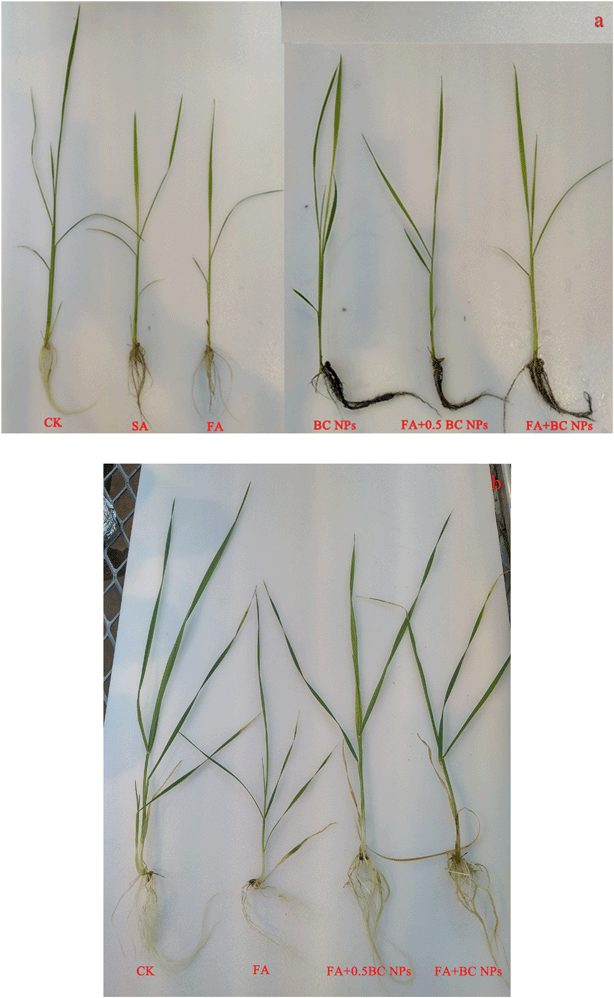 |
| Fig. 2 Phenotypes and growth responses in rice under six treatments of the hydroponic experiment (a), and under four treatments of the pot experiment (b) after 30 days. Note: (a) CK, control; SA, 284.53 mg L−1 (2.06 mM) salicylic acid; FA, 400.00 mg L−1 (2.06 mM) ferulic acid; BC NPs, 400.00 mg L−1 nano-BC; FA + 0.5 BC NPs, 400.00 mg L−1 FA + 200.00 mg L−1 nano-BC; FA + BC NPs, 400.00 mg L−1 FA + 400.00 mg L−1 nano-BC; (b) CK, control; FA, 400.00 mg kg−1 ferulic acid; FA + 0.5 nano-BC, 400.00 mg kg−1 FA + 200.00 mg kg−1 nano-BC; FA + nano-BC, 400.00 mg kg−1 FA + 400.00 mg kg−1 nano-BC. | |
To verify the adsorption effects of nano-BC in soil, we performed the pot experiment. The seedling growth in the sample treated with FA was significantly weakened in comparison to the samples that underwent the three other treatments; and the seedling heights were shorter in the two nano-biochar-added treatments than in the control, as were the leaf lengths (Fig. 2b). In addition, after 400 mg kg−1 nano-BC was added to FA treatment, the growth of the seedlings and the root lengths turned normal (Fig. 2b). The root length was significantly shorter in the 400 mg kg−1 nano-BC-added FA treatment than that in the control and the treatment with the addition of nano-BC.
We then compared the seedling biomass changes in the hydroponic and pot experiments (Fig. 2b). We found that the growth of the seedlings in the control significantly increased in 30 days (p < 0.05), with the highest rate of 343% at day 30; the growth in the nano-BC-added group was higher than with the growth in the nano-BC-added and FA treatments, and all three treatments showed a growth increase trend during the experimental period, obtaining the maximum rates of 256%, 188%, and 118%, respectively. The seedling biomass of the SA and FA treatments displayed a growth decrease trend, with a significant (p < 0.05) minimum of −38% and −1%, respectively, at day 30 (Fig. 3a). We then analyzed the seedling biomass changes in the pot experiment. We found a similar trend in the seedling biomass change, and the control and the nano-BC and FA treatment exhibited an increasing trend. Specifically, the biomass increase rates of the control and the two nano-BC-added treatments significantly increased (p < 0.05) at every check point, and the seedling biomass increase rate in the control was higher than the rate of the two nano-BC-added treatments, reaching 545%, 435%, and 344%, respectively, at day 30. The seedling biomass change rate of the FA treatment was stable for the first 18 days; it then decreased significantly (p < 0.05) (Fig. 3b).
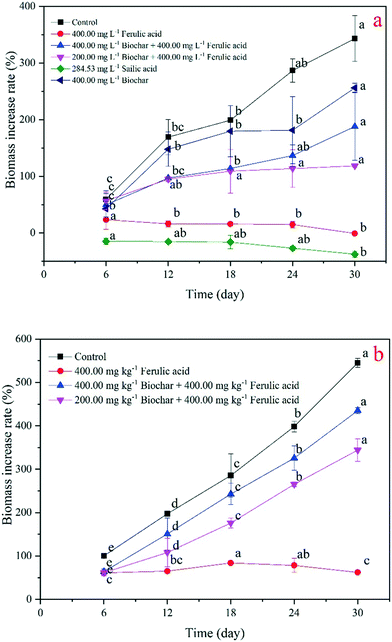 |
| Fig. 3 The rice biomass increase rates (%) under salicylic acid, ferulic acid and nano-biochar added treatments in the hydroponic experiment (a); under ferulic acid and nano-biochar added treatments in the pot experiment (b) after 30 days. Bars with different letters are significantly different (one-way ANOVA with Duncan's test). | |
Total chlorophyll concentration, SOD activity and MDA concentration changes
The chlorophyll concentrations of the rice leaf are a significant indicator of plant recovery from environmental stress.25 In our study, we found that the exogenous addition of nano-BC could increase the chlorophyll concentration under the FA treatments in the hydroponic and pot experiments. Specifically, the total chlorophyll concentrations of the rice seedlings in the 200 and 400 mg kg−1 nano-BC-added FA treatments were, respectively, 9% and 4% lower than the total chlorophyll concentrations in the control at day 30; they were 52% and 55% higher than the concentrations in the FA treatment in the hydroponic experiment, respectively (Fig. 4b). At day 30 in the pot experiment, the total chlorophyll concentrations of the rice seedlings in the 200 and 400 mg L−1 nano-BC-added FA treatments were 1.80-fold and 1.96-fold that in the FA treatment (Fig. 4b).
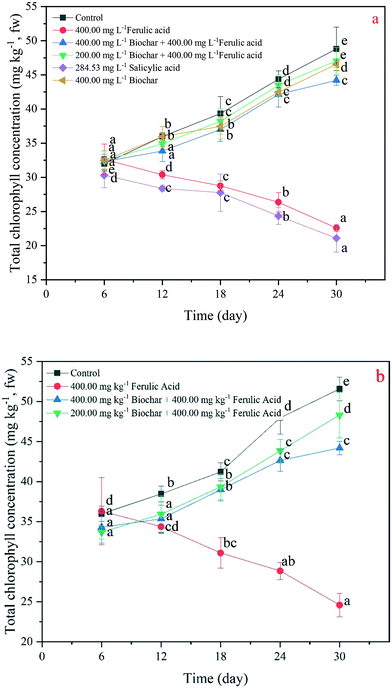 |
| Fig. 4 Total chlorophyll concentration (mg kg−1) under salicylic acid, ferulic acid and nano-biochar added treatments in the hydroponic experiment (a); under ferulic acid and the nano-biochar added treatments in the pot experiment (b) after 30 days. Bars with different letters are significantly different (one-way ANOVA with Duncan's test). | |
SOD is a kind of antioxidant enzyme that is the first antioxidant to transform a superoxide radical into either ordinary molecular oxygen or H2O2 under oxidative stress in plant cells,33 and it is often used to describe the stress strength in plant cells.34,35 Our results demonstrate that the SOD activities of all the treatments with added exogenous material increased in both the hydroponic and pot experiments, but differences in means between plants in different pots when compared to hydroponics are not great. Specifically, the SOD activity of the SA treatment demonstrated the most significant increase (p < 0.05) among all the treatments, and the activity value reached 122.87 U g−1 (Fig. 5a). Moreover, the SOD activities of the FA treatment from the two experiments were significantly higher (p < 0.05) than the activities of the 200 and 400 mg L−1 nano-BC-added FA treatments; the increased values were 34.03 U g−1 and 31.38 U g−1 and 52.46 U g−1 and 44.85 U g−1 in the hydroponic and pot experiments, respectively (Fig. 5a and b).
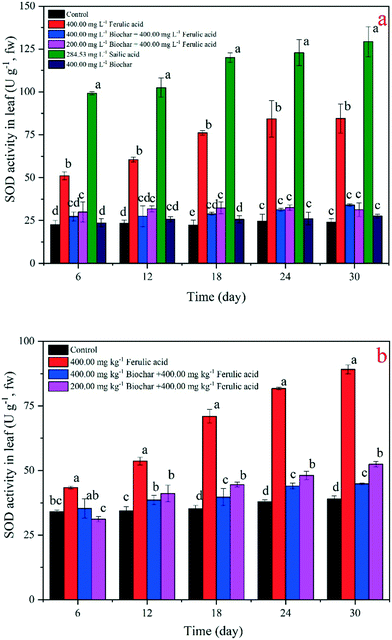 |
| Fig. 5 SOD activity (U g−1) of rice leaves under salicylic acid, ferulic acid and nano-biochar added treatments in the hydroponic experiment (a); under ferulic acid and nano-biochar added treatments in the pot experiment (b) after 30 days. Bars with different letters are significantly different (one-way ANOVA with Duncan's test). | |
MDA occurs naturally, and it is a marker for oxidative stress and toxicity in plant cells.36 In the present study, we applied this index to analyze the toxicity change when nano-BC was added to the FA treatments. Our results showed that the MDA concentrations all increased when the exogenous materials were added. In the SA-treated rice seedlings, the MDA concentration was the highest at day 30 (41.34 nmol g−1). The MDA concentrations of the two nano-BC-added groups decreased in the FA-added groups in the hydroponic and pot experiments; the values of the 200 and 400 mg L−1 nano-BC-added treatments decreased to 12.36 nmol g−1, 14.48 nmol g−1, and 14.54, 17.11 nmol g−1, respectively. However, the total MDA concentration still increased significantly (p < 0.05) for the FA-treated group in the experimental periods. We also found that the SA treatment had a significantly stronger effect on the SOD activity and MDA concentration than the FA treatment; the difference in the rates was 44.80 U g−1 and 10.68 nmol g−1 in the hydroponic experiment, respectively (Fig. 5a and 6a). Changes in the SOD activity and MDA concentration were similar in the 400 mg kg−1 nano-BC-treated rice seedlings and the control; no significant changes in the values were observed in the pot experiment (Fig. 5b and 6b).
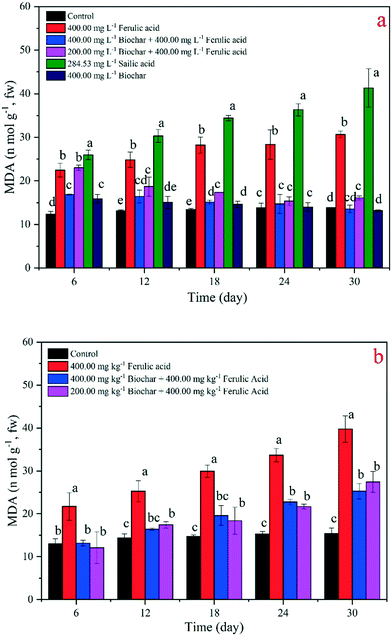 |
| Fig. 6 The MDA concentrations (nmol g−1) of rice leaves under salicylic acid, ferulic acid and the nano-biochar added treatments in the hydroponic experiment (a); under ferulic acid and the nano-biochar added treatments in the pot experiment (b) after 30 days (fw, fresh weight). Bars with different letters are significantly different (one-way ANOVA with Duncan's test). | |
Related SA gene expression
At day 30, we harvested the rice seedlings and tested the SA gene expressions of the leaves in both the hydroponic and pot experiments using qRT-PCR. The expressions of three SA-related genes in the SA and FA treatments were significantly (p < 0.05) up-regulated relative to the other treatments (Fig. 7). In the hydroponic experiment (Fig. 7a), the up-regulation of the OsNCED1, OsABA8ox1, and OsPR10a expressions in the rice leaves of the 285 mg L−1 SA and 400 mg L−1 FA treatments were high, but the gene expressions decreased after adding 400 and 200 mg L−1 nano-BC to the FA treatments. The expression of these three genes in the pot experiment (Fig. 7b) showed a similar trend to the results in the hydroponic experiment, but the up-regulation of the three genes was lower. Moreover, in the nano-BC-added treatment, the expression of these three genes was the same as the control group (no significant up-regulation) in both the hydroponic and pot experiments.
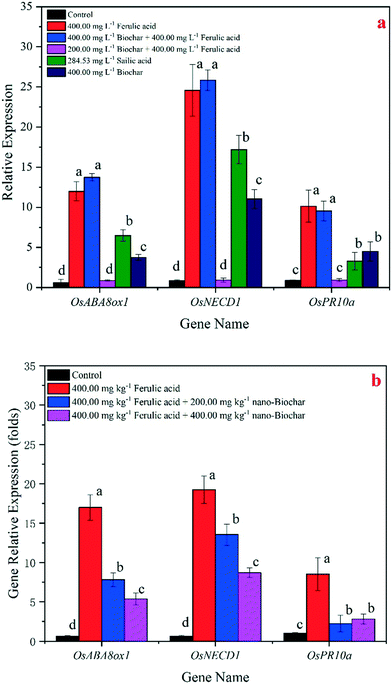 |
| Fig. 7 The expression of OsABA8ox1, OsNCED1 and OsPR10a in rice leaf under salicylic acid, ferulic acid and nano-biochar added treatments in the hydroponic experiment (a); under ferulic acid and nano-biochar added treatments in the pot experiment (b) after 30 days. Bars with different letters are significantly different (one-way ANOVA with Duncan's test). | |
Discussion
Detoxification effects caused by nano-BC
It is known that invasive plant root exudates increase toxicity to other plants, then control native plant growth and damage the antioxidant system.13 After the addition of nano-BC, the growth of rice seedlings was better than the growth in the SA and FA treatments in the hydroponic experiment and the FA treatment in the pot experiment (Fig. 2 and 3 and S4†). It has been reported that softwood chip biochar can reduce the leaching risks of phenanthrene, sulfamethazine, and isoproturon in soil, and the addition of biochar could enhance the chemical's sorption coefficient.37 After rice straw biochar was added, the rice increased its heavy metal resistance, and the activities of SOD, peroxidase, and catalase recovered from cadmium (Cd) pollution.38 In our results, this nano-BC (corn biochar – 350 °C treated) could adsorb over 77.50% FA in solution (Fig. S2†), and the residual FA concentration was much lower than that in the 400 mg L−1 FA solution. In the hydroponic experiment (Table S5†), we observed that the FA concentration decreased after 30 days of nano-BC addition, and our results indicate that the plant resistance increased and more FA remained in the solution. This is an important reason why the seedlings recovered from the stress.
Among the antioxidants in the antioxidant systems, SOD is the most effective intracellular enzymatic antioxidant system (AOS).39 It is well established that various environmental stresses often lead to the increased generation of reactive oxygen species (ROS), where SOD has been proposed to be important in plant stress tolerance and to provide the first line of defense against the toxic effects of elevated levels of ROS.33 Thus, SOD can be used as an index of AOS response under environmental stress;25 that is why we chose SOD in this study. It has been reported that an increase in SOD activity is an indication of stress strengthening in plants.40,41 We observed that the SOD activities of the nano-BC-added groups decreased in comparison to the groups that were only treated with FA and SA in both the hydroponic and pot experiments. With an increase in nano-BC-added concentration, the SOD activity further decreased, and the recovery was better (Fig. 5). In the pot experiment, we found that the SOD activities were significantly (p < 0.05) lower when the nano-BC concentration increased (Fig. 5b). Therefore, lower SOD activity means that the stress and toxicity caused by FA and SA become weak when nano-BC was added to the FA and SA treatments.
MDA is the final product of polyunsaturated fatty acid peroxidation in the cell lipid peroxidation process of environmental stress.42,43 At the same time, MDA damages DNA, forming adducts of deoxyguanosine and deoxyadenosine.44 It has been suggested that an increase in the MDA concentration would lead to cell membrane system damage and carcinogenesis in plants. We found that the MDA concentrations in rice seedlings were significantly higher (p < 0.05) in the FA- and SA-treated groups than any of the other treatments. When combined with the rice seedling phenotype changes (Fig. 2), the rice seedlings in the higher MDA concentration groups showed negative growth, and their growth is weaker than that in the control and nano-BC-added groups. The seedlings in all the nano-BC added groups grew well, and they underwent the same development stages as the control; that is, they all had the fourth and the fifth leaf at the 30th day. Preliminarily, it is inferred that the addition of nano-BC could reduce the stress from SA and FA, and the AOS would be recovered and the lipid peroxidation process would be weakened. Accordingly, we suggest that nano-BC has a detoxification effect in rice seedlings under SA and FA treatments.
Rice growth safety after the addition of nano-BC
In general, native species would be overwhelmed by invasive plants after being invaded, and there would be no space for native plant survival.45 Recently, it has been reported that biochar has negative effects on plant growth.46 It was reported that 0.3 g mL−1 rice straw biochar would significantly (p < 0.05) reduce the germination rate of corn, wheat and rice seeds.47 Moreover, one study found that wheat chaff biochar particles would inhibit wheat seed germination and seedling growth.48 The percentage of Lepidium sativum seed germination was significantly reduced under stress of biochar particles.49 Our results support the findings reported in previous research studies: nano-BC could inhibit rice seedling growth. Specifically, we found that the height and the biomass of the rice seedlings that were only treated with the addition of nano-BC were lower than those in the control (Fig. 2a), and the biomass was reduced significantly (p < 0.05) (Fig. 3) in the group that was only treated with the addition of 400 mg L−1 nano-BC in the hydroponic experiment at day 30. It is suggested that, while nano-BC still has toxicity to rice seedlings, the rice seedlings grow better and their biomass increases significantly (p < 0.05) in the FA nano-BC-added groups in comparison to the groups that only underwent the SA and FA treatments in the hydroponic and pot experiments. Thus, it can be inferred that the addition of nano-BC could reduce the stress from FA and promote rice seedling growth and development under allelochemical treatments.
Changes in the chlorophyll concentration are an important indicator of environmental response in plants, and the concentration becomes decreased when plants are under environmental stress.50 The leaf color and chlorophyll concentrations are also important for plant safety and protection under environmental stress.49,50 It has been reported that tomato chlorophyll concentrations increase after the addition of 2.5 mM Na2SiO3 under salt stress,51 and the exogenous addition of melatonin could alter the nano-ZnO stress in wheat seedlings, especially the recovery of the chlorophyll concentration.52 Thus, the recovery of the chlorophyll concentration could also offer a new insight into improving plant resistance under environmental stress. In our study, the leaf color turned green after the addition of nano-BC (Fig. 2 and S4†), and we also recorded the increase in the chlorophyll concentrations after the addition of nano-BC under the FA treatments. Thus, nano-BC could protect rice growth. It is suggested that the addition of exogenous nano-BC could improve the chlorophyll concentration in crops in invasive species-growing agriculture and under conditions of environmental stress.
We also applied three SA-related genes associated with plant growth to test the response of rice seedlings at the molecular level after the addition of nano-BC. We found that OsNCED1 could regulate rice growth under cold, salt, and leaf senescence stress.53–55OsABA8ox1 encodes ABA 8′-hydroxylase in rice, and its over-expression also controls rice shoot growth.56OsPR10a (pathogenesis-related protein 10a) is a promoter of SA biosynthesis when rice is under stress, and it is also related to leaf senescence.57 Our results (Fig. 7) demonstrated that the expression of these three genes significantly decreased (p < 0.05) after the addition of nano-BC in the FA treatment, and the gene expressions of the seedlings in the 400 mg L−1 nano-BC-added groups were also lower than those in the 200 mg L−1 nano-BC-added groups and the FA treatment in the hydroponic experiment (Fig. 7a). The same changes were observed in the pot experiment; the expression of these three genes in the nano-BC-added groups were significantly lower (p < 0.05) than those in the FA treatments (Fig. 7b). Based on the functions of the three genes, over-expression of OsNCED1, OsABA8ox1, and OsPR10a led to weaker and shorter rice seedlings under the SA and FA treatments in the hydroponic experiment. The same changes in the rice seedlings occurred under the FA treatment in the pot experiment. However, after the addition of nano-BC, the expression of these three genes decreased, and the gene expressions in the two nano-BC-added groups were significantly lower than those in the SA and FA treatments in the hydroponic experiment (Fig. 7a). The rate of the decreased expression of the three genes was higher in the pot experiment (Fig. 7b). Recovery was also observed in the phenotypes of rice seedlings after the addition of nano-BC. In the nano-BC-added groups, the expression of the three genes is still higher than that in the control. However, the growth of rice seedlings also recovers from the FA treatments, although the biomass and rice growth conditions are not consistent with the control. Thus, at the molecular and phenotype levels, it is suggested that rice seedlings can recover from the stress caused by FA after the addition of nano-BC, and the seedlings could continue growing under the FA treatment.
Conclusions
This study attempted to apply nano-BC to protect native environments and native species, and to reduce the stress caused by invasive species. Application of nano-BC can promote rice seedling growth, enhance biomass, increase the root length and the chlorophyll concentration, reduce oxidative stress and lipid peroxidation, and decrease the negative gene expressions at the molecular level under invasive species root exudate treatment. Therefore, our study provides a new potential method for controlling the spread of invasive species in the environment and offers a new strategy for rice production protection under the threat from I. cylindrica.
Conflicts of interest
There are no conflicts to declare.
Acknowledgements
This work was supported by the National Natural Science Foundation of China (31770546, 31370521), the Priority Academic Program Development of Jiangsu Higher Education Institutions (PAPD), the Graduate Student Training Innovation Project of Jiangsu Province (KYZZ16_0378) and USDA-NIFA Hatch program/UMass CAFE (MAS 00549). Yu Shen and Haiyan Tang thank the China Scholarship Council (CSC) for the financial support to study at the University of Massachusetts, Amherst.
References
- D. Pimentel, R. Zuniga and D. Morrison, Update on the environmental and economic costs associated with alien-invasive species in the United States, Ecol. Econ., 2005, 52, 273–288 CrossRef.
- R. Aerts, M. Ewald, M. Nicolas, J. Piat, S. Skowronek, J. Lenoir, T. Hattab, C. X. Garzon-Lopez, H. Feilhauer, S. Schmidtlein, D. Rocchini, G. Decocq, B. Somers, R. Van De Kerchove, K. Denef and O. Honnay, Invasion by the Alien Tree Prunus serotina Alters Ecosystem Functions in a Temperate Deciduous Forest, Front. Plant Sci., 2017, 8, 179 Search PubMed.
- C. Antunes, A. J. Pereira, P. Fernandes, M. Ramos, L. Ascensão, O. Correia and C. Máguas, Understanding plant drought resistance in a Mediterranean coastal sand dune ecosystem: differences between native and exotic invasive species, J. Plant Ecol., 2018, 11, 26–38 CrossRef.
- K. M. Zhang, Y. Shen, Y. M. Fang, P. C. Bhowmik and Y. Li, Effects of Bidens pilosa shoot parts on chlorophyll fluorescence in Pteris multifida gametophyte, Allelopathy J., 2017, 42, 233–240 CrossRef.
- C. Morris, C. A. Call, T. A. Monaco, P. R. Grossl and S. A. Dewey, Evaluation of elemental allelopathy in Acroptilon repens (L.) DC. (Russian Knapweed), Plant Soil, 2006, 289, 279–288 CrossRef CAS.
-
D. Jaggi, M. Varun, S. Pagare, N. Tripathi, M. Rathore, R. Singh and B. Kumar, Invasive Alien Weed Species: A Threat to Plant Biodiversity, Cabi Publishing-C a B Int, Wallingford, 2017 Search PubMed.
-
USDA, Crop Production: 2012 Summary, National Agricultural Statistics Service, 2013 Search PubMed.
- R. D. Lucardi, L. E. Wallace and G. N. Ervin, Evaluating hybridization as a potential facilitator of successful cogongrass (Imperata cylindrica) invasion in Florida, USA, Biol. Invasions, 2014, 16, 2147–2161 CrossRef.
- G. Burkard, Sawah first! The cultural ecology of alang-alang in a rain forest margin community, J. Agr. Rural Dev. Trop., 2005, 106, 1–14 Search PubMed.
- R. Walter and B. R. Rao, Biochars influence sweet-potato yield and nutrient uptake in tropical Papua New Guinea, J. Plant Nutr. Soil Sci., 2015, 178, 393–400 CrossRef CAS.
- J. L. Hierro and R. M. Callaway, Allelopathy and exotic plant invasion, Plant Soil, 2003, 256, 29–39 CrossRef CAS.
- M. A. Rua, S. Nijjer, A. Johnson, W. E. Rogers and E. Siemann, Experimental approaches to test allelopathy: A case study using the invader Sapium sebiferum, Allelopathy J., 2008, 22, 1–13 Search PubMed.
- K. M. Zhang, Y. Shen, J. Yang, X. Miu, P. C. Bhowmik, X. Zhou, Y. M. Fang and B. S. Xing, The defense system for Bidens pilosa root exudate treatments in Pteris multifida gametophyte, Ecotoxicol. Environ. Saf., 2019, 173, 203–213 CrossRef CAS PubMed.
- A. Cantor, A. Hale, J. Aaron, M. B. Traw and S. Kalisz, Low allelochemical concentrations detected in garlic mustard-invaded forest soils inhibit fungal growth and AMF spore germination, Biol. Invasions, 2011, 13, 3015–3025 CrossRef.
- T. D. Xuan, T. Toyama, M. Fukuta, T. D. Khanh and S. Tawata, Chemical interaction in the invasiveness of cogongrass (Imperata cylindrica (L.) Beauv.), J. Agric. Food Chem., 2009, 57, 9448–9453 CrossRef CAS PubMed.
- D. Wang, W. Zhang, X. Hao and D. Zhou, Transport of biochar particles in saturated granular media: effects of pyrolysis temperature and particle size, Environ. Sci. Technol., 2013, 47, 821–828 CrossRef CAS PubMed.
- B. Gamiz, L. Cox, M. C. Hermosin, K. Spokas and R. Celis, Assessing the Effect of Organoclays and Biochar on the Fate of Abscisic Acid in Soil, J. Agric. Food Chem., 2017, 65, 29–38 CrossRef CAS PubMed.
- J. J. Pignatello, S. Kwon and Y. F. Lu, Effect of natural organic substances on the surface and adsorptive properties of environmental black carbon (char): Attenuation of surface activity by humic and fulvic acids, Environ. Sci. Technol., 2006, 40, 7757–7763 CrossRef CAS PubMed.
- J. E. Kilduff, T. Karanfil, Y. P. Chin and W. J. Weber, Adsorption of natural organic polyelectrolytes by activated carbon: A size-exclusion chromatography study, Environ. Sci. Technol., 1996, 30, 1336–1343 CrossRef CAS.
- H. Hyung, J. D. Fortner, J. B. Hughes and J. H. Kim, Natural organic matter stabilizes carbon nanotubes in the aqueous phase, Environ. Sci. Technol., 2007, 41, 179–184 CrossRef CAS PubMed.
- H. Peng, N. Liang, H. Li, F. Chen, D. Zhang, B. Pan and B. Xing, Contribution of coated humic acids calculated through their surface coverage on nano iron oxides for ofloxacin and norfloxacin sorption, Environ. Pollut., 2015, 204, 191–198 CrossRef CAS PubMed.
- A. De Martino, M. Iorio, B. Xing and R. Capasso, Removal of 4-chloro-2-methylphenoxyacetic acid from water by sorption on carbon nanotubes and metal oxide nanoparticles, RSC Adv., 2012, 2, 5693–5700 RSC.
- B. Chen, Z. Chen and S. Lv, A novel magnetic biochar efficiently sorbs organic pollutants and phosphate, Bioresour. Technol., 2011, 102, 716–723 CrossRef CAS PubMed.
- D. A. Laird, P. Fleming, D. D. Davis, R. Horton, B. Wang and D. L. Karlen, Impact of biochar amendments on the quality of a typical Midwestern agricultural soil, Geoderma, 2010, 158, 443–449 CrossRef CAS.
- Y. Shen, J. Li, S. Shi, R. Gu, X. Zhan and B. Xing, Application of carotenoid to alleviate the oxidative stress caused by phenanthrene in wheat, Environ. Sci. Pollut. Res., 2019, 26, 3593–3602 CrossRef CAS PubMed.
- R. Moran, Formulae for determination of chlorophyllous pigments extracted with N,N-dimethylformamide, Plant Physiol., 1982, 69, 1376–1381 CrossRef CAS PubMed.
- M. M. Bradford, A rapid and sensitive method for the quantification of microgram quantities of protein utilizing the principle of protein-dye binding, Anal. Biochem., 1976, 76, 248–253 CrossRef.
- D. H. Lee, Y. S. Kim and C. B. Lee, The inductive responses of the antioxidant enzymes by salt stress in the rice (Oryza sativa L.), J. Plant Physiol., 2001, 158, 737–745 CrossRef CAS.
- H. Kosugi and K. Kikugawa, Thiobarbituric acid reaction of aldehydes and oxidized lipids in glacial acetic-Acid, Lipids, 1985, 20, 915–921 CrossRef CAS.
- A. C. Da Luz, I. R. Pretti and M. D. P. Batitucci, Comparison of rna extraction methods for passiflora edulis sims leaves, Rev. Bras. Frutic., 2016, 38, 226–232 CrossRef.
- P. A. Herklotz, P. Gurung, B. Vanden Heuvel and C. A. Kinney, Uptake of human pharmaceuticals by plants grown under hydroponic conditions, Chemosphere, 2010, 78, 1416–1421 CrossRef CAS PubMed.
- J. Lopez-Bucio, O. M. de la Vega, A. Guevara-Garcia and L. Herrera-Estrella, Enhanced phosphorus uptake in transgenic tobacco plants that overproduce citrate, Nat. Biotechnol., 2000, 18, 450–453 CrossRef CAS PubMed.
- S. S. Gill and N. Tuteja, Reactive oxygen species and antioxidant machinery in abiotic stress tolerance in crop plants, Plant Physiol. Biochem., 2010, 48, 909–930 CrossRef CAS PubMed.
- S. H. Lee, N. Ahsan, K. W. Lee, D. H. Kim, D. G. Lee, S. S. Kwak, S. Y. Kwon, T. H. Kim and B. H. Lee, Simultaneous overexpression of both CuZn superoxide dismutase and ascorbate peroxidase in transgenic tall fescue plants confers increased tolerance to a wide range of abiotic stresses, J. Plant Physiol., 2007, 164, 1626–1638 CrossRef CAS PubMed.
- J. G. Scandalios, Oxygen Stress and Superoxide Dismutases, Plant Physiol., 1993, 101, 7–12 CrossRef CAS PubMed.
- D. Del Rio, A. J. Stewart and N. Pellegrini, A review of recent studies on malondialdehyde as toxic molecule and biological marker of oxidative
stress, Nutr., Metab. Cardiovasc. Dis., 2005, 15, 316–328 CrossRef PubMed.
- B. S. Trinh, D. Werner and B. J. Reid, Application of a full-scale wood gasification biochar as a soil improver to reduce organic pollutant leaching risks, J. Chem. Technol. Biotechnol., 2017, 92, 1928–1937 CrossRef CAS.
- Z. Zhang, J. Meng, S. Dang and W. Chen, Effect of Biochar on Relieving Cadmium Stress and Reducing Accumulation in Super japonica Rice, J. Integr. Agric., 2014, 13, 547–553 CrossRef CAS.
- F. J. Menvielle-Bourg, Superoxide dismutase (SOD), the powerful natural antioxidant, now available orally, Phytotherapie, 2005, 3, 1–4 CrossRef.
- M. Djanaguiraman, D. D. Devi, A. K. Shanker, J. A. Sheeba and U. Bangarusamy, Selenium – an antioxidative protectant in soybean during senescence, Plant Soil, 2005, 272, 77–86 CrossRef CAS.
- F. R. Cavalcanti, J. T. A. Oliveira, A. S. Martins-Miranda, R. A. Viegas and J. A. G. Silveira, Superoxide dismutase, catalase and peroxidase activities do not confer protection against oxidative damage in salt-stressed cowpea leaves, New Phytol., 2004, 163, 563–571 CrossRef CAS.
- T. Tounekti, A. M. Vadel, M. Oñate, H. Khemira and S. Munné-Bosch, Salt-induced oxidative stress in rosemary plants: Damage or protection?, Environ. Exp. Bot., 2011, 71, 298–305 CrossRef CAS.
- M. W. Davey, E. Stals, B. Panis, J. Keulemans and R. L. Swennen, High-throughput determination of malondialdehyde in plant tissues, Anal. Biochem., 2005, 347, 201–207 CrossRef CAS PubMed.
- L. J. Marnett, Lipid peroxidation-DNA damage by malondialdehyde, Mutat. Res., 1999, 424, 83–95 CrossRef CAS PubMed.
- J. D. Fridley, J. J. Stachowicz, S. Naeem, D. F. Sax, E. W. Seabloom, M. D. Smith, T. J. Stohlgren, D. Tilman and B. Von Holle, The invasion paradox: reconciling pattern and process in species invasions, Ecology, 2007, 88, 3–17 CrossRef CAS PubMed.
- D. Lin and B. Xing, Phytotoxicity of nanoparticles: inhibition of seed germination and root growth, Environ. Pollut., 2007, 150, 243–250 CrossRef CAS PubMed.
- S. Liao, B. Pan, H. Li, D. Zhang and B. Xing, Detecting free radicals in biochars and determining their ability to inhibit the germination and growth of corn, wheat and rice seedlings, Environ. Sci. Technol., 2014, 48, 8581–8587 CrossRef CAS PubMed.
- Z. M. Solaiman, D. V. Murphy and L. K. Abbott, Biochars influence seed germination and early growth of seedlings, Plant Soil, 2012, 353, 273–287 CrossRef CAS.
- W. Buss, M. C. Graham, J. G. Shepherd and O. Mašek, Risks and benefits of marginal biomass-derived biochars for plant growth, Sci. Total Environ., 2016, 569, 496–506 CrossRef PubMed.
- Y. Shen, J. Li, R. Gu, L. Yue, X. Zhan and B. Xing, Phenanthrene-triggered Chlorosis is caused by elevated Chlorophyll degradation and leaf moisture, Environ. Pollut., 2017, 220, 1311–1321 CrossRef CAS PubMed.
- K. Al-Aghabary, Z. Zhu and Q. H. Shi, Influence of silicon supply on chlorophyll content, chlorophyll fluorescence, and antioxidative enzyme activities in tomato plants under salt stress, J. Plant Nutr., 2004, 27, 2101–2115 CrossRef CAS.
- Z. Y. Zuo, L. Y. Sun, T. Y. Wang, P. Miao, X. C. Zhu, S. Q. Liu, F. B. Song, H. P. Mao and X. N. Li, Melatonin Improves the Photosynthetic Carbon Assimilation and Antioxidant Capacity in Wheat Exposed to Nano-ZnO Stress, Molecules, 2017, 22, 1727 CrossRef PubMed.
- H. C. Chen, W. H. Cheng, C. Y. Hong, Y. S. Chang and M. C. Chang, The transcription factor OsbHLH035 mediates seed germination and enables seedling recovery from salt stress through ABA-dependent and ABA-independent pathways, respectively, Rice, 2018, 11, 17 CrossRef PubMed.
- C. J. Mao, S. C. Lu, B. Lv, B. Zhang, J. B. Shen, J. M. He, L. Q. Luo, D. D. Xi, X. Chen and F. Ming, A Rice NAC Transcription Factor Promotes Leaf Senescence via ABA Biosynthesis, Plant Physiol., 2017, 174, 1747–1763 CrossRef CAS PubMed.
- R. Mega, A. Meguro-Maoka, A. Endo, E. Shimosaka, S. Murayama, E. Nambara, M. Seo, Y. Kanno, S. R. Abrams and Y. Sato, Sustained low abscisic acid levels increase seedling vigor under cold stress in rice (Oryza sativa L.), Sci. Rep., 2015, 5, 13 Search PubMed.
- H. Saika, M. Okamoto, K. Miyoshi, T. Kushiro, S. Shinoda, Y. Jikumaru, M. Fujimoto, T. Arikawa, H. Takahashi, M. Ando, S. Arimura, A. Miyao, H. Hirochika, Y. Kamiya, N. Tsutsumi, E. Nambara and M. Nakazono, Ethylene promotes submergence-induced expression of OsABA8ox1, a gene that encodes ABA 8′-hydroxylase in rice, Plant Cell Physiol., 2007, 48, 287–298 CrossRef CAS PubMed.
- L. F. Huang, K. H. Lin, S. L. He, J. L. Chen, J. Z. Jiang, B. H. Chen, Y. S. Hou, R. S. Chen, C. Y. Hong and S. L. Ho, Multiple Patterns of Regulation and Overexpression of a Ribonuclease-Like Pathogenesis-Related Protein Gene, OsPR10a, Conferring Disease Resistance in Rice and Arabidopsis, PLoS One, 2016, 11, e0156414 CrossRef PubMed.
Footnote |
† Electronic supplementary information (ESI) available. See DOI: 10.1039/c9en00828d |
|
This journal is © The Royal Society of Chemistry 2020 |